DOI:
10.1039/C8NH00299A
(Communication)
Nanoscale Horiz., 2019,
4, 415-425
Photonic cancer nanomedicine using the near infrared-II biowindow enabled by biocompatible titanium nitride nanoplatforms†
Received
12th September 2018
, Accepted 10th October 2018
First published on 10th October 2018
Abstract
Light-activated photoacoustic imaging (PAI) and photothermal therapy (PTT) using the second near-infrared biowindow (NIR-II, 1000–1350 nm) hold great promise for efficient tumor detection and diagnostic imaging-guided photonic nanomedicine. In this work, we report on the construction of titanium nitride (TiN) nanoparticles, with a high photothermal-conversion efficiency and desirable biocompatibility, as an alternative theranostic agent for NIR-II laser-excited photoacoustic (PA) imaging-guided photothermal tumor hyperthermia. Working within the NIR-II biowindow provides a larger maximum permissible exposure (MPE) and desirable penetration depth of the light, which then allows detection of the tumor to the full extent using PA imaging and complete tumor ablation using photothermal ablation, especially in deeper regions. After further surface polyvinyl-pyrrolidone (PVP) modification, the TiN–PVP photothermal nanoagents exhibited a high photothermal conversion efficiency of 22.8% in the NIR-II biowindow, and we further verified their high penetration depth using the NIR-II biowindow and their corresponding therapeutic effect on the viability of tumor cells in vitro. Furthermore, these TiN–PVP nanoparticles were developed as a contrast agent for NIR-II-activated PA imaging both in vitro and in vivo for the first time and realized efficient photothermal ablation of the tumor in vivo within both the NIR-I and NIR-II biowindows. This work not only provides a paradigm for TiN–PVP photothermal nanoagents working in the NIR-II biowindow both in vitro and in vivo, but also proves the feasibility of PAI and PTT cancer theranostics using NIR-II laser excitation.
Conceptual insights
As one of the most promising cancer therapy modalities, nanoagent-enabled photothermal cancer ablation is highly promising because of its noninvasiveness, controllability and high efficiency. The ability to fabricate high-performance photo-responsive nanoagents with a desirable photothermal-conversion efficiency and photo-responsive wavelength range will determine the future therapeutic outcome, and is of high significance for potential clinical translation. This work has achieved intriguing near infrared-triggered photothermal cancer hyperthermia based on novel titanium nitride nanoplatforms using the NIR-II biowindow, which provides a larger maximum permissible exposure and desirable penetration depth of the light compared to the traditional NIR-I biowindow. Especially and importantly, contrast-enhanced photoacoustic cancer imaging in the NIR-II biowindow has also been realized using these as-designed titanium nitride nanoplatforms as the contrast agents. The demonstrated high biocompatibility and biosafety further guarantee the clinical-translation potential of these titanium nitride nanoplatforms as photo-responsive nanoagents for photonic cancer hyperthermia.
|
Introduction
The fast development of advanced nanotechnology and novel nanoplatforms has triggered and propelled numerous well-designed proposals and strategies for efficient cancer therapy.1–8 Compared to conventional treatments such as surgical removal, radiotherapy and chemotherapy, photothermal therapy (PTT), as one of the most promising and effective approaches for cancer ablation and hyperthermia, has attracted great attention in recent years.9–16 By employing light as an external and remote stimulus to generate heat, PTT features versatile advantages, such as easy localization, minimal invasiveness, tumor specificity, desirable controllability and a high therapeutic efficacy. To achieve efficient PTT, the photothermal-conversion nanoagents should be carefully designed and engineered, and the examples include well-constructed Au nanoparticles,17–19 transition-metal dichalcogenides,20–23 black phosphorous,24–26 MXenes,27,28 graphene and its derivatives,29–34 and even some organic nanoagents.35,36 Most studies have focused on designing and developing photothermal-conversion nanoagents with either a strong light absorption or high photothermal-conversion efficiency when using visible and short-wavelength near-infrared light (NIR-I, 750–1000 nm).10,37–40 However, the human tissue is a highly scattering medium for electromagnetic waves in the optical spectrum. When it comes to tumors in deeper sites, these photothermal nanoagents that work in the NIR-I biowindow don’t perform well because of heavy loss of laser energy on passing though the human body. To overcome this critical issue, utilization of light excitation within the second NIR (NIR-II) biowindow (1000–1350 nm) is preferable based on the potential for a satisfactory penetration depth of the tissue, with less light absorption, for efficient deep-located tumor therapies.
To optimize therapeutic efficiency and monitor therapeutic responses, bestowing the photothermal-conversion nanoagents with a concurrent diagnostic-imaging function and therapeutic ability is necessary.41–46 Fluorescence imaging provides safe and real-time detection along with the key advantage of its high spatial resolution.47–50 This benefit of high resolution unfortunately comes at the expense of limited tissue penetration. Photoacoustic imaging (PAI) based on optical excitation and ultrasonic detection is an emerging diagnostic-imaging modality.51–58 Specifically, after the nanoprobes absorb the excitation light, they generate acoustic pressure waves which can be detected by the external equipment and utilized for photoacoustic (PA) imaging. Compared to traditional optical-imaging technology that suffers from high scattering of the electromagnetic waves by the human tissues, PAI can break through the optical diffusion limit with much less scattering of the ultrasonic waves, providing high spatial resolution and real-time in vivo imaging without using any ionizing radiation. Great efforts have been made to explore new PA nanoprobes, such as carbon nanotubes,59 Au nanorods,60 methylene blue dye,61etc. However, similar to the PTT nanoagents, the main efforts have been devoted only to the design of NIR-I biowindow-excited PA contrast agents (CAs). There is a paucity of research on NIR-II-induced PA CAs, which result in blindness for deep tumor tissues or a certain degree of abnormal tissue morphology in the early stage of a tumor, impeding their further use in the clinic. Therefore, it is highly desirable to explore novel NIR-II biowindow-activated PA nanoagents for deep-site tumor detection and simultaneous achievement of efficient tumor-photothermal ablation.
Titanium nitride (TiN), an excellent conductive material, has been extensively used in many fields from solar thermoelectric generators to plasmon-mediated photocatalysis.62–64 It has been noted that both the titanium and nitrogen components in titanium nitride are highly biocompatible, therefore, it is expected to exert some specific functionalities in biomedical applications.65 In this work, to overcome the limited penetration depth of light and optimize the therapeutic efficiency, TiN nanoparticles (TiN NPs) with a simple composition and high photothermal-conversion efficiency were explored to serve as both PAI and PTT nanoagents using a NIR-II laser stimulus to activate these photothermal-conversion nanoagents. After further surface engineering using polyvinyl-pyrrolