DOI:
10.1039/C9NA00471H
(Paper)
Nanoscale Adv., 2019,
1, 3973-3979
High-performance ultra-violet phototransistors based on CVT-grown high quality SnS2 flakes†
Received
1st August 2019
, Accepted 21st August 2019
First published on 21st August 2019
Abstract
van der Waals layered two-dimensional (2D) metal dichalcogenides, such as SnS2, have garnered great interest owing to their new physics in the ultrathin limit, and become potential candidates for the next-generation electronics and/or optoelectronics fields. Herein, we report high-performance UV photodetectors established on high quality SnS2 flakes and address the relatively lower photodetection capability of the thinner flakes via a compatible gate-controlling strategy. SnS2 flakes with different thicknesses were mechanically exfoliated from CVT-grown high-quality 2H-SnS2 single crystals. The photodetectors fabricated using SnS2 flakes reveal a desired response performance (Rλ ≈ 112 A W−1, EQE ≈ 3.7 × 104%, and D* ≈ 1.18 × 1011 Jones) under UV light with a very low power density (0.2 mW cm−2 @ 365 nm). Specifically, SnS2 flakes present a positive thickness-dependent photodetection behavior caused by the enhanced light absorption capacity of thicker samples. Fortunately, the responsivity of thin SnS2 flakes (e.g. ∼15 nm) could be indeed enhanced to ∼140 A W−1 under a gate bias of +20 V, reaching the performance level of thicker samples without gate bias (e.g. ∼144 A W−1 for a ∼60 nm flake). Our results offer an efficient way to choose 2D crystals with controllable thicknesses as optimal candidates for desirable optoelectronic devices.
Introduction
Two-dimensional (2D) materials, such as graphene since its first isolation from bulk crystals, have created opportunities to access novel features at reduced dimensionality.1–3 Inspired by this, there has been growing interest to find new 2D structures, because fundamentally and technologically interesting properties (charge carriers and photons are confined in a 2D plane, etc.) have been identified in the ultrathin limit, but most attention so far has been paid to layered transition metal dichalcogenides (TMDs).4–7 Furthermore, graphene shows a zero-band gap which traditionally has limited its application in optoelectronic devices.8 In addition, layered semiconducting TMDs with a general description of MX2 (a metal atom, M = Mo, W, Sn, etc. is sandwiched between two adjacent chalcogen layers X = S, S, Te, etc.) have exhibited extraordinary properties in many fields, emerging as suitable candidates for next-generation electronic or photoelectronic systems beyond the current silicon era.9–13
The surprising and appealing characteristics found in the low-dimensional limit stimulate the exploration of other van der Waals layered materials.14–16 Nowadays, various layered semiconductors can be prepared via top-down (e.g. mechanical exfoliation) or bottom-up (e.g. chemical vapour deposition, CVD) methods.17,18 Nevertheless, in view of sustainable development, earth-abundant 2D materials are more essential for widespread use in modern devices. Tin disulfide (SnS2, belonging to the IV–VIA group) comprises earth-abundant constituents (Sn and S), which could vastly support the industrial requirements.19 Bulk SnS2 crystals (usually representing a bandgap of 2.1–2.31 eV) have long been explored for possible applications in photovoltaics and photoelectrochemistry.20,21 In addition, recent evidence has already pointed out the fascinating progress in applying 2D SnS2 structures in lithium ion batteries,22 field-effect devices23 and photodetectors,24 benefitting from the simple exfoliation from bulk crystals and the controlled bottom-up synthesis.25,26 In particular, due to a considerable absorption coefficient (106 cm−1),27 light sensors using 2D SnS2 structures (most are obtained by CVD) exhibit superior UV-vis sensing performance such as high responsivity and good stability.28–30 Note that, however, the thickness dependent responsivity of 2D material (including SnS2 flakes) based photodetectors (i.e. the relatively lower photodetection capability in thinner flakes) may make them inappropriate for the upcoming smart systems with high integration density because the chip manufacturing process in development requires thinner (monolayer of few-layer) semiconductor channels.31–33 Therefore, some prospective strategies should be proposed to address this problem and make these prototypes appealing for practical applications. Unfortunately, this issue has not been well considered in previous optoelectronic applications.
In this paper, we have achieved high-performance UV photodetectors established on SnS2 flakes and employed a gate-tunable strategy to address the challenge posed by the positive thickness-dependent light sensing behaviour of 2D materials. SnS2 flakes with different thicknesses (that can be thinned to ∼7 nm in the work) were mechanically exfoliated from CVT-grown high-quality 2H-SnS2 single crystals. We describe several characterization methods to identify the phase and microstructure of these multilayer SnS2 samples. The photodetectors fabricated by using individual SnS2 flakes reveal a desired response performance (Rλ ≈ 112 A W−1, EQE ≈ 3.7 × 104%, and D* ≈ 1.18 × 1011 Jones) under UV light with a very low power density (0.2 mW cm−2 @ 365 nm). Specifically, the SnS2 flakes present a relatively low photodetection ability in the thinner flakes. But it is found that the responsivity of thin flakes (e.g. ∼15 nm) could be enhanced to ∼140 A W−1 aided by a positively gated bias (+20 V), and it is comparable to the performance of a non-gated ∼60 nm flake. This indeed provides a potential way to address the positive thickness-dependent detection capability mainly caused by the enhanced light absorption capacity of thicker samples, and our findings imply that such earth-abundant and environmentally friendly tin-based chalcogenides are desirable for sustainable “green” optoelectronics applications.
Experimental section
Preparation of multilayer SnS2 flakes
The starting materials here are SnS2 bulk crystals, grown via a chemical vapor transport (CVT) route with pure iodine as the transport agent. The pre-mixed powders of Sn (99.99%, Aladdin) and S (99.99%, Alfa Aesar) at a stoichiometric ratio of 1
:
2 with additional iodine (99.8%, Aladdin, 5 mg cm−3) were vacuum sealed (>10−4 Torr) in a quartz tube. The quartz tube was then placed in a two-zone furnace. The reactant zone was slowly heated up (∼10 h) to 800 °C while the other end was set to 750 °C. The growth process was maintained for ∼10 h, followed by a naturally cooling process down to room temperature. Afterwards, strip-like products were obtained. Thin SnS2 flakes were mechanically exfoliated from the as-synthesized crystals aided by adhesive tape and then dry-transferred onto a freshly cleaned SiO2/Si substrate (with a dielectric layer, ∼300 nm thick SiO2). Thin samples were roughly identified using an optical microscope (Olympus, CX41) in combination with a charge-coupled device.
Materials characterization
The phases of the crystals were characterized by X-ray diffraction (XRD) on a Rigaku Miniflex 600x powder diffractometer. XPS measurements were performed using a Thermo ESCALAB 250XI X-ray photoelectron spectrometer. The thicknesses of the exfoliated SnS2 samples were measured by atomic force microscopy (AFM, Agilent 5500). A home-built Raman spectroscope/microscope (iHR320, Horiba) was utilized to acquire Raman spectra and spatially resolved Raman maps with an incident laser of 532 nm while employing a 405 nm light for photoluminescence (PL) measurements. A transmission electron microscope (TEM, JEM-2100F) was employed to evaluate the morphologies and crystal structure of thin SnS2 flakes. The UV-vis spectrum was measured using a spectrophotometer (MPC-3100, Shimadzu).
Device fabrication and measurements
The fabrication of photodetectors based on individual SnS2 flakes relies on a standard UV lithography (URE-2000/25) process followed by thermal evaporation (JSD 300) of desired electrode metals (Cr/Au, ∼10/60 nm). The photoresponse measurements were executed on a probe station (ZFT-50T) equipped with two sourcemeters (Model 2450, Keithley). Light sources (THORLABS) of different wavelengths with tunable power were applied in the photodetection tests.
Results and discussion
To get SnS2 samples with varied thicknesses, we employed tape-assisted mechanical isolation from raw crystals synthesized via a chemical vapour transport (CVT) approach. Previous research suggests that SnS2 occurs as different polytypes associated with different interlayer stacking of S–Sn–S layers; a low-temperature (<800 °C) synthesis process tends to produce the 2H-polytype.34,35Fig. 1a illustrates the 3D structure model of 2H-SnS2; the S–Sn–S atomic planes (the distance between adjacent planes is ∼0.6 nm) with covalent bonding are held together by weak van der Waals force.36 In this work, SnS2 crystals were prepared via a CVT process in a two-zone tube furnace, as schematically shown in Fig. 1b (more information has been provided in the Experimental section). During the CVT growth, gas transport is realized by temperature gradient and thus impact on the final products (Fig. S1†). In this work, a 50 °C temperature gradient is in favour of growing high quality SnS2 single crystals, in agreement with a previous report,37 and large sized crystals (given in the inset of Fig. 1c, lateral size > 3 mm) with a clean surface could be found at the lower temperate zone. The phase structure of the crystals was identified through the powder X-ray diffraction (XRD) technology, as depicted in Fig. 1c. The products exhibit a hexagonal structure (JCPDS PDF number 23-0677, a = b = 0.36 nm, c = 0.59 nm). Strong diffraction reflections emerging around ∼15.0°, ∼30.3° and ∼46.1° are indexed to the (0001), (0002) and (0003) planes, respectively. The predominance of the (000N) (N = 0, 1, 2, etc.) peaks suggests that the Z-direction with the (0001) plane as the basal plane is the preferential orientation for these growing SnS2 crystals.38 The valence states of the SnS2 crystals were characterized by X-ray photoelectron spectroscopy (XPS). The spectra have been calibrated by employing absorbed C (1s) as the reference. Expected Sn and S elements from these crystals were detected in the XPS survey (Fig. 1d). Two peaks at 486.5 and 495.1 eV (Fig. 1e) originate from Sn3d5/2 and Sn3d3/2 of Sn4+.39Fig. 1f illustrates the high-resolution core XPS spectrum of S 2p (∼162.6 eV), an indication of the existence of S2p3/2 and S2p1/2 orbitals.40 These results evidence the formation of layered SnS2 crystals with high quality.
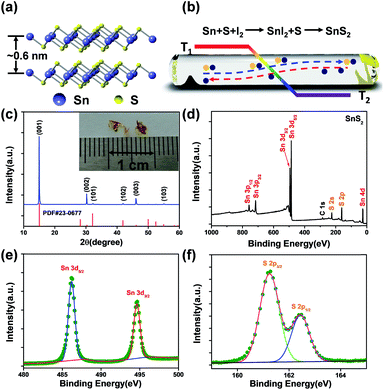 |
| Fig. 1 Synthesis of SnS2 crystals. (a) Crystal structure (side view) of layered SnS2. (b) Schematic diagram showing the CVT process. (c) XRD patterns of the as-synthesized products, inset: the photograph of SnS2 single crystals. (d) XPS survey of SnS2 crystals, (e) Sn3d XPS and (f) high resolution S 2p XPS of SnS2 single crystals. | |
Thin flakes with varied thicknesses (Fig. 2a) were obtained through mechanical exfoliation from large crystals exceeding 10 μm (limited by the AFM scanning range) accompanied by a clean surface and pristine state. The height of multilayer SnS2 flakes often presents lateral dimension changes shown in Fig. 2b measured at the edge between the thicker and thinner part indicating a thickness of ∼7 nm (corresponding to about ten layers). Raman spectroscopy was employed to quantify and map SnS2 flakes. Previous reports suggest the existence of different crystal polytypes for layered SnS2 crystals, 4H- and 2H-phases, respectively.41 The most intense Raman peak for the 4H polytype emerges at 313.5 cm−1, relative to the joint contribution of A1 and E phonon modes (this peak is very close to the A1g mode of 2H-SnS2 at 315 cm−1), while the E-mode generates a doublet at 200 and 214 cm−1, respectively.19 In parallel, in 2H-SnS2 crystals, the Eg mode generates a single, intense line around 205 cm−1, allowing a facile distinction against the 4H polytype.28 The observed Raman active modes in Fig. 2c, i.e. two main peaks at 205 (Eg, in plane) and 315 cm−1 (A1g, out of plane) occur in the thick flakes (>200 nm, the inset of Fig. 2c), hence, providing an unambiguous fingerprinting of 2H polytypic crystals in this work. Here, the Eg modes weaken and become unobservable with the reduction of flake thickness (down to the nanometer level), which could be presumably attributed to the reduction of in-plane scattering centers in the ultrathin SnS2 flakes.42,43 But the A1g (out of plane) mode illustrates a significant increase in the peak intensity with the increasing thickness (Fig. 2d), probably arising from the enhanced light absorption capacity of the thicker samples,44,45 which may have an influence on the device performance. Raman mapping (Fig. 2c) of a SnS2 flake (the optical image is depicted in Fig. 2d) using the characteristic line at 315 cm−1 demonstrates its uniform polytype. 2H-SnS2 flakes exhibit isotropy in the (0001) plane as confirmed by the polarized Raman characterization of the A1g vibration mode. Therefore, the origination dependent photo-responsivity should not be a problem for their application in photodetection.46 The PL spectra of 2D SnS2 flakes in Fig. 2e consist of a single feature, attributed to the nature of their indirect band-gap structure, with a value of ∼2.20 eV similar to the reported data of 2H-SnS2.28 Moreover, the peak shifts to lower energies with increasing thickness (Fig. 2f). During PL measurements, holes will combine with the photo-excited electrons via Coulomb interactions with the binding energy lying in the band-gap region; however, strong spatial confinement and a reduced screening effect in ultrathin samples could result in a significantly enhanced excitonic effect causing a blue shift in the bandgap.47
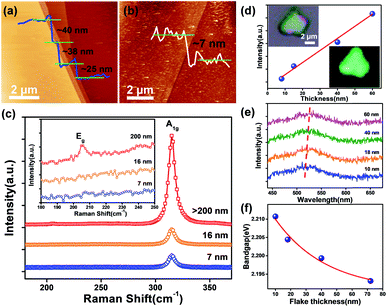 |
| Fig. 2 Characterization of SnS2 flakes. (a and b) Typical AFM images of ultrathin SnS2 flakes acquired via mechanical exfoliation. (c) Thickness dependent Raman spectra of SnS2 flakes, inset: enlarged view of the characteristic peak at ∼205 cm−1. (d) Change in the intensity of the A1g peak with layer thickness, inset: an optical image of a SnS2 flake and the corresponding Raman map of the A1g mode. (e) PL spectrum of SnS2 with different thicknesses. (f) The layer-dependent bandgap of few-layer SnS2. | |
The microscopic structure and the compositions of the 2D SnS2 crystals were determined using a transmission electron microscope (TEM) and an energy-dispersive X-ray detector (EDX). Fig. 3a shows a low magnification view (bright field) of a single flake with no observable terraces. A direct view of the lattice structure (Fig. 3b, reflected by the HRTEM image of the labeled region in Fig. 3a) suggests the hexagonal arrangement (atomic mode given on the top) of Sn and S atoms in 2H SnS2 crystals. The plane distance of ∼0.32 nm well matches the d-spacing of the {10 − 10} planes for hexagonal phase SnS2.48 The selected area electron diffraction (SAED) pattern (obtained by applying incident electrons parallel to the c-axis) in Fig. 3c shows well-sequenced diffraction spots with a six-fold symmetry, indicating high-quality crystallinity of this crystal caused by a vertically stacking layer plane along the [0001] direction. The composition of the crystals was verified by adopting energy-dispersive X-ray spectroscopy (EDX). EDX mapping results in Fig. 3d and e suggest the homogeneous distribution of Sn and S elements in the flake. In parallel, the EDX spectrum (Fig. 3f) clearly reveals signals of Sn and S with an approximate atomic ratio of 2
:
1. The aforementioned demonstrations signify the high purity of the single-crystalline SnS2 flakes.
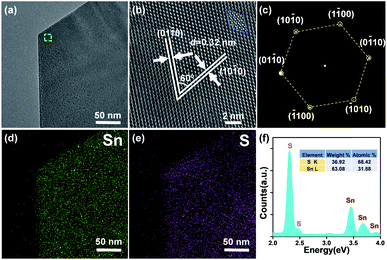 |
| Fig. 3 TEM identification of SnS2 flakes. (a) Low-magnification TEM image of an ultrathin SnS2 flake. (b) High resolution TEM characterization with atomic mode on the top and (c) the corresponding SAED pattern of the SnS2 flake. EDX elemental mapping of (d) Sn and (e) S revealing uniform distributions. (f) EDX spectrum of the SnS2 flake; the inset illustrates the atomic ratio of Sn and S. | |
Photodetectors established on individual flakes were employed to systematically estimate the optoelectronic properties of the SnS2 crystals. The set-up diagram of a two-terminal light sensor is illustrated in Fig. S3a,† where the incident light is perpendicular to the SnS2 flake. Fig. S3b† and 4a present the current–voltage (IDS–VGS) characteristics of the representative SnS2 light sensor in darkness and under illumination with varied wavelengths. The linear curves result from the near ohmic barrier between the Cr/Au electrodes and SnS2 channel. The plot of responsivity (Fig. 4b, Rλ = Iph/PS, Iph = Ilight − Idark, S refers to the activated area of ∼5.17 μm2 and P is the power intensity of incident light, VDS = 1 V) versus wavelength provides a quantitative assessment of the photoresponse ability of the SnS2 flake. The optical image of the SnS2 channel with a thickness of ∼15 nm and a length of ∼3 μm is illustrated in the inset. The cut-off wavelength is about 550 nm, close to its deduced bandgap, Eindirect ≈ 2.18 eV from the UV-vis absorption spectrum in Fig. S4.†29 The light sensor exhibits high photo responsivity in the ultra-violet range. The stability and reproducibility of the SnS2 based photodetector (Fig. 4c, measured at a bias of 1 V) are uncovered via tracking current under periodic illumination of 0.2 mW cm−2 @ 365 nm. Enlarged views of the rising and decay sides are illustrated in Fig. S3c,† from which the response time (τrising) and recovery time (τdecay) are calculated to be 40 and 160 ms, respectively. Fig. 4d displays the dependence of photocurrent on light intensity, which can be well described by a power law, Iph ∼ Pβ, where β is an exponent determined by trap states on the surface of the photo-sensitive media.49 In general, a low power intensity would benefit the occupation of surface states by holes separated from photo-induced electron–hole pairs, followed by a rapid recombination with the negatively charged oxygen. But abundant electron–hole pairs will be generated under a higher light intensity. The reduction of the hole-trap states at the surface until the complete occupation of surface traps will contribute to faster recombination (in several picoseconds) between extra charges; subsequently, these fresh pairs will not contribute to the charge transfer process, but form non-radiative carrier-recombination centers, thus leading to a decline (Fig. 4e) of responsivity and quantum efficiency (EQE = hcRλ/eλ, h is Planck's constant).50,51 The sub-linear behaviour in Fig. 4d with a fitting value β ∼ 0.98 (very close to 1) may be associated with a low concentration of traps or defects in these SnS2 flakes.52 The parameters of our sensors are comparable or superior to those of documented 2D SnS2 and other 2D material-based UV sensors as summarized in Table 1, potentially offered by the high-quality, large specific surface area.53 In addition, we find a positive thickness-dependent photodetection capability of these 2D SnS2 flakes with a similar active zone (Rλ up to 144 A W−1 for a ∼60 nm thick SnS2 flake, in Fig. 4f), which has also been documented in other research.54 For a given wavelength range, the photocurrent of photosensitive flakes is proportional to the absorption if we consider a constant namely the internal quantum efficiency (η), which can be expressed as Iph = α × d × η, where α is the absorption and d is the thickness of the flake.55 Therefore, the dependence of photoresponsivity on flake thickness may originate from enhanced absorption in thicker samples.56
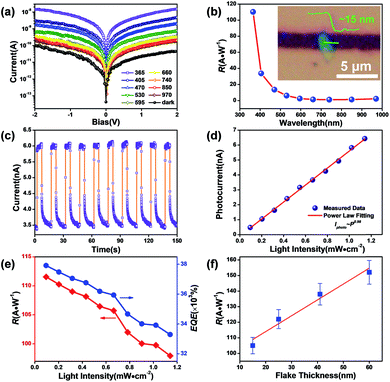 |
| Fig. 4 Photodetectors established on ultrathin SnS2 flakes. (a) I–V characteristics measured in darkness and under incident light of varied wavelengths (under comparable light intensity). (b) Spectral responsivity of the SnS2 flake based photodetector, inset: the representative two-terminal devices designed on a thin SnS2 flake. (c) Time resolved current of the light sensor measured at VDS = 1 V under 365 nm (0.2 mW cm−2). (d) Power law fitting photocurrents versus light intensities. (e) Responsivity (Rλ) and external quantum efficiency (EQE) plotted as a function of light intensity. (f) Thickness dependent responsivity of SnS2 flake based devices. | |
