DOI:
10.1039/C8MT00223A
(Paper)
Metallomics, 2019,
11, 128-140
Characterization of the metal status of natively purified alpha-synuclein from human blood, brain tissue, or recombinant sources using size exclusion ICP-MS reveals no significant binding of Cu, Fe or Zn†
Received
1st August 2018
, Accepted 13th November 2018
First published on 13th November 2018
Abstract
Abnormal protein structure and function have been implicated as the toxic species in many diseases including neurodegenerative diseases, such as Parkinson's. One key pathological hallmark in Parkinson's disease is the formation of Lewy bodies, of which alpha-synuclein is the major component. These Lewy bodies are formed by the aggregation and oligomerization of alpha-synuclein. The oligomeric form of the protein is suspected to be the main contributor to the neurotoxicity seen in the disease. The formation of toxic oligomers has been shown to occur through reactions with lipids, dopamine, hydrogen peroxide as well as metals. The interplay between metals and alpha-synuclein has also been proposed to cause oxidative stress, which promotes the formation of protein aggregates. Most studies investigating the relationship of Cu, Fe and Zn with alpha-synuclein have relied on the use of recombinant protein and there is little evidence that the interaction between metals and alpha-synuclein are physiologically relevant. To address this gap in our knowledge we have characterized the metal content and metal binding capacity of alpha-synuclein purified from human erythrocytes and brain tissue. In addition, we examined the ability of dityrosine cross-linked alpha-synuclein oligomers to bind Cu, Fe and Zn. Using size exclusion chromatography-inductively coupled plasma-mass spectrometry we demonstrated that native human alpha-synuclein, recombinant familial mutants and oligomers do not bind to significant amounts of metal even when they are added to the protein in excess.
Significance to metallomics
We determined the amount of Cu, Fe, and Zn bound to the natively purified alpha-synuclein using size exclusion directly coupled to ICP-MS. There is extensive literature on metals binding to alpha-synuclein and the potential pathological role this may have in Parkinson's disease. However, there is a lack of evidence that the metal binding observed in vitro occurs in vivo. The delivery of metals in vivo is a tightly regulated process and metal interactions in vitro do not necessarily translate to the same metal being bound in a cellular context. Our work is highly significant because it demonstrates the power of using speciation ICP-MS techniques combined with modern quantitative mass spectrometry to determine the metal status and metal stoichiometry of a protein. Importantly, these techniques can be translated to other metalloproteins for direct in vivo characterization. Further, we show that in vitro binding does not necessarily translate metal binding in vivo.
|
Introduction
Alpha-synuclein is an abundant neurological protein with a number of proposed, yet unclear, physiological functions.1,2 Whilst its involvement in Parkinson's disease (PD) pathogenesis is the dominant subject with regard to alpha-synuclein pathology, it has also been shown to elicit effects in a range of other neurological conditions such as, Dementia with Lewy bodies (DLB)3 and multiple systems atrophy (MSA),4 all of which are now termed synucleinopathies.
Parkinson's disease is the second most common neurodegenerative disease following Alzheimer's disease. PD is a movement disorder where symptoms worsen with disease progression. The major symptoms of the disease are bradykinesia, stiffness, and tremor. The disease is characterized by the loss of dopamine neurons in the substantia nigra and the formation of Lewy bodies rich in alpha-synuclein. Parkinson's disease can either be sporadic with no known cause or hereditary. Some known heredity risk factors include mutations in genes such as SNCA, LRRK2, PARK16, PARK2 and DJ-1.5,6 The SNCA gene encodes for the protein alpha-synuclein which composes a major proportion of the Lewy bodies observed. Within the SNCA gene, familial point mutations include A53T,7 E46K,8 H50Q,9 G51D,10 A53E,11 and A30P.12 The inheritance of PD by these mutations can be recessive or dominant depending on the genetic mutation. Although alpha-synuclein is a highly abundant protein, gene triplication doubles the expression of alpha-synuclein which results in PD.13 The genetic evidence provides strong support for a causal role for alpha-synuclein in disease.
Alpha-synuclein is a soluble intrinsically disordered protein consisting of 140 amino acid residues. At an unknown point in the disease, and for reasons yet to be elucidated, alpha-synuclein begins to form self-associated oligomers that are neurotoxic in ex vivo models.14,15 One proposed mechanism of toxicity of alpha-synuclein oligomers is the disruption of membranes, which can lead to neuronal cell death.16 Oligomers of alpha-synuclein can be generated through a variety of mechanisms including reaction with dopamine,17 binding of lipids,18 iron,19 copper,20 and modification by oxidants including peroxynitrite21 and hydrogen peroxide.22,23 The binding of metals, such as Cu, has been suggested to be one cause of increased oxidative stress in PD.24 Additionally, Cu has been recognised to have an important role in the disease, this is potentially through the direct binding of Cu to alpha-synuclein.25,26
The interaction between alpha-synuclein and metal ions (such as Cu, Fe, and Zn) causes conformational changes resulting in abnormal folding of the protein.27 Experiments identifying protein–metal binding properties typically use non-native in vitro experimental procedures. While still providing important information regarding the chemical properties of alpha-synuclein, protein–metal interactions determined under simplified systems of the protein in the presence or absence of metal can misrepresent what occurs in the complex cellular environment of the neuron or red blood cell,28 two cell types with abundant expression of alpha-synuclein. With the above caveat in mind, in vitro and recombinant protein studies have identified specific metal binding sites in the alpha-synuclein sequence.25,26,29–34 Although these studies have investigated the binding of Cu and Fe to alpha-synuclein directly they have mostly focused on the monomeric form. However, the interaction of alpha-synuclein and Cu may not be limited to the monomeric state, the presence of Cu has been shown to induce the formation of alpha-synuclein oligomers20 and there is evidence that chelators can ameliorate the toxicity of hydrogen peroxide induced oligomers.23 This suggests a direct interaction between the oligomers and metal but there have been limited reports that have directly measured if oligomers of alpha-synuclein can directly bind to Cu or Fe.
The presence of metal binding domains identified in previous studies is only indicative of the potential for alpha-synuclein to bind metals under physiological conditions. Conditions within the cell microenvironments will have significant implications on the nature of metal–protein interactions observed.35 Of particular concern is metal misincorporation when metalloproteins are expressed and purified from recombinant sources. As an example the probing of the metalloproteome of Pyrococcus furiosus identified widespread metal misincorporation when the proteins were purified after being expressed in Escherichia coli.35 For instance, recombinant rubredoxin, an iron-based protein, will incorporate Zn instead of its native iron cofactor.36 Therefore, an accurate picture of physiological alpha-synuclein metal binding characteristics can only be confirmed from natively isolated protein where the specific chaperones, post-translation modifications, and microenvironments direct metal incorporation. For instance, in vivo alpha-synuclein is N-terminally acetylated37,38 which abolishes N-terminal metal binding.39 To determine the metal status of alpha-synuclein from a natural source we purified alpha-synuclein under native conditions from human red blood cells as well as brain tissue and directly measured the metals bound to the purified protein. We also mimicked the oxidative environment that occurs in PD and determined whether the covalently cross-linked oligomers, produced through oxidation,40,41 could directly bind Cu, Fe or Zn. Metal binding was directly measured using size exclusion chromatography-inductively coupled plasma-mass spectrometry (SEC-ICP-MS).
Materials and methods
Production of 15N labelled and unlabelled recombinant alpha-synuclein
Alpha-synuclein plasmid (pRSETB)17 was transformed in E. coli (BL21 (DE3)) cells were incubated overnight at 37 °C, on Luria broth agar (LB) plates with ampicillin for selection. The following day, one colony was picked and used to inoculated 2 mL of LB media, followed by incubation for 2 hours at 37 °C with shaking. After which, the cells were pelleted and transferred to 10 mL of growth media (50 mM KH2PO4, 50 mM Na2HPO4, 5 mM Na2SO4, 2.5 g L−1 NH4Cl, 2 mM MgSO4, 8 g L−1 glucose and 100 µg mL−1 ampicillin) and grown at 30 °C overnight, with shaking. The following day the cells were added to auto-inducing media specific for 15N protein expression42 at a 1
:
100 ratio (10 mL to 1 L) and then grown at 37 °C for 3 hours followed by overnight incubation at 20 °C. Cells were then were pelleted in preparation for purification as previously described,17 with the alteration of the size exclusion column being used in the final step to a Superdex75 10/300 GL size exclusion column (GE Lifesciences). The concentration of purified 15N labelled alpha-synuclein was determined by amino acid analysis (Australian Proteome Analysis Facility Ltd).
Buffer preparation
The preparation of phosphate buffers was done using a mixture of monobasic and dibasic phosphate salts estimated by the Henderson–Hasselbalch equation and the final pH was adjusted using 0.1 M trace element grade HCL or ammonium hydroxide (Sigma-Aldrich, 99.999% trace metals basis). The use of trace element grade HCL and ammonium hydroxide is critical to preventing contamination of the solutions with metals (e.g. Cu, Fe, Zn). Similarly, Tris buffer was made from 1.5 M stock solutions (Bio-Rad) as powdered stocks are typically contaminated with Cu and other trace elements. All solutions used were routinely monitored by ICP-MS for trace element contamination.
Sample preparation of erythrocytes or brain tissue
Frozen human brain frontal cortex tissue (50–100 g) was obtained from the Victorian Brain Bank Network. Experiments were approved by the University of Melbourne health sciences, human ethics committee (ID1136882). Human erythrocytes (100–300 mL), isolated as previously described,43 were lysed using 3× the volume of the packed red blood cells using lysis buffer (150 mM NH4Cl, 10 mM KHCO3, 10 µM EDTA pH 7.4, or without EDTA) with protease inhibitors (cOmplete™ EDTA free protease inhibitors cocktail tablets, Roche). Cells were rocked at 4 °C for 10 minutes prior to centrifugation at 14
000 × g for 10 minutes. Tissue free from neurological disease was homogenized in 4× the volume using 50 mM Tris, 150 mM NaCl pH 7.4 with protease inhibitors (cOmplete™ EDTA free, Roche) using a dounce homogenizer. The samples were then centrifuged at 16
000 × g for 30 minutes at 4 °C. The supernatant were used for the next step of the purification.
Ammonium sulfate precipitation
The supernatant from the human erythrocytes or brain tissue were brought up to 25% saturation (calculated from tables in Wingfield et al.44) ammonium sulfate by slowly adding solid powder to a stirred solution kept at 0 °C via an ice bath. After addition of the ammonium sulfate the sample was stirred for 1 hour prior to the pelleting at 20
000 × g for 20 min. The resulting supernatant was brought up to 50% ammonium sulfate as done previously. After centrifugation the pellet was then washed using 55% ammonium sulfate solution, this was performed by rocking the sample in the solution for 10 minutes and then centrifuging at 20
000 × g for 20 minutes. This process was repeated typically 3–5 times, until the red colour in the supernatant was absent.
Hydrophobic interaction chromatography (HIC)
The resulting pellets from the ammonium sulfate precipitation were then resuspended in 50 mM sodium phosphate, 1 M (NH4)2SO4 pH 7 by rocking the sample at 4 °C for 1 hour. Samples were then centrifuged, and the supernatant recovered for injection onto the column. The hydrophobic interaction column (HiTrap Phenyl HP 5 mL, GE) was equilibrated using buffer A (50 mM sodium phosphate, 1 M (NH4)2SO4 pH 7). Proteins were eluted with a linear gradient of 0–100% 50 mM sodium phosphate pH 7 over 15 column volumes with a flow rate of 1.5 mL min−1 and 1.5 mL fractions being collected across the entire run. The resulting fractions were analyzed SDS-PAGE and western blot analysis. Fractions were mixed with 4× loading dye (BioRad) and the samples were heated at 90 °C for 5 minutes. The samples were loaded on to a 26 well 4–12% Bis–Tris Criterion-XT precast gel (BioRad) and separated at 120 V for 1 hour. Following this, gels were either transferred to the PVDF membrane or stained for total protein with Oriole™ (BioRad) for 2 hours. Following transfer to PVDF membrane the blot was incubated overnight with anti-alpha-synuclein antibody [4D6] (ab1903, ABcam) at a 1
:
5000 dilution in 5% non-fat dry milk in TBS-T (Tris buffered saline-0.05% Tween 20). The membrane was then incubated with the secondary anti-mouse-HRP conjugate antibody (Dako), 1
:
5000 dilution, for 1 hour prior to three washes in TBS-T and development with ECL imaging reagents (Millipore). Fractions positive for alpha-synuclein were pooled together and buffer exchanged into anion exchange buffer A (20 mM Tris, 25 mM NaCl pH 8) and concentrated using 10k MWCO centricons (Millipore).
Anion exchange chromatography (AEX)
The anion exchange column (4.6 × 50 mm SAX guard column, Agilent) was equilibrated using buffer A (20 mM Tris, 25 mM NaCl pH 8). Proteins were eluted with a linear gradient of 0–50% buffer B (20 mM Tris, 1 M NaCl pH 8) over 15 column volumes at a flow rate of 0.5 mL min−1 with 0.5 mL fractions being collected across the entire run. Fractions were once again analyzed using western blots and SDS-PAGE. The positive fractions were then concentrated individually using 3k MWCO 0.5 mL centricons (Millipore) before size exclusion chromatography. The size exclusion column (Superdex 75 10/300, GE) was developed with 50 mM ammonium acetate pH 8.5. for 1.5 column volumes at a flow rate of 0.75 mL min−1 with 0.75 mL fractions collected. Purity was assessed using SDS-PAGE and protein identity was confirmed using mass spectrometry analysis on trypsin digested material.
Nondenaturing EDTA free purification of alpha-synuclein from erythrocytes
We purified alpha-synuclein according to Fauvet et al. in the absence of any EDTA.45 Briefly, red blood cells (RBC) were lysed, haemoglobin was depleted using nickel loaded HiTrap™ HP (GE Healthcare) and haemoglobin depleted material was fractionated by anion exchange chromatography, followed by hydrophobic interaction chromatography and lastly by size exclusion chromatography.
Addition of Cu to apo Cu, Zn superoxide dismutase 1 (SOD1)
ApoSOD (Bovine, Sigma) was generated using previously published protocols.46 ApoSOD1 (2.6 µM) was mixed with 2.6 µM Cu–glycine (1
:
1 mol
:
mol) or 266 µM Cu–Gly (10
:
1 mol
:
mol) and incubated for 30 minutes at 37 °C. The mixtures were analyzed by SEC-ICP-MS. The controls (holoSOD1, apoSOD1) were submitted to the same conditions (time and temperature). The Cu–glycine and Zn–glycine buffers were prepared by mixing Cu or Zn sulfate with a 4 molar excess of glycine and Fe sulfate was mixed with 4 molar excess of citrate buffer.
Addition of Cu to recombinant alpha-synuclein (WT, A30P, A53T) or alpha-synuclein from red blood cells or ribonuclease A
Alpha-synuclein (2.6 µM) was mixed with 5 µM Cu–glycine, riobonuclease a was mixed with 266 µM Cu–glycine (1
:
10 mol
:
mol) and each sample was incubated for 30 minutes at 37 °C. After incubation, metal to protein ratios were measured by SEC-ICP-MS.
SEC-ICP-MS analysis
Size exclusion (SEC)-inductively coupled plasma mass spectrometry (ICP-MS) was performed as previously described.47 Briefly, The Agilent Technologies 1200 HPLC system was directly connected to the Agilent Technologies 7700x ICP-MS, allowing the sample to be separated by size exclusion chromatography (Bio SEC-3 HPLC column, 4.6 × 300 mm, 3 µm particle size, 150 Å pore size, Agilent Technologies or a Superdex 200 column, 5 × 150 mm, GE Healthcare) and then flow into the ICP-MS where the metal ions present in the samples were detected. Samples were separated using 200 mM ammonium nitrate pH 8, containing 10 µg L−1 Ce and 10 µg L−1 Sb as internal standards. Absorbance was monitored at 280 nm (1200 series VWD, Agilent). ICP-MS parameters are listed in Table 1. The protein standards used were Bovine Cu/Zn superoxide dismutase (SOD, Sigma), ferritin (FTN), conalbumin and ribonuclease A (GE Healthcare). The SOD and ferritin standards were reconstituted in ammonium nitrate running buffer to contain 200 µg L−1 of Cu and Zn and 2000 µg L−1 of Fe as determined by bulk analysis of total metal by ICP-MS. Standard curves were generated from this sample by making injections were made of 1, 5, 10, 15 and 30 µL and the instrument response was converted to pg of each metal per second, as we have previously described.48 The SOD/FTN standards were used in the calibration of the ICP-MS for the metal Cu, Fe, and Zn and after linear regression was applied to the curves a conversion factor was determined for each of the metals so that the ICP-MS counts per second (CPS) can be converted into picograms per second. All analysis was performed using Graphpad Prism 6 or 7.
Table 1 Agilent 7700x ICP-MS
Parameters |
RF power |
1550 W |
Sample depth |
8.0 mm |
Carrier gas |
0.55 L min−1 |
Makeup gas |
0.50 L min−1 |
Spray chamber temperature |
2 °C |
Extracts 1, 2 |
5.0, −145 V |
Omega bias, lens |
−50, 9.0 V |
Deflect, plate bias |
1, −70 V |
Cell entrance, exit |
−40, −70 V |
Octopole bias, RF |
−18, 170 V |
Collision gas |
He, 4.0 mL min−1 |
Generation of alpha-synuclein oligomers
Protein concentrations were determined using UV spectroscopy (for hemoglobin a wavelength of 405 nm and an extinction coefficient of 178
000 M−1 cm−1 and for recombinant alpha-synuclein a wavelength of 280 nm and an extinction coefficient of 5120 M−1 cm−1). Haemoglobin (5 µM) was incubated with hydrogen peroxide (100 µM, H2O2) for 5 minutes. Formation of oligomers was induced by addition of recombinant alpha-synuclein to the hydrogen peroxide/hemoglobin mixture to a final concentration of 20 µM followed by a further addition of hydrogen peroxide to a final concentration of 20 µM. Phosphate buffered saline (1× PBS, 137 mM NaCl, 2.7 KCl, 10 mM Na2KHPO4, 1.8 mM KH2PO4) was added to reach the desired reaction volume of 150 µL. After 20 minutes of incubation, copper sulfate was added to the reaction mixture at a 1
:
1 molar ratio to alpha-synuclein. Since alpha-synuclein and hemoglobin are similar in molecular weight they required separation before analysis of metal content by SEC-ICP-MS. To remove hemoglobin from alpha-synuclein the sample was fractionated using strong anion exchange chromatography (BIOSAX 4.6 × 50 mm, 5 µm, Agilent Technologies). The sample was loaded in 20 mM Tris pH 8 and separated over a 20 column volume gradient from 0–35% buffer B (20 mM Tris, 1 M NaCl pH 8) at a flow rate of 1 mL min−1. Fractions (850 µL) were collected into a 1 mL 96 deep well plate (P-DW-11-C, Axygen) across the gradient. Separation of the proteins and oligomer location was confirmed by western blot of the collected fractions. For western blot analysis the fractions were diluted 1 in 10, and 5 µL of 4× SDS loading dye (BioRad) was added before being heated at 70 °C for 10 minutes. 25 µL of each sample was loaded onto a 26 well 4–20% Criterion TGX precast gel. Protein separation occurred at 180 V for 30 minutes. The fractions that contained monomeric and oligomeric alpha-synuclein were analyzed for metal binding using size exclusion chromatography-inductively coupled plasma-mass spectrometry (SEC-ICP-MS).
Concentration of alpha-synuclein by LC-MS/MS
The amount of alpha-synuclein in each of the anion exchange fractions was determined using multiple reaction monitoring (MRM) liquid chromatography mass spectrometry assay (LC-MS). This was performed using an Agilent 6495 triple quadrupole LC/MS/MS equipped with an Infinity series II UHPLC (Agilent). Anion exchange fractions (15 µL) were spiked with 15N labeled alpha-synuclein to a final concentration of 20 fmol µL−1. To this reaction, 0.2 µg of trypsin (Sigma, was added and the samples were digested at 50 °C for 2 hours). Following digestion, the samples were acidified with 5 µL of 10% formic acid. 1 µL of the digested material was loaded onto an AdvanceBio peptide map column (2.1 × 150 mm, 2.7 µm, Agilent technologies) maintained at 55 °C. The peptides were loaded onto the column at a flow rate of 0.4 mL min−1 in 97% buffer A, 0.1% formic acid in water: 3% buffer B, 0.1% formic acid in acetonitrile (ACN). The column eluent was diverted to waste for the first 5 minutes to ensure any excess salt in the sample did not flow into the mass spectrometer. The column eluent was then allowed to flow onto the mass spectrometer and the peptides in the sample were eluted using a gradient of increasing amounts of buffer B (time (min): % B), 0
:
3, 5
:
3, 6.5
:
10, 11.5
:
25, 12.5
:
81, 13.5
:
81, 13.6
:
3, stop time 14 minutes with 3 minute post-run re-equilibration.
Optimized conditions for the detection of alpha-synuclein peptides were determined using the Agilent optimizer software in combination with Skyline (version 3.5.0.9319) with Agilent automation app (version B.07.01, build 7.1.7112.0). This process determines retention time windows and optimized collision energies and transitions for detection of peptides (Table S1, ESI†). The amount of alpha-synuclein in each of the column fractions was determined by determining the ratio of light to heavy alpha-synuclein.
In-gel digest and protein identification
Oriole stained protein bands of interest were excises and minced using a scalpel. The gel pieces were reduced with dithiothreitol (DTT), alkylated iodoacetamide and trypsin digested using previously published protocols49 with the following modification of ammonium bicarbonate being replaced with triethylammonium bicarbonate (Sigma-Aldrich). Nano liquid chromatography (Agilent Chip-cube) MS/MS was conducted on a quadrupole time of flight mass spectrometer (Agilent 6550QTOF) using a top-10 data dependent acquisition. Peptide searches were done with Mascot search engine50 with the following parameters; peptide tolerance 15 ppm and MS/MS tolerance 0.05 Da, database SwissProt, taxonomy homo sapiens, fixed modification carbamidomethyl cysteine, variable modification acetylation, methionine oxidation and pyroglutamate formation.
Results
Alpha-synuclein purified from erythrocytes
Alpha-synuclein was successfully purified from human erythrocytes based on the previously described protocols,45,51 (Fig. 1A), with sufficient purity (>70%) required for characterization. The purification results were confirmed by SDS-PAGE (Fig. 1B) followed by mass spectrometry identification, the contaminating material were identified as heat shock protein 70 (P11142) and unidentified small peptide fragments (Table 2). Following purification on the superdex75 size exclusion column the alpha-synuclein positive fractions were subjected to metalloproteins analysis via size exclusion chromatography coupled to an ICP-MS (SEC-ICP-MS). SEC-ICP-MS was used to determine if there were metalloproteins present in the final purified material. The results of this experiment showed that natively purified, erythrocyte, alpha-synuclein did not bind to significant amounts of Fe, Cu or Zn (Fig. 2A) nor did we see any binding of Co, Mn or Ni (data not shown). Similar results were found for material purified without 10 µM EDTA in the lysis buffer or material purified using the Fauvet et al. method45 for the isolation of native alpha-synuclein (Fig. 2H). Recombinant studies of metals binding to alpha-synuclein require the addition of metal. We replicated the addition of Cu, Fe or Zn based on published protocols29,32,52 to the purified native protein at a ratio of 1
:
1.2 (protein
:
metal) followed by SEC-ICP-MS analysis. The results showed no significant binding of metal to alpha-synuclein (Fig. 2B). Overall, these results indicate that alpha-synuclein, natively purified from erythrocytes by these three different protocols, was not a metalloprotein. We also observed a significant shift in the retention time of alpha-synuclein purified from red blood cells (Fig. 2A and B) the elution time shifted from the 370 s, observed for recombinant, brain and other preparations of RBC purified alpha-synuclein, to 420 s indicative of a smaller hydrodynamic radius and thus lower molecular mass (ca. 15–20 kDa). To determine if this corresponded to alpha-synuclein we collected fractions (40 µL each) across the entire chromatographic run and subjected them to trypsin digestion and quantitative MS/MS using 15N-labelled alpha-synuclein. This indicated alpha-synuclein eluted at 420 s (data not shown). We attribute the variation in elution to the specific donor that was used. Individual variability in the amount of alpha-helical content of alpha-synuclein has been reported previously53 and may explain this observation. Despite this difference in apparent molecular mass there was no association of Cu, Zn or Fe (Fig. 2A).
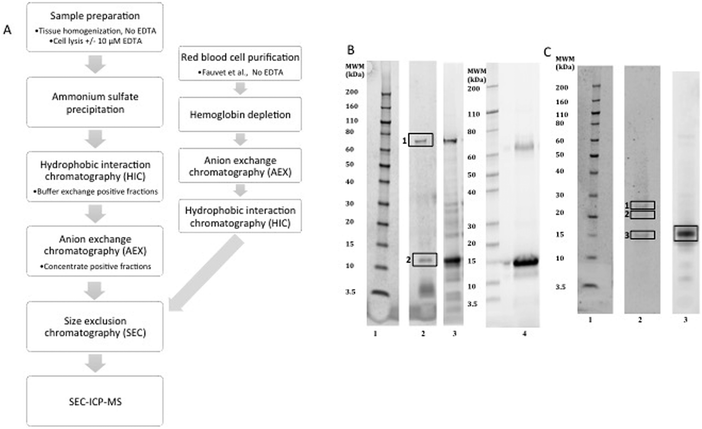 |
| Fig. 1 Purification of alpha-synuclein. (A) Outline of the purification steps used to purify alpha-synuclein from human erythrocytes or brain tissue. (B) Alpha-synuclein purified from human erythrocytes analysed for purity by SDS-PAGE gel stained with Oriole (BioRAD) a general protein stain. (C) Purified alpha-synuclein from human brain tissue. Lane 1 molecular weight marker and lane 2 purified alpha-synuclein from red blood cells or brain with 10 µM EDTA in the lysis buffer or without EDTA Lane 3. Material purified from red blood cells using the Fauvet et al. method, lane 4 (B). The boxes indicate the bands excised for mass spectrometry identification listed in Table 2 for the red blood cell material (B) and Table 3 for the brain derived material (C). | |
Table 2 Mass spectrometry results for the SEC-purified blood alpha-synuclein digested with trypsin, as indicated in Fig. 1A
Band |
Protein ID |
MASCOT score |
MW (Da) |
Peptides |
%Protein sequence coverage |
1 |
Heat shock cognate 70 (P11142) |
809 |
71 082 |
16 |
23 |
2 |
Alpha-synuclein (P37840) |
1965 |
14 451 |
38 |
59 |
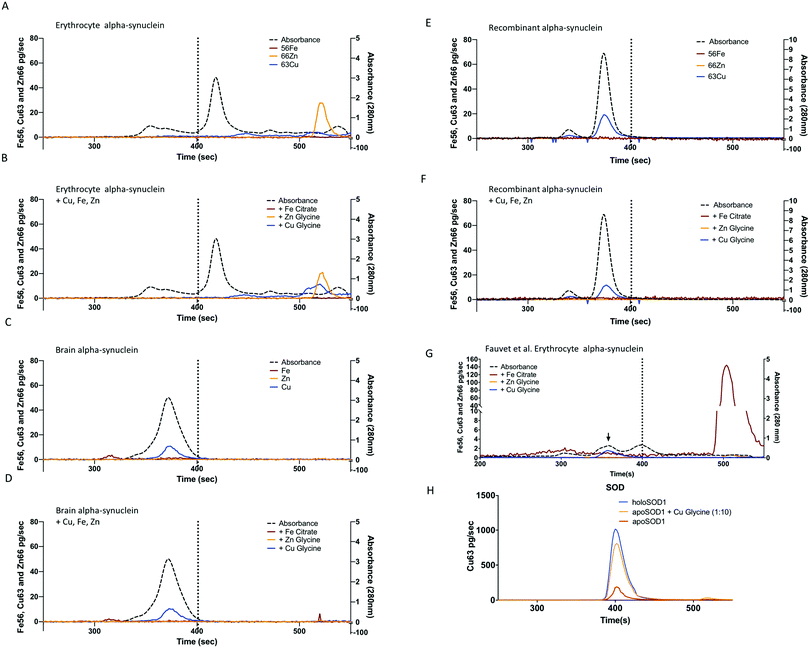 |
| Fig. 2 Metalloproteomic analysis of alpha-synuclein. (A) The average (n = 3) SEC-ICP-MS metal traces for natively purified alpha-synuclein from erythrocytes and (B) the same material after incubation with Cu glycine (blue line), Zn glycine (orange) or Fe citrate (red) added (1.2 : 1 metal : protein ratio). Panels A–F conducted with Agilent BioSEC 4.6 × 300 mm column with the vertical dashed line indicated the elution of the 32 kDa SOD standard. (C) SEC-ICP-MS metal chromatograms for natively purified alpha-synuclein from brain and (D) the same material with metals added before analysis. (E) Analysis of recombinant alpha-synuclein or (F) recombinant protein after the addition of Cu, Fe and Zn. (G) Analysis of natively purified alpha-synuclein from red bloods using the Fauvet et al. method (blue trace, material from Fig. 1B lane 4) with a 1 : 1 molar ratio addition of Cu–glycine, Zn glycine or Fe citrate. (H) Apo-SOD (2.6 µM, 0.22 mol Cu : protein, red trace) at the same concentration as the alpha-synuclein used in panels A–G, after incubated with 1 : 1 ratio of Cu : glycine (orange trace) and in the holo form (blue trace). | |