Table 1 Comparison of the 2D UV photodetector performance with those reported by others. T: thickness, ME: mechanical exfoliation, D*: detectivity
Material |
Synthesis |
Electrode |
T [nm] |
λ [nm] |
R
λ
[A W−1] |
EQE [%] |
D* [Jones] |
τ [ms] |
Ref. |
WO3 |
CVD |
Cr/Au |
12 |
365 |
293 |
997 |
— |
40/80 |
57
|
β-Ga2O3 |
Oxidant |
Cr/Au |
6 |
254 |
3.3 |
1.6 × 103 |
4 × 1012 |
30/60 |
58
|
Bi2Te3 |
ME |
Pt |
— |
325 |
26.82 |
102 |
1.29 × 109 |
280/1600 |
59
|
SnS2 |
CVD |
Ti/Au |
— |
390 |
— |
150 |
— |
8/150 |
60
|
SnS2 |
CVD |
Cr/Au |
10 |
350 |
260 |
9.3 × 104 |
1.9 × 1010 |
20/16 |
28
|
SnS2 |
CVD |
Cr/Au |
114 |
100–800 |
1.568 |
480.1 |
— |
42/40 |
61
|
SnS2 |
ME |
Cr/Au |
15 |
365 |
112 |
3.7 × 104 |
1.18 × 1011 |
40/160 |
This work |
The positive thickness-dependent photodetection capability may be a challenge for the system-on-a-chip design where a thinner channel is required. Previous work employed the surface sensitization of SnS2 nanosheets using a 2 nm thick HfO2 nanolayer grown by atomic layer deposition (ALD).54 However, this inevitably leads to a complex procedure or a higher production cost. A phototransistor, in which the increase of gate voltage gives rise to an increase in the channel current, may pave an alternative way to address this issue.62,63 Here, the emphasis has been placed on the device (as schematically shown in the inset of Fig. 5b) performance operating under illumination (0.2 mW cm−2 @ 365 nm). The light output characteristics (thickness ∼ 15 nm) are displayed in Fig. 5a. The output and transfer characteristics measured in darkness are illustrated in Fig. S5.† Both the SnS2 channels reveal a typical n-type semiconducting behaviour. The correlation between the channel current and back-gate bias (IDS–VGS curves) under illumination and dark conditions is plotted in Fig. 5b. An increase in the channel current can be found as VGS increases, possibly due to the leading role of photo-generation in comparison with tunneling or thermionic currents.64 The false-color plot in Fig. 5c provides direct evidence that higher photocurrent could be achieved under a high (positive) gate bias. Thereby, the indicators of light sensors relying on SnS2 flakes, such as responsivity and detectivity (Fig. 5d), could be further improved (up to 140 A W−1) through changing the gate voltage. Under a positive gate bias, the Fermi level in the (n-type) semiconductor will approach the conduction band and lead to a reduction of barrier height; as a result, more photo-excited charges could overcome the gate barrier and contribute to an increased carrier density.65,66 Hence, this compatible manufacturing approach could address the positive thickness-dependent sensing capability caused by the enhanced absorption capacity in thicker samples.
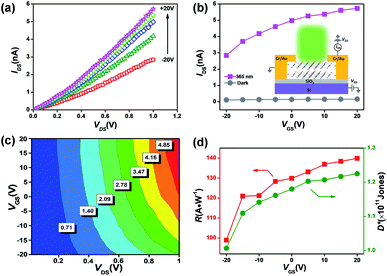 |
| Fig. 5 Phototransistors based on SnS2 flakes. (a) Output characteristics of the SnS2 (∼15 nm) transistors operated under incident light of 0.2 mW cm−2 @ 365 nm. (b) IDS–VGS curves under 365 nm light and in darkness at VDS = 1 V, inset: the layout of the phototransistor. (c) False-color plot of the SnS2 phototransistor exposed to incident light, where the color reflects the intensity of the measured photocurrent. (d) Photoresponsivity and detectivity measured at VDS = 1 V as a function of VGS. | |
Conclusions
In summary, we report high-performance UV photodetectors established on SnS2 flakes and address the relatively low photodetection capability in the thinner flakes via a compatible gate-tunable route. Multilayer SnS2 flakes (that can be thinned to ∼7 nm in this work) were mechanically isolated from CVT-grown high-quality 2H-SnS2 single crystals whose components are both inexpensive and earth-abundant. The phase and microstructure were unambiguously identified through several characterization techniques, including XRD, XPS, AFM, Raman and TEM. When exposed to UV illumination with a very low power density (0.2 mW cm−2 @ 365 nm), the light sensors using SnS2 flakes exhibit high responsivity (112 A W−1), EQE (3.7 × 104%) and detectivity (1.18 × 1011 Jones), comparable or superior to those of reported SnS2 and other 2D material-based UV photodetectors. Most importantly, SnS2 flakes present a positive thickness-dependent photodetection behaviour, possibly attributed to the enhanced light absorption capacity of thicker samples. However, the responsivity of thinner flakes (e.g. ∼15 nm) can be further improved (up to 140 A W−1) under a gate bias of +20 V, comparable with the performance of a non-gated thick flake (∼144 A W−1 for a ∼60 nm flake). Hence, our results offer an efficient way to choose 2D materials with an optimal thickness, and such earth-abundant and environmentally friendly tin-based chalcogenides are highly desirable for sustainable “green” optoelectronics applications.
Conflicts of interest
There are no conflicts to declare.
Acknowledgements
This work was supported by the National Natural Science Foundation of China (51803043, 51872069 and 11847076) and Zhejiang Provincial Natural Science Foundation of China (LY18F040006 and LQ19E020004).
Notes and references
- L. Ju, L. Wang, T. Cao, T. Taniguchi, K. Watanabe, S. G. Louie, F. Rana, J. Park, J. Hone, F. Wang and P. L. McEuen, Science, 2017, 358, 907–910 CrossRef CAS PubMed.
- X. W. Yu, H. H. Cheng, M. Zhang, Y. Zhao, L. T. Qu and G. Q. Shi, Nat. Rev. Mater., 2017, 2, 17046 CrossRef CAS.
- Y. D. Zhao, S. Bertolazzi and P. Samorì, ACS Nano, 2019, 13, 4814–4825 CrossRef CAS PubMed.
- J. D. Zhou, J. H. Lin, X. W. Huang, Y. Zhou, Y. Chen, J. Xia, H. Wang, Y. Xie, H. M. Yu, J. C. Lei, D. Wu, F. C. Liu, Q. D. Fu, Q. S. Zeng, C. H. Hsu, C. L. Yang, L. Lu, T. Yu, Z. X. Shen, H. Lin, B. I. Yakobson, Q. Liu, K. Suenaga, G. T. Liu and Z. Liu, Nature, 2018, 556, 355–359 CrossRef CAS PubMed.
- M. S. Stark, K. L. Kuntz, S. J. Martens and S. C. Warren, Adv. Mater., 2019, 31, 1808213 CrossRef PubMed.
- F. Wang, Z. X. Wang, T. A. Shifa, Y. Wen, F. M. Wang, X. Y. Zhan, Q. S. Wang, K. Xu, Y. Huang, L. Yin, C. Jiang and J. He, Adv. Funct. Mater., 2017, 27, 1603254 CrossRef.
- R. G. Mendes, J. B. Pang, A. Bachmatiuk, H. Q. Ta, L. Zhao, T. Gemming, L. Fu, Z. F. Liu and M. H. Rummeli, ACS Nano, 2019, 13, 978–995 CAS.
- K. S. Novoselov, A. Mishchenko, A. Carvalho and A. H. Castro Neto, Science, 2016, 353, aac9439 CrossRef CAS PubMed.
- M. Q. Zeng, Y. Xiao, J. X. Liu, K. N. Yang and L. Fu, Chem. Rev., 2018, 118, 6236–6296 CrossRef CAS PubMed.
- Y. W. Sheng, T. X. Chen, Y. Lu, R. J. Chang, S. Sinha and J. H. Warner, ACS Nano, 2019, 13, 4530–4537 CrossRef CAS PubMed.
- X. Zhou, Q. Zhang, L. Gan, H. Q. Li, J. Xiong and T. Y. Zhai, Adv. Sci., 2016, 3, 1600177 CrossRef PubMed.
- F. G. Yan, Z. M. Wei, X. Wei, Q. S. Lv, W. K. Zhu and K. Y. Wang, Small Methods, 2018, 2, 1700349 CrossRef.
- H. Zhan, D. Guo and G. X. Xie, Nanoscale, 2019, 11, 13181–13212 RSC.
- C. Jin, E. Y. Ma, O. Karni, E. C. Regan, F. Wang and T. F. Heinz, Nat. Nanotechnol., 2018, 13, 994–1003 CrossRef CAS PubMed.
- S. Z. Zhu, P. Pochet and H. T. Johnson, ACS Nano, 2019, 13, 6925–6931 CrossRef CAS PubMed.
- H. Yuan, X. Y. Zhou, Y. Cao, Q. Bian, Z. Y. Zhang, H. G. Sun, S. J. Li, Z. B. Shao, J. Hu, Y. L. Zhu, Z. Q. Mao, W. Ji and M. H. Pan, npj 2D Mater. Appl., 2019, 3, 12 CrossRef.
- Z. Y. Cai, B. L. Liu, X. L. Zou and H. M. Cheng, Chem. Rev., 2018, 118, 6091–6133 CrossRef CAS PubMed.
- D. C. Geng and H. Y. Yang, Adv. Mater., 2018, 30, 1800865 CrossRef PubMed.
- Y. Huang, E. Sutter, J. T. Sadowski, M. Cotlet, O. L. A. Monti, D. A. Racke, M. R. Neupane, D. Wickramaratne, R. K. Lake, B. A. Parkinson and P. Sutter, ACS Nano, 2014, 8, 10743–10755 CrossRef CAS PubMed.
- B. A. Parkinson, Langmuir, 1988, 4, 967–976 CrossRef CAS.
- B. Fotouhi, A. Katty and O. Gorochov, J. Electrochem. Soc., 1985, 132, 2181–2184 CrossRef CAS.
- B. Luo, B. Y. Fang, B. Wang, J. S. Zhou, H. H. Song and L. J. Zhi, Energy Environ. Sci., 2012, 5, 5226–5230 RSC.