Alpha-synuclein purification from human brain
The purification protocol used for the red blood cell isolation was repeated two times using human cortical brain tissue (Fig. 1) in the presence or absence of 10 µM EDTA and yielded a purity of >60% (Fig. 1C) with the only detectable contaminating proteins being hemoglobin and β-synuclein (Table 3). SEC-ICP-MS analysis on the purified brain material demonstrated a lack of any metals (Cu, Zn, or Fe; Fig. 2 or Mn, Co and Ni Data not shown) bound to alpha-synuclein. For both Fe and Zn, the metal to protein ratio was below our detection limits of 0.001 ratio (Zn) and 0.002 (Fe) moles of metal to a mole of alpha-synuclein. For Cu, the metal to protein ratio was 0.0033 ± 0.0001 (average ± STDEV, n = 3) as isolated with EDTA in lysis buffer only, and 0.0034 ± 0.0001 moles Cu
:
protein without EDTA in lysis buffer and 0.0011 ± 0.0002 as isolated with the Fauvet et al. method (Fig. 1B). These values of Cu
:
protein were above the detection limit of 0.001 but not above the limit of quantification (0.010) Cu
:
moles of a-syn. This is far below the expected 1
:
1 ratio predicted from studies on recombinant alpha-synuclein. For reference, if alpha-synuclein had metals bound at a 1
:
1 ratio the expected response for Cu would have had a peak height of ca. 2000 pg s−1. The purification process involves several chromatographic steps that could have potentially stripped the metal away from alpha-synuclein, therefore, we added Cu, Fe or Zn to the purified brain alpha-synuclein and repeated the metalloproteomic analysis, however, similar to the red blood cell material, there was no significant metal binding (Fig. 2D). As a positive control to test that the SEC-ICP-MS system we used could detect Cu binding proteins under the conditions employed we used apo-superoxide dismutase (Bos Taurus, 0.23 ± 0.03 Cu
:
SOD, mean ± SD) and mixed it with copper–glycine under identical conditions used for alpha-synuclein and used SEC-ICP-MS to determine binding of Cu to the protein (Fig. 2H). This resulted in 0.98 ± 0.11 moles of Cu per mole of SOD, consistent with the expected 1
:
1 ratio known for SOD, and measured when purified from natural sources.54 We hypothesized that the weak association of Cu with alpha-synuclein was not due to a specific metal binding site but due to an electrostatic interaction that can occur with proteins in general terms. To test this we exposed ribonuclease A, a protein with no know metal association, to Cu under the same conditions used for SOD and alpha-synuclein. Analysis via SEC-ICP-MS demonstrated a similar level of Cu associated with ribonuclease A as we saw for alpha-synuclein (0.0035 ± 0.0009, mean ± SD) when Cu was added at a 1
:
1 molar ratio, with no significant increase in Cu observed (0.0042 ± 0.0007, p = 0.5, 2-tailed, paired t-test). When the molar ratio of Cu
:
protein in the mixture was increased to 10
:
1 (266 µM Cu) there was a significant increase in the amount of Cu associated with ribonuclease A (0.00561 ± 0.0007, p = 0.008) compare to the protein alone, but still far below what would be expected for a bona fide Cu binding protein.
Table 3 Mass spectrometry results for the SEC-purified brain alpha-synuclein digested with trypsin, as indicated in Fig. 1C
Band |
Protein ID |
MASCOT score |
MW (Da) |
Peptides |
%Protein sequence coverage |
1 |
Alpha-synuclein |
329 |
14 451 |
11 |
54 |
Beta-synuclein |
227 |
14 279 |
7 |
46 |
2 |
Haemoglobin subunit beta |
217 |
16 102 |
6 |
32 |
3 |
Alpha-synuclein |
606 |
14 451 |
23 |
53 |
Beta-synuclein |
450 |
14 279 |
22 |
34 |
Alpha-synuclein |
646 |
|
24 |
59 |
Beta-synuclein |
512 |
|
22 |
34 |
Recombinant alpha-synuclein
We conducted SEC-ICP-MS with recombinant alpha-synuclein purified from E. coli using conditions that replicate previous reports that produced metal bound recombinant alpha-synuclein. Consistent with our observations with the erythrocyte and brain material, the recombinant protein did not bind metals (Fig. 2E). However, similar to the brain alpha-synuclein, there was a sub-stoichiometric association of Cu with the protein ((0.027 ± 0.003) metal
:
protein, Fig. 2F). We also investigated if the pathological mutants induced a binding site for copper. We recombinantly expressed the three familial mutants A30P, E46K, A53T55 and determined if copper bound to these proteins under the same conditions as described above. The measured molar ratio of Cu to alpha-synuclein for mutants was similar to the wild type recombinant protein with no significant association of Cu. The molar ratios of Cu
:
protein were as follows; A30P with copper added (0.0034 ± 0.002 moles Cu
:
protein), A53T with copper (0.0041 ± 0.0002), and E46K with copper (0.003 ± 0.0001).
Generation of alpha-synuclein oligomers
Hemoglobin (Hb) is an abundant protein partner to alpha-synuclein in red blood cells and in the presence of excess hydrogen peroxide can cause degradation of Hb and release of free Fe that can participate in Fenton chemistry.56,57 Hb–Fe(IV) oxo species were generated in the presence of hydrogen peroxide, these species were then used to generate alpha-synuclein oligomers.
We confirmed that this reaction mixture produced alpha-synuclein oligomers by western blot (Fig. 3B) and mass spectrometry.40 The creation of oligomers via this mechanism would potentially mimic the excess Fe situation in the disease affected regions of the brain in PD and may contribute to the oligomerisation that occurs in vivo.
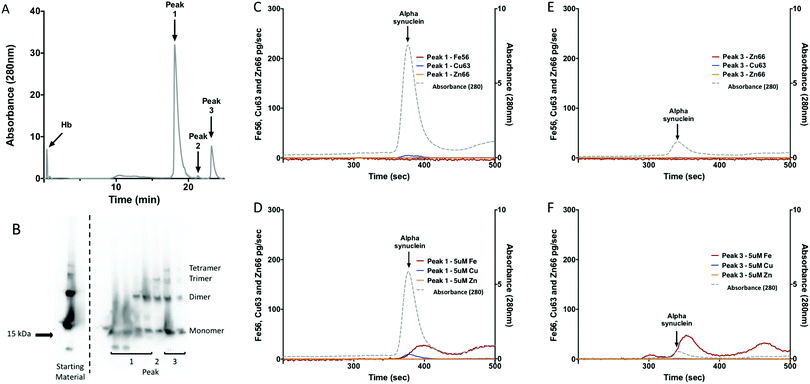 |
| Fig. 3 Metalloproteomic analysis of alpha-synuclein oligomers. (A) UVA280 chromatograph of the anion exchange separation of hemoglobin (Hb) and recombinant alpha-synuclein reaction mixture. The elution of Hb and the oligomeric peaks of recombinant alpha-synuclein are indicated by arrows. (B) Alpha-synuclein western blot demonstrates the formation of recombinant alpha-synuclein oligomers. (C) SEC-ICP-MS analysis of the metal content of the monomeric recombinant alpha-synuclein, (D) after the addition of 5 µM Cu, Fe and Zn, (e) analysis of the oligomeric containing fraction, (F) same as (E) but after the addition of 5 µM Cu, Fe and Zn. Grey dotted line indicated the protein elution by absorbance at 280 nm. The maroon, blue and orange lines indicated the SEC-ICP-MS data for Fe56, Cu63, and Zn66 in pg s−1 respectively. | |
Metal binding capacity of isolated alpha-synuclein oligomers
Because Hb and alpha-synuclein have similar elution times from SEC we needed to further isolate the protein mixture to avoid metal associated with Hb being interpreted as metal associated with alpha-synuclein. To do this we used strong anion exchange (BIOSAX) chromatography at pH 8, under these conditions Hb does not significantly interact with the BIOSAX column and elutes in the flow through (Fig. 3A). Anion exchange chromatography resulted in three main alpha-synuclein peaks (Fig. 3A). The first species to elute from the anion exchange represents monomeric alpha-synuclein, while peak 3 represents an oligomeric form of the protein. The metal binding ability of alpha-synuclein oligomers and monomers were assessed using SEC-ICP-MS (Fig. 3C–F). Without the addition of extra exogenous metals after the separation neither the monomeric or oligomeric form of alpha-synuclein had detectable levels of Cu, Fe or Zn bound (Fig. 3C and E). Addition of Cu, Fe and Zn to the samples, resulted in an interaction of monomeric of alpha-synuclein with Cu and Fe but not Zn (Fig. 3E and F). An interaction with Fe was observed for the oligomer (Fig. 3F). The interaction with Fe was accompanied by a shift in the retention time of alpha-synuclein consistent with a change in the molecular shape of the protein.
To determine the molar ratio of Cu to alpha-synuclein we determined the concentration of alpha-synuclein by QQQ MS using 15N labeled recombinant alpha-synuclein as an internal standard. The quantitation of Cu from the SEC-ICP-MS combined with the protein concentration allowed for the molar ratio of Cu
:
alpha-synuclein to be determined. The molar ratio of the monomer of alpha-synuclein was 0.003 ± 0.0003 moles of Cu
:
alpha-synuclein (0.3% Cu; Table 4). The molar ratio for oligomer of alpha-synuclein was 0.02 ± 0.002 moles of Cu
:
alpha-synuclein (2% Cu; Table 4). The ratio of Fe, in the shifted peak (Fig. 3F), was similar to that seen with Cu (Table 4). Although the Cu peak appears to be significant when this is compared to the amount of alpha-synuclein that was injected it is less than 1% of what would be expected for a 1
:
1 molar ratio of Cu
:
alpha-synuclein.
Table 4 Molar ratio of metal and recombinant alpha-synuclein monomers and oligomers (Fig. 3D and F)
|
Molar ratio Cu : protein |
Molar ratio Fe : protein |
Molar ratio Zn : protein |
Monomeric alpha-synuclein |
0.003 |
0.00006 |
Below detection |
Oligomeric alpha-synuclein |
0.02 |
0.002 |
Below detection |
Discussion
Previous studies have shown that alpha-synuclein binds metals and this can even produce an enzymatic activity,33 however, these results relied on recombinant protein. If alpha-synuclein binds to metals in vivo then it should be possible to isolate the metal-bound form of the protein, unless the interaction were too weak to survive purification. Many of the previous purifications for alpha-synuclein from human tissue used heat denaturation steps that take advantage of the heat stability of alpha-synuclein58 The use of heat often in combination with metal chelators (EDTA) would most likely strip any metal associated with alpha-synuclein. Hence the purification steps used in these experiments endeavoured to keep the protein in its native state and avoid excessive chelators.
Alpha-synuclein purified from human erythrocytes appeared to only contain one other major contaminating protein identified as HSP70 (P11142) and small peptide like fragments (Fig. 1). This result is not entirely unexpected, as it has been reported that alpha-synuclein is able to interact with this HSP70 and it has been previously reported as a contaminant.59–62 The protein–protein interaction may be the reason why it is still present in the sample at the very end of the purification protocol. Alternatively, due to its high abundance in the red blood cells it may be due to incomplete purification.
The alpha-synuclein from human brain contained two contaminating proteins (Fig. 1). These were determined to be Hb and β-synuclein. The Hb is likely caused by carry over from the initial steps in the purification. Beta-synuclein is known to be located within the CNS, and has been shown to co-localise with alpha-synuclein in the mouse brain.63 Previous purifications of alpha-synuclein have also reported the co-purification of beta-synuclein.53
Size exclusion-inductively coupled mass spectrometry (SEC-ICP-MS) analysis of purified alpha-synuclein demonstrated that alpha-synuclein purified from brain, or erythrocytes did not bind metals at the 1
:
1 ratio that was expected. The initial purification involved lysis of the erythrocytes and brain with 10 µM EDTA, an amount well below the level used in protease inhibitor cocktails (1 mM) and consistent with the concentrations used in the purification of other metalloenzymes (SOD, xanthine oxidase, carbonic anhydrase).54,64,65 However, due to the possibility that it was chelating metals from alpha-synuclein we repeated the purification without EDTA and with a separate protocol that does not involve EDTA (Fig. 1B and C). Despite these attempts to preserve any metal associated with alpha-synuclein in RBC or brain, the SEC-ICP-MS analysis demonstrated there was no significant association of Cu, Fe, Zn, Mn, Ni or Co (Fig. 2). Further, addition of Cu, Fe and Zn using previously reported conditions29,32,52 did not alter the metal status of alpha-synuclein. In vivo N-terminal acetylation and N-terminal truncation of alpha-synuclein are well documented37,38 and would prevent the binding of Cu, Fe or Zn to the N-terminal binding sites previously described.39,66 The material purified from brain and erythrocytes demonstrated N-terminal acetylation consistent with previous reports.37,38 Based on this the lack of metal bound to the protein is not entirely unexpected, especially if the key residues are missing and/or acetylation is present. However, we also failed to demonstrate binding of Cu, Fe or Zn to the recombinant alpha-synuclein which does not have the N-terminal acetylation. In a similar observation to the natively sourced alpha-synuclein the recombinant material also had a small, <5%, binding of Cu and no interaction with Fe or Zn. The oxidation state of Cu is an important determinate of binding67,68 and previous reports have indicated that alpha-synuclein also binds Cu(I).52 We did not investigate the binding of Cu(I) in this study but future work will explore this possibility. Another reason we did not observe any metal binding could be that the SEC-ICP-MS system, and the conditions used, were not able to preserve metal protein interaction. As a positive control, we exposed apoSOD to copper under the same conditions used for alpha-synuclein and demonstrated that we could restore the metal (Fig. 2H). Further, we suspect that the low percentage of Cu associated with alpha-synuclein was due to electrostatic interactions (e.g. the binding of sodium cations). We tested this by exposing ribonuclease A, a protein without any known metal binding affinity,69 to 1 and 10 molar excess of Cu, 26 µM and 266 µM Cu, respectively. In both conditions there was a significant association of Cu with the protein, but less than 5% molar ratio, consistent with a weak columbic interaction that has been previously described for NaCl and ribonuclease A.70 This agreement in the magnitude of Cu binding between alpha-synuclein and ribonuclease A is consistent with Cu binding to alpha-synuclein being driven by ionic interactions and is not of physiological significance. Our results are consistent with previous Cu binding reports which have often used 5 to 10 fold molar excess, and concentrations of Cu >50 µM, due to the nature of the techniques used (e.g. NMR, EPR, CD). For example an NMR study demonstrated the binding of Cu(I) to alpha-synuclein at equal molar ratio with a 300 µM protein concentration gave apparent affinities greater than 20 µM consistent with other reports in the high µM range. In a report using isothermal titration calorimetry (ITC) they describe sub nM affinities of alpha-synuclein for Cu(II) but again all experiments were conducted at 100 µM protein concentration and titration of Cu 400 µM.71 These levels of Cu are far above those achievable in vivo and are not likely to be different from affinities of general soluble globular proteins. However, negative controls performed under similar conditions with proteins that don’t have a clear high affinity (e.g. femtomolar) copper-binding site are not available. The biophysical evidence describes two copper binding sties for alpha-synuclein – one at the N-terminus in the nM range34,71,72 and another in the C-terminus between residues 119–136 which have been shown to bind Cu, Mn and Co but at µM affinities.32,73 Overall, neither of these binding affinities are sufficient, or comparable to known Cu biding proteins that have fM affinities.74,75 Further, the intracellular levels of Cu are tightly controlled and it is highly unlikely that concentrations would reach the nM range. Moreover, the nM site is abolished by N-terminal acetylation and truncation,37,38 thus it is a moot point to consider it in vivo. This only leaves the µM affinity-binding sites, that we have shown through a comparison with ribonuclease A, is likely to be a weak ionic interaction not specific for alpha-synuclein but common for many proteins. Further, the affinity and concentration of intracellular glutathione limits intracellular Cu to sub fM concentrations76,77 thus precluding alpha-synuclein from binding Cu. Traditional copper binding proteins have fM affinities74,75 that are 1
000
000 or more times greater than the nM affinities estimated for alpha-synuclein.
Oligomers of alpha-synuclein are neurotoxic and Cu has been shown to induce oligomerization20 and oxidation78 of alpha-synuclein. Yet it is not clear if oligomers bind transition metals. We used Hb and hydrogen peroxide to generate oligomers of alpha-synuclein, which we have shown are dityrosine cross-linked.40 The shift in elution time of alpha-synuclein oligomers that we observed was consistent with the ability of Fe to accelerate the rate of fibril formation19 and with the know differences in the class of fibrils generated by Cu compared to Fe.79 Oligomers generated in the presence of hydrogen peroxide and Hb were subjected to SEC-ICP-MS analysis to determine if any significant binding to Cu, Fe, or Zn occurs. The results indicate that although we could see some binding of Cu to alpha-synuclein (0.02 moles Cu
:
protein), it is not a significant amount. Indicating that although Cu can help induce the formation of alpha-synuclein oligomers the resulting oligomers do not bind significant amounts of Cu. There was an interaction with Fe observed but at a sub-stoichiometric amount (0.2%, Table 4). This suggests that the mechanism of Cu or Fe in driving the formation of oligomers and higher order aggregates may be through the induction of a seed which serves to accelerate fibril and oligomerisation80 or even by promoting oxidation of the protein without any significant direct interaction necessary. The interaction of the oligomers with Fe is consistent with reports of chelators preventing the oxidative stress induced by alpha-synuclein oligomers in cell culture models23 indicating that the sub stoichiometric binding of Fe may indeed have a pathogenic role and could represent a drugable target.