- R. J. Chang, H. J. Tan, X. C. Wang, B. Porter, T. X. Chen, Y. W. Sheng, Y. Q. Zhou, H. F. Huang, H. Bhaskaran and J. H. Warner, ACS Appl. Mater. Interfaces, 2018, 10, 13002–13010 CrossRef CAS PubMed.
- G. X. Su, V. G. Hadjiev, P. E. Loya, J. Zhang, S. D. Lei, S. Maharjan, P. Dong, P. M. Ajayan, J. Lou and H. B. Peng, Nano Lett., 2015, 15, 506–513 CrossRef CAS PubMed.
- Y. Hu, T. Chen, X. Q. Wang, L. B. Ma, R. P. Chen, H. F. Zhu, X. Yuan, C. Z. Yan, G. Y. Zhu, H. L. Lv, J. Liang, Z. Jin and J. Liu, Nano Res., 2017, 10, 1434–1447 CrossRef CAS.
- J. H. Ahn, M. J. Lee, H. Heo, J. H. Sung, K. Kim, H. Hwang and M. H. Jo, Nano Lett., 2015, 15, 3703–3708 CrossRef CAS PubMed.
- Q. Zhang, X. Li, Z. W. He, M. X. Xu, C. C. Jin and X. Zhou, J. Phys. D: Appl. Phys., 2019, 52, 303002 CrossRef.
- X. Zhou, Q. Zhang, L. Gan, H. Q. Li and T. Y. Zhai, Adv. Funct. Mater., 2016, 26, 4405–4413 CrossRef CAS.
- D. Yang, B. Li, C. Hu, H. Deng, D. D. Dong, X. K. Yang, K. K. Qiao, S. J. Yuan and H. S. Song, Adv. Opt. Mater., 2016, 4, 419–426 CrossRef CAS.
- Y. R. Tao, X. C. Wu, W. Wang and J. N. Wang, J. Mater. Chem. C, 2015, 3, 1347–1353 RSC.
- M. Bernardi, M. Palummo and J. C. Grossman, Nano Lett., 2013, 13, 3664–3670 CrossRef CAS PubMed.
- M. Z. Zhong, Q. L. Xia, L. F. Pan, Y. Q. Liu, Y. B. Chen, H. X. Deng, J. B. Li and Z. M. Wei, Adv. Funct. Mater., 2018, 28, 1802581 CrossRef.
- K. Wang, C. C. Wu, D. Yang, Y. Y. Jiang and S. Priya, ACS Nano, 2018, 12, 4919–4929 CrossRef CAS PubMed.
- R. S. Mitchell, Y. Fujiki and Y. Ishizawa, J. Cryst. Growth, 1982, 57, 273–279 CrossRef CAS.
- B. Palosz, W. Steurer and H. Schulz, Acta Crystallogr., Sect. B: Struct. Sci., 1990, 46, 449–455 CrossRef.
- B. Wang, S. P. Zhong, Z. B. Zhang, Z. Q. Zheng, Y. P. Zhang and H. Zhang, Appl. Mater. Today, 2019, 15, 115–138 CrossRef.
- A. L. Li, Q. X. Chen, P. P. Wang, Y. Gan, T. L. Qi, P. Wang, F. D. Tang, J. Z. Wu, R. Chen, L. Y. Zhang and Y. P. Gong, Adv. Mater., 2018, 31, 1805656 CrossRef PubMed.
- J. Xia, D. D. Zhu, L. Wang, B. Huang, X. Huang and X. M. Meng, Adv. Funct. Mater., 2015, 25, 4255–4261 CrossRef CAS.
- Y. Q. Lei, S. Y. Song, W. Q. Fan, Y. Xing and H. J. Zhang, J. Phys. Chem. C, 2009, 113, 1280–1285 CrossRef CAS.
- X. Xiong, Q. Zhang, L. Gan, X. Zhou, X. N. Xing, H. Q. Li and T. Y. Zhai, Nano Res., 2016, 9, 3848–3857 CrossRef CAS.
- A. J. Smith, P. E. Meek and W. Y. Liang, J. Phys. C: Solid State Phys., 1977, 10, 1321–1333 CrossRef CAS.
- B. H. Qu, C. Z. Ma, G. Ji, C. H. Xu, J. Xu, Y. S. Meng, T. H. Wang and J. Y. Lee, Adv. Mater., 2014, 26, 3854–3859 CrossRef CAS PubMed.
- S. D. Lei, L. H. Ge, Z. Liu, S. Najmaei, G. Shi, G. You, J. Lou, R. Vajtai and P. M. Ajayan, Nano Lett., 2013, 13, 2777–2781 CrossRef CAS.
- I. Vlassiouk, S. Smirnov, M. Regmi, S. P. Surwade, N. Srivastava, R. Feenstra, G. Eres, C. Parish, N. Lavrik, P. Datskos, S. Dai and P. Fulvio, J. Phys. Chem. C, 2013, 117, 18919–18926 CrossRef CAS.
- W. Lu, H. Nan, J. Hong, Y. Chen, C. Zhu, Z. Liang, X. Ma, Z. Ni, C. Jin and Z. Zhang, Nano Res., 2014, 7, 853–859 CrossRef CAS.