These results have important implications for understanding the role of Cu, Fe, and alpha-synuclein in PD and the potential of oligomers to have ferrireductase activity.33 As well as our understanding of how oligomers of alpha-synuclein bind Cu and Fe which are often depicted as a 1
:
1 ratio.81 One caveat is that the delivery of Cu & Fe is a highly regulated process in a cell thus there could be chaperones or other factors in vivo including the association with membranes that could impact the loading of Cu and Fe to alpha-synuclein. Further validation of alpha-synuclein oligomers isolated from in vivo sources or in the presence of membranes are required to validate our results. However, this work leaves the possibility open that Cu and Fe may serve to induce a small percentage <1% of the alpha-synuclein molecules to nuclei that can serve to generate oligomers and fibrils. The difference in how Fe and Cu interact with alpha-synuclein to generate unique nuclei may contribute to the different morphologies observed with these two metals.80
Conclusion
Our results demonstrate that alpha-synuclein purified from human brain or red blood cells was not a classic metalloprotein with 1
:
1 binding ratio as often described. Further, recombinant alpha-synuclein and pathological mutants (A30P, E46K and A53T) failed to demonstrate an interaction with Cu, Fe or Zn. In a similar fashion oligomers of alpha-synuclein generated in the presence of Hb and hydrogen peroxide were able to bind small amounts of Cu and Fe, with molar ratios of 0.02 and 0.002 respectively but in a manner consistent with a weak ionic interaction and of no physiological consequence. Thus, we conclude that oligomers of alpha-synuclein do not bind Cu, Fe or Zn in a classic 1
:
1 ratio as may be expected and that natively purified alpha-synuclein is not a metalloprotein in vivo. Overall, the substoichiometric interactions we observed are not of sufficient affinity to be of any physiological significance, and when combined with the known fM intracellular Cu concentrations it explains why alpha-synuclein isolated from red blood cells or brain fails to have metal associated with it. One caveat of our study is we used non-pathogenic tissues, thus it is possible that under pathological conditions modifications of alpha-synuclein may induce metal binding via post-translational modification (e.g. phosphorylation) or changes in binding substrates (e.g. lipids, protein binding partners) that result in altered cellular compartmentalization or structural rearrangements. Another important limitation to our study is the purification was done under aerobic conditions that would not preserve a volatile Cu(I) bound to alpha-synuclein future studies will explore this possibility.
Conflicts of interest
Blaine R. Roberts receives research support from Agilent Technologies.
Acknowledgements
We acknowledge funding from the Victorian Government's Operational Infrastructure Support Program. BR is a National Health and Medical Research Council Dementia leadership fellow (Grant ID APP1138673), and partial support from the Cooperative Research Centre for Mental Health (Grant ID: 20100104) and receives research and financial support from Agilent technologies.
References
- M. Goedert, Alpha-synuclein and neurodegenerative diseases, Nat. Rev. Neurosci., 2001, 2(7), 492–501 CrossRef CAS
.
- T. F. Outeiro and S. Lindquist, Yeast cells provide insight into alpha-synuclein biology and pathobiology, Science, 2003, 302(5651), 1772–1775 CrossRef CAS
.
- M. G. Spillantini, M. L. Schmidt, V. M.-Y. Lee, J. Q. Trojanowski, R. Jakes and M. Goedert, α-Synuclein in Lewy bodies, Nature, 1997, 388(6645), 839–840 CrossRef CAS
.
- P. H. Tu, J. E. Galvin, M. Baba, B. Giasson, T. Tomita, S. Leight, S. Nakajo, T. Iwatsubo, J. Q. Trojanowski and V. M. Lee, Glial cytoplasmic inclusions in white matter oligodendrocytes of multiple system atrophy brains contain insoluble alpha-synuclein, Ann. Neurol., 1998, 44(3), 415–422 CrossRef CAS
.
- W. Satake, Y. Nakabayashi, I. Mizuta, Y. Hirota, C. Ito, M. Kubo, T. Kawaguchi, T. Tsunoda, M. Watanabe, A. Takeda, H. Tomiyama, K. Nakashima, K. Hasegawa, F. Obata, T. Yoshikawa, H. Kawakami, S. Sakoda, M. Yamamoto, N. Hattori, M. Murata, Y. Nakamura and T. Toda, Genome-wide association study identifies common variants at four loci as genetic risk factors for Parkinson's disease, Nat. Genet., 2009, 41(12), 1303–1307 CrossRef CAS PubMed
.
- J. Simon-Sanchez, C. Schulte, J. M. Bras, M. Sharma, J. R. Gibbs, D. Berg, C. Paisan-Ruiz, P. Lichtner, S. W. Scholz, D. G. Hernandez, R. Kruger, M. Federoff, C. Klein, A. Goate, J. Perlmutter, M. Bonin, M. A. Nalls, T. Illig, C. Gieger, H. Houlden, M. Steffens, M. S. Okun, B. A. Racette, M. R. Cookson, K. D. Foote, H. H. Fernandez, B. J. Traynor, S. Schreiber, S. Arepalli, R. Zonozi, K. Gwinn, M. van der Brug, G. Lopez, S. J. Chanock, A. Schatzkin, Y. Park, A. Hollenbeck, J. Gao, X. Huang, N. W. Wood, D. Lorenz, G. Deuschl, H. Chen, O. Riess, J. A. Hardy, A. B. Singleton and T. Gasser, Genome-wide association study reveals genetic risk underlying Parkinson's disease, Nat. Genet., 2009, 41(12), 1308–1312 CrossRef CAS PubMed
.
- M. H. Polymeropoulos, C. Lavedan, E. Leroy, S. E. Ide, A. Dehejia, A. Dutra, B. Pike, H. Root, J. Rubenstein, R. Boyer, E. S. Stenroos, S. Chandrasekharappa, A. Athanassiadou, T. Papapetropoulos, W. G. Johnson, A. M. Lazzarini, R. C. Duvoisin, G. Di Iorio, L. I. Golbe and R. L. Nussbaum, Mutation in the α-Synuclein Gene Identified in Families with Parkinson's Disease, Science, 1997, 276(5321), 2045–2047 CrossRef CAS
.
- J. J. Zarranz, J. Alegre, J. C. Gómez-Esteban, E. Lezcano, R. Ros, I. Ampuero, L. Vidal, J. Hoenicka, O. Rodriguez, B. Atarés, V. Llorens, E. G. Tortosa, T. del Ser, D. G. Muñoz and J. G. de Yebenes, The new mutation, E46K, of α-synuclein causes Parkinson and Lewy body dementia, Ann. Neurol., 2004, 55(2), 164–173 CrossRef CAS
.
- C. Proukakis, C. G. Dudzik, T. Brier, D. S. MacKay, J. M. Cooper, G. L. Millhauser, H. Houlden and A. H. Schapira, A novel α-synuclein missense mutation in Parkinson disease, Neurology, 2013, 80(11), 1062–1064 CrossRef
.
- S. Lesage, M. Anheim, F. Letournel, L. Bousset, A. Honoré, N. Rozas, L. Pieri, K. Madiona, A. Dürr and R. Melki, G51D α-synuclein mutation causes a novel Parkinsonian–pyramidal syndrome, Ann. Neurol., 2013, 73(4), 459–471 CrossRef CAS
.
- P. Pasanen, L. Myllykangas, M. Siitonen, A. Raunio, S. Kaakkola, J. Lyytinen, P. J. Tienari, M. Pöyhönen and A. Paetau, A novel α-synuclein mutation A53E associated with atypical multiple system atrophy and Parkinson's disease-type pathology, Neurobiol. Aging, 2014, 35(9), 2180.e1–2180.e5 CrossRef CAS
.
- R. Krüger, W. Kuhn, T. Müller, D. Woitalla, M. Graeber, S. Kösel, H. Przuntek, J. T. Epplen, L. Schols and O. Riess, AlaSOPro mutation in the gene encoding α-synuclein in Parkinson's disease, Nat. Genet., 1998, 18(2), 106–108 CrossRef
.
- D. W. Miller, S. M. Hague, J. Clarimon, M. Baptista, K. Gwinn-Hardy, M. R. Cookson and A. B. Singleton, Alpha-synuclein in blood and brain from familial Parkinson disease with SNCA locus triplication, Neurology, 2004, 62(10), 1835–1838 CrossRef CAS
.
- B. Winner, R. Jappelli, S. K. Maji, P. A. Desplats, L. Boyer, S. Aigner, C. Hetzer, T. Loher, M. Vilar and S. Campioni, In vivo demonstration that α-synuclein oligomers are toxic, Proc. Natl. Acad. Sci. U. S. A., 2011, 108(10), 4194–4199 CrossRef CAS
.
- J. A. Wright, X. Wang and D. R. Brown, Unique copper-induced oligomers mediate alpha-synuclein toxicity, FASEB J., 2009, 23(8), 2384–2393 CrossRef CAS
.
- G. Fusco, S. W. Chen, P. T. F. Williamson, R. Cascella, M. Perni, J. A. Jarvis, C. Cecchi, M. Vendruscolo, F. Chiti, N. Cremades, L. Ying, C. M. Dobson and A. De Simone, Structural basis of membrane disruption and cellular toxicity by α-synuclein oligomers, Science, 2017, 358(6369), 1440–1443 CrossRef CAS
.
- R. Cappai, S.-L. Leck, D. J. Tew, N. A. Williamson, D. P. Smith, D. Galatis, R. A. Sharples, C. C. Curtain, A. Feda’E and R. A. Cherny, Dopamine promotes α-synuclein aggregation into SDS-resistant soluble oligomers via a distinct folding pathway, FASEB J., 2005, 19(10), 1377–1379 CrossRef CAS
.
- N. B. Cole, D. D. Murphy, T. Grider, S. Rueter, D. Brasaemle and R. L. Nussbaum, Lipid droplet binding and oligomerization properties of the Parkinson's disease protein α-synuclein, J. Biol. Chem., 2002, 277(8), 6344–6352 CrossRef CAS
.
- N. Ostrerova-Golts, L. Petrucelli, J. Hardy, J. M. Lee, M. Farer and B. Wolozin, The A53T α-synuclein mutation increases iron-dependent aggregation and toxicity, J. Neurosci., 2000, 20(16), 6048–6054 CrossRef CAS
.
- S. Hyun-Ju, L. Ju-Hyun, C.-S. Chang and K. Jongsun, Copper(II)-induced self-oligomerization of α-synuclein, Biochem. J., 1999, 340(3), 821–828 CrossRef
.
- J. M. Souza, B. I. Giasson, Q. Chen, V. M.-Y. Lee and H. Ischiropoulos, Dityrosine Cross-linking Promotes Formation of Stable α-Synuclein Polymers Implication of Nitrative and Oxidative Stress in the Pathogenesis of Neurodegenerative Synucleinopathies, J. Biol. Chem., 2000, 275(24), 18344–18349 CrossRef CAS
.
- M. Hashimoto, L. J. Hsu, Y. Xia, A. Takeda, A. Sisk, M. Sundsmo and E. Masliah, Oxidative stress induces amyloid-like aggregate formation of NACP/α-synuclein in vitro, NeuroReport, 1999, 10(4), 717–721 CrossRef CAS
.
- E. Deas, N. Cremades, P. R. Angelova, M. H. Ludtmann, Z. Yao, S. Chen, M. H. Horrocks, B. Banushi, D. Little, M. J. Devine, P. Gissen, D. Klenerman, C. M. Dobson, N. W. Wood, S. Gandhi and A. Y. Abramov, Alpha-Synuclein Oligomers Interact with Metal Ions to Induce Oxidative Stress and Neuronal Death in Parkinson's Disease, Antioxid. Redox Signaling, 2016, 24(7), 376–391 CrossRef CAS
.
- C. Wang, L. Liu, L. Zhang, Y. Peng and F. Zhou, Redox Reactions of the α-Synuclein–Cu2+ Complex and Their Effects on Neuronal Cell Viability, Biochemistry, 2010, 49(37), 8134–8142 CrossRef CAS
.
- R. M. Rasia, C. W. Bertoncini, D. Marsh, W. Hoyer, D. Cherny, M. Zweckstetter, C. Griesinger, T. M. Jovin and C. O. Fernández, Structural characterization of copper(II) binding to α-synuclein: insights into the bioinorganic chemistry of Parkinson's disease, Proc. Natl. Acad. Sci. U. S. A., 2005, 102(12), 4294–4299 CrossRef CAS PubMed
.
- A. Binolfi, L. Quintanar, C. W. Bertoncini, C. Griesinger and C. O. Fernández, Bioinorganic chemistry of copper coordination to alpha-synuclein: relevance to Parkinson's disease, Coord. Chem. Rev., 2012, 256(19), 2188–2201 CrossRef CAS
.
- V. N. Uversky, J. Li and A. L. Fink, Metal-triggered structural transformations, aggregation, and fibrillation of human α-synuclein a possible molecular link between Parkinson's disease and heavy metal exposure, J. Biol. Chem., 2001, 276(47), 44284–44296 CrossRef CAS
.
- C. R. Scherzer, J. A. Grass, Z. Liao, I. Pepivani, B. Zheng, A. C. Eklund, P. A. Ney, J. Ng, M. McGoldrick and B. Mollenhauer, GATA transcription factors directly regulate the Parkinson's disease-linked gene α-synuclein, Proc. Natl. Acad. Sci. U. S. A., 2008, 105(31), 10907–10912 CrossRef CAS
.
- D. R. Brown, Metal binding to alpha-synuclein peptides and its contribution to toxicity, Biochem. Biophys. Res. Commun., 2009, 380(2), 377–381 CrossRef CAS PubMed
.
- A. Binolfi, R. M. Rasia, C. W. Bertoncini, M. Ceolin, M. Zweckstetter, C. Griesinger, T. M. Jovin and C. O. Fernandez, Interaction of alpha-synuclein with divalent metal ions reveals key differences: a link between structure, binding specificity and fibrillation enhancement, J. Am. Chem. Soc., 2006, 128(30), 9893–9901 CrossRef CAS PubMed
.
- Bharathi and K. S. J. Rao, Thermodynamics imprinting reveals differential binding of metals to alpha-synuclein: relevance to Parkinson's disease, Biochem. Biophys. Res. Commun., 2007, 359(1), 115–120 CrossRef CAS
.
- S. C. Drew, S. Ling Leong, C. L. Pham, D. J. Tew, C. L. Masters, L. A. Miles, R. Cappai and K. J. Barnham, Cu2+ Binding Modes of Recombinant α-Synuclein-Insights from EPR Spectroscopy, J. Am. Chem. Soc., 2008, 130(24), 7766–7773 CrossRef PubMed
.
- P. Davies, D. Moualla and D. R. Brown, Alpha-synuclein is a cellular ferrireductase, PLoS One, 2011, 6(1), e15814 CrossRef CAS
.
- A. Binolfi, G. R. Lamberto, R. Duran, L. Quintanar, C. W. Bertoncini, J. M. Souza, C. Cerveñansky, M. Zweckstetter, C. Griesinger and C. O. Fernández, Site-Specific Interactions of Cu(II) with α and β-Synuclein: Bridging the Molecular Gap between Metal Binding and Aggregation, J. Am. Chem. Soc., 2008, 130(35), 11801–11812 CrossRef CAS
.
- A. Cvetkovic, A. L. Menon, M. P. Thorgersen, J. W. Scott, F. L. Poole II, F. E. Jenney Jr, W. A. Lancaster, J. L. Praissman, S. Shanmukh and B. J. Vaccaro, Microbial metalloproteomes are largely uncharacterized, Nature, 2010, 466(7307), 779–782 CrossRef CAS
.
- F. E. Jenney Jr and M. W. Adams, Rubredoxin from Pyrococcus furiosus, Methods Enzymol., 2001, 334, 45–55 CAS
.
- J. F. Kellie, R. E. Higgs, J. W. Ryder, A. Major, T. G. Beach, C. H. Adler, K. Merchant and M. D. Knierman, Quantitative measurement of intact alpha-synuclein proteoforms from post-mortem control and Parkinson's disease brain tissue by intact protein mass spectrometry, Sci. Rep., 2014, 4, 5797 CrossRef CAS
.
- A. Ohrfelt, H. Zetterberg, K. Andersson, R. Persson, D. Secic, G. Brinkmalm, A. Wallin, E. Mulugeta, P. T. Francis, E. Vanmechelen, D. Aarsland, C. Ballard, K. Blennow and A. Westman-Brinkmalm, Identification of novel alpha-synuclein isoforms in human brain tissue by using an online nanoLC-ESI-FTICR-MS method, Neurochem. Res., 2011, 36(11), 2029–2042 CrossRef
.
- G. M. Moriarty, C. A. Minetti, D. P. Remeta and J. Baum, A revised picture of the Cu(II)-alpha-synuclein complex: the role of N-terminal acetylation, Biochemistry, 2014, 53(17), 2815–2817 CrossRef CAS PubMed
.
- S. Mukherjee, E. A. Kapp, A. Lothian, A. M. Roberts, Y. V. Vasil’ev, B. A. Boughton, K. J. Barnham, W. M. Kok, C. A. Hutton, C. L. Masters, A. I. Bush, J. S. Beckman, S. G. Dey and B. R. Roberts, Characterization and Identification of Dityrosine Cross-Linked Peptides Using Tandem Mass Spectrometry, Anal. Chem., 2017, 89(11), 6136–6145 CrossRef CAS
.
- A. van Maarschalkerweerd, M. N. Pedersen, H. Peterson, M. Nilsson, T. Nguyen, T. Skamris, K. Rand, V. Vetri, A. E. Langkilde and B. Vestergaard, Formation of covalent di-tyrosine dimers in recombinant alpha-synuclein, Intrinsically Disord. Proteins, 2015, 3(1), e1071302 CrossRef CAS
.
- F. W. Studier, Protein production by auto-induction in high-density shaking cultures, Protein Expression Purif., 2005, 41(1), 207–234 CrossRef CAS
.
- B. R. Roberts, J. D. Doecke, A. Rembach, L. F. Yevenes, C. J. Fowler, C. A. McLean, M. Lind, I. Volitakis, C. L. Masters, A. I. Bush, D. J. Hare and A. r. group, Rubidium and potassium levels are altered in Alzheimer's disease brain and blood but not in cerebrospinal fluid, Acta Neuropathol. Commun., 2016, 4(1), 119 CrossRef
.
-
P. Wingfield, Protein precipitation using ammonium sulfate, Curr. Protoc. Protein Sci., 2001, Appendix 3.F.1–3.F.8 Search PubMed
.
- B. Fauvet, M. K. Mbefo, M. B. Fares, C. Desobry, S. Michael, M. T. Ardah, E. Tsika, P. Coune, M. Prudent, N. Lion, D. Eliezer, D. J. Moore, B. Schneider, P. Aebischer, O. M. El-Agnaf, E. Masliah and H. A. Lashuel, Alpha-Synuclein in central nervous system and from erythrocytes, mammalian cells, and Escherichia coli exists predominantly as disordered monomer, J. Biol. Chem., 2012, 287(19), 15345–15364 CrossRef CAS
.
- T. J. Lyons, A. Nersissian, H. Huang, H. Yeom, C. R. Nishida, J. A. Graden, E. B. Gralla and J. S. Valentine, The metal binding properties of the zinc site of yeast copper-zinc superoxide dismutase: implications for amyotrophic lateral sclerosis, J. Biol. Inorg. Chem., 2000, 5(2), 189–203 CrossRef CAS
.