- R. Y. Wang, F. Y. Zhou, L. Lv, S. S. Zhou, Y. W. Yu, F. W. Zhuge, H. Q. Li, L. Gan and T. Y. Zhai, CCS Chem., 2019, 1, 268–277 Search PubMed.
- X. L. Li, W. P. Han, J. B. Wu, X. F. Qiao, J. Zhang and P. H. Tan, Adv. Funct. Mater., 2017, 27, 1604468 CrossRef.
- Y. F. Sun, H. Cheng, S. Gao, Z. H. Sun, Q. H. Liu, Q. Liu, F. C. Lei, T. Yao, J. F. He, S. Q. Wei and Y. Xie, Angew. Chem., Int. Ed., 2012, 51, 8806–8810 CrossRef PubMed.
- X. Zhou, Q. Zhang, L. Gan, X. Li, H. Q. Li, Y. Zhang, D. Golberg and T. Y. Zhai, Adv. Funct. Mater., 2016, 26, 704–712 CrossRef CAS.
- D. Shao, J. Gao, P. Chow, H. Sun, G. Xin, P. Sharma, J. Lian, N. A. Koratkar and S. Sawyer, Nano Lett., 2015, 15, 3787–3792 CrossRef CAS PubMed.
- F. K. Wang, T. Gao, Q. Zhang, Z. Y. Hu, B. Jin, L. Li, X. Zhou, H. Q. Li, G. Van Tendeloo and T. Y. Zhai, Adv. Mater., 2019, 31, 1806306 CrossRef PubMed.
- X. N. Xing, Q. Zhang, Z. Huang, Z. J. Lu, J. B. Zhang, H. Q. Li, H. B. Zeng and T. Y. Zhai, Small, 2016, 12, 874–881 CrossRef CAS PubMed.
- S. C. Kung, W. Xing, W. E. van der Veer, F. Yang, K. C. Donavan, M. Cheng, J. C. Hemminger and R. M. Penner, ACS Nano, 2011, 5, 7627–7639 CrossRef CAS PubMed.
- X. S. Jia, C. C. Tang, R. H. Pan, Y. Z. Long, C. Z. Gu and J. J. Li, ACS Appl. Mater. Interfaces, 2018, 10, 18073–18081 CrossRef CAS PubMed.
- S. D. Lei, L. H. Ge, S. Najmaei, A. George, R. Kappera, J. Lou, M. Chhowalla, H. Yamaguchi, G. Gupta, R. Vajtai, A. D. Mohite and P. M. Ajayan, ACS Nano, 2014, 8, 1263–1272 CrossRef CAS PubMed.
- Y. D. Zhao, J. S. Qiao, Z. H. Yu, P. Yu, K. Xu, S. P. Lau, W. Zhou, Z. Liu, X. N. Wang, W. Ji and Y. Chai, Adv. Mater., 2017, 29, 1604230 CrossRef.
- J. Z. Liu, M. Z. Zhong, J. B. Li, A. L. Pan and X. L. Zhu, Mater. Lett., 2015, 148, 184–187 CrossRef CAS.
- W. Feng, X. N. Wang, J. Zhang, L. F. Wang, W. Zheng, P. A. Hu, W. W. Cao and B. Yang, J. Mater. Chem. C, 2014, 2, 3254–3259 RSC.
- A. Sharma, A. K. Srivastava, T. D. Senguttuvan and S. Husal, Sci. Rep., 2017, 7, 17911 CrossRef PubMed.
- G. L. Ye, Y. J. Gong, S. D. Lei, Y. M. He, B. Li, X. Zhang, Z. H. Jin, L. L. Dong, J. Lou, R. Vajtai, W. Zhou and P. M. Ajayan, Nano Res., 2017, 10, 2386–2394 CrossRef CAS.
- C. Fan, Y. Li, F. Y. Lu, H. X. Deng, Z. M. Wei and J. B. Li, RSC Adv., 2016, 6, 422–427 RSC.
- N. J. Huo and G. Konstantatos, Nat. Commun., 2017, 8, 572 CrossRef.
- Y. Huang, H. X. Deng, K. Xu, Z. X. Wang, Q. S. Wang, F. M. Wang, F. Wang, X. Y. Zhan, S. S. Li, J. W. Luo and J. He, Nanoscale, 2015, 7, 14093–14099 RSC.
- X. D. Wang, P. Wang, J. L. Wang, W. D. Hu, X. H. Zhou, N. Guo, H. Huang, S. Sun, H. Shen, T. Lin, M. H. Tang, L. Liao, A. Q. Jiang, J. L. Sun, X. J. Meng, X. S. Chen, W. Lu and J. H. Chu, Adv. Mater., 2015, 27, 6575–6581 CrossRef CAS PubMed.
- L. Li, X. S. Fang, T. Y. Zhai, M. Liao, U. K. Gautam, X. Wu, Y. Koide, Y. Bando and D. Golberg, Adv. Mater., 2010, 22, 4151–4156 CrossRef CAS PubMed.
- X. Xiong, Q. Zhang, X. Zhou, B. Jin, H. Q. Li and T. Y. Zhai, J. Mater. Chem. C, 2016, 4, 7817–7823 RSC.
Footnote |
† Electronic supplementary information (ESI) available. See DOI: 10.1039/c9na00471h |
|
This journal is © The Royal Society of Chemistry 2019 |
Click here to see how this site uses Cookies. View our privacy policy here.