- A. Lothian and B. R. Roberts, Standards for Quantitative Metalloproteomic Analysis Using Size Exclusion ICP-MS, J. Visualized Exp., 2016, 110, e53737 Search PubMed
.
- A. Lothian and B. R. Roberts, Standards for Quantitative Metalloproteomic Analysis Using Size Exclusion ICP-MS, J. Visualized Exp., 2016,(110), e53737 Search PubMed
.
- A. Shevchenko, H. Tomas, J. Havlis, J. V. Olsen and M. Mann, In-gel digestion for mass spectrometric characterization of proteins and proteomes, Nat. Protoc., 2006, 1(6), 2856–2860 CrossRef CAS
.
- D. N. Perkins, D. J. Pappin, D. M. Creasy and J. S. Cottrell, Probability-based protein identification by searching sequence databases using mass spectrometry data, Electrophoresis, 1999, 20(18), 3551–3567 CrossRef CAS PubMed
.
- T. Bartels, J. G. Choi and D. J. Selkoe, alpha-Synuclein occurs physiologically as a helically folded tetramer that resists aggregation, Nature, 2011, 477(7362), U107–U123 CrossRef
.
- M. C. Miotto, A. A. Valiente-Gabioud, G. Rossetti, M. Zweckstetter, P. Carloni, P. Selenko, C. Griesinger, A. Binolfi and C. O. Fernández, Copper binding to the N-terminally acetylated, naturally occurring form of alpha-synuclein induces local helical folding, J. Am. Chem. Soc., 2015, 137(20), 6444–6447 CrossRef CAS
.
- E. S. Luth, T. Bartels, U. Dettmer, N. C. Kim and D. J. Selkoe, Purification of alpha-synuclein from human brain reveals an instability of endogenous multimers as the protein approaches purity, Biochemistry, 2015, 54(2), 279–292 CrossRef CAS
.
- B. B. Keele Jr., J. M. McCord and I. Fridovich, Further characterization of bovine superoxide dismutase and its isolation from bovine heart, J. Biol. Chem., 1971, 246(9), 2875–2880 CAS
.
- K. Banerjee, M. Sinha, L. Pham Cle, S. Jana, D. Chanda, R. Cappai and S. Chakrabarti, Alpha-synuclein induced membrane depolarization and loss of phosphorylation capacity of isolated rat brain mitochondria: implications in Parkinson's disease, FEBS Lett., 2010, 584(8), 1571–1576 CrossRef CAS
.
- J. Gutteridge, Iron promoters of the Fenton reaction and lipid peroxidation can be released from haemoglobin by peroxides, FEBS Lett., 1986, 201(2), 291–295 CrossRef CAS
.
- A. Puppo and B. Halliwell, Formation of hydroxyl radicals from hydrogen peroxide in the presence of iron. Is haemoglobin a biological Fenton reagent?, Biochem. J., 1988, 249(1), 185–190 CrossRef CAS
.
- R. Jakes, M. G. Spillantini and M. Goedert, Identification of two distinct synucleins from human brain, FEBS Lett., 1994, 345(1), 27–32 CrossRef CAS
.
- P. K. Auluck, H. E. Chan, J. Q. Trojanowski, V. M.-Y. Lee and N. M. Bonini, Chaperone suppression of α-synuclein toxicity in a Drosophila model for Parkinson's disease, Science, 2002, 295(5556), 865–868 CrossRef CAS PubMed
.
- N. M. Bonini, Chaperoning brain degeneration, Proc. Natl. Acad. Sci. U. S. A., 2002, 99(suppl 4), 16407–16411 CrossRef CAS PubMed
.
- I. R. Brown, Heat shock proteins and protection of the nervous system, Ann. N. Y. Acad. Sci., 2007, 1113(1), 147–158 CrossRef CAS
.
- J. Klucken, Y. Shin, E. Masliah, B. T. Hyman and P. J. McLean, Hsp70 Reduces alpha-Synuclein Aggregation and Toxicity, J. Biol. Chem., 2004, 279(24), 25497–25502 CrossRef CAS
.
- M. Hashimoto, E. Rockenstein, M. Mante, M. Mallory and E. Masliah, β-Synuclein inhibits α-synuclein aggregation: a possible role as an anti-Parkinsonian factor, Neuron, 2001, 32(2), 213–223 CrossRef CAS
.
- J. B. Feldstein and D. N. Silverman, Purification and characterization of carbonic anhydrase from the saliva of the rat, J. Biol. Chem., 1984, 259(9), 5447–5453 CAS
.
- N. Ozer, M. Muftuoglu, D. Ataman, A. Ercan and I. H. Ogus, Simple, high-yield purification of xanthine oxidase from bovine milk, J. Biochem. Biophys. Methods, 1999, 39(3), 153–159 CrossRef CAS
.
- R. J. Mason, A. R. Paskins, C. F. Dalton and D. P. Smith, Copper Binding and Subsequent Aggregation of α-Synuclein Are Modulated by N-Terminal Acetylation and Ablated by the H50Q Missense Mutation, Biochemistry, 2016, 55(34), 4737–4741 CrossRef CAS
.
- L. Cortes, A. G. Wedd and Z. Xiao, The functional roles of the three copper sites associated with the methionine-rich insert in the multicopper oxidase CueO from E. coli, Metallomics, 2015, 7(5), 776–785 RSC
.
- T. R. Young, A. Kirchner, A. G. Wedd and Z. Xiao, An integrated study of the affinities of the Aβ16 peptide for Cu(I) and Cu(II): implications for the catalytic production of reactive oxygen species, Metallomics, 2014, 6(3), 505–517 RSC
.
- T. D. Alger, Copper(II) and manganese(II) effects on ribonuclease A activity, Biochemistry, 1970, 9(16), 3248–3255 CrossRef CAS
.
- C. H. Ramos and R. L. Baldwin, Sulfate anion stabilization of native ribonuclease A both by anion binding and by the Hofmeister effect, Protein Sci., 2002, 11(7), 1771–1778 CrossRef CAS
.
- P. Davies, X. Wang, C. J. Sarell, A. Drewett, F. Marken, J. H. Viles and D. R. Brown, The Synucleins Are a Family of Redox-Active Copper Binding Proteins, Biochemistry, 2011, 50(1), 37–47 CrossRef CAS
.
- C. G. Dudzik, E. D. Walter and G. L. Millhauser, Coordination features and affinity of the Cu(2)+ site in the alpha-synuclein protein of Parkinson's disease, Biochemistry, 2011, 50(11), 1771–1777 CrossRef CAS
.
- P. Wongkongkathep, J. Y. Han, T. S. Choi, S. Yin, H. I. Kim and J. A. Loo, Native Top-Down Mass Spectrometry and Ion Mobility MS for Characterizing the Cobalt and Manganese Metal Binding of alpha-Synuclein Protein, J. Am. Soc. Mass Spectrom, 2018, 29(9), 1870–1880 CrossRef CAS
.
- L. Banci, I. Bertini, S. Ciofi-Baffoni, T. Kozyreva, K. Zovo and P. Palumaa, Affinity gradients drive copper to cellular destinations, Nature, 2010, 465(7298), 645–648 CrossRef CAS PubMed
.
- Z. Xiao, J. Brose, S. Schimo, S. M. Ackland, S. La Fontaine and A. G. Wedd, Unification of the copper(I) binding affinities of the metallo-chaperones Atx1, Atox1, and related proteins: detection probes and affinity standards, J. Biol. Chem., 2011, 286(13), 11047–11055 CrossRef CAS
.
- M. T. Morgan, L. A. H. Nguyen, H. L. Hancock and C. J. Fahrni, Glutathione limits aquacopper(I) to sub-femtomolar concentrations through cooperative assembly of a tetranuclear cluster, J. Biol. Chem., 2017, 292(52), 21558–21567 CrossRef CAS
.
- T. D. Rae, P. J. Schmidt, R. A. Pufahl, V. C. Culotta and T. V. O’Halloran, Undetectable intracellular free copper: the requirement of a copper chaperone for superoxide dismutase, Science, 1999, 284(5415), 805–808 CrossRef CAS
.
- M. C. Miotto, E. E. Rodriguez, A. A. Valiente-Gabioud, V. Torres-Monserrat, A. Binolfi, L. Quintanar, M. Zweckstetter, C. Griesinger and C. O. Fernandez, Site-specific copper-catalyzed oxidation of alpha-synuclein: tightening the link between metal binding and protein oxidative damage in Parkinson's disease, Inorg. Chem., 2014, 53(9), 4350–4358 CrossRef CAS
.
- S. Indi and K. Rao, Copper-and iron-induced differential fibril formation in α-synuclein: TEM study, Neurosci. Lett., 2007, 424(2), 78–82 CrossRef PubMed
.
- A. K. Buell, C. Galvagnion, R. Gaspar, E. Sparr, M. Vendruscolo, T. P. Knowles, S. Linse and C. M. Dobson, Solution conditions determine the relative importance of nucleation and growth processes in α-synuclein aggregation, Proc. Natl. Acad. Sci. U. S. A., 2014, 111(21), 7671–7676 CrossRef CAS
.
- E. Carboni and P. Lingor, Insights on the interaction of alpha-synuclein and metals in the pathophysiology of Parkinson's disease, Metallomics, 2015, 7(3), 395–404 RSC
.
Footnotes |
† Electronic supplementary information (ESI) available. See DOI: 10.1039/c8mt00223a |
‡ These authors contributed equally. |
|
This journal is © The Royal Society of Chemistry 2019 |
Click here to see how this site uses Cookies. View our privacy policy here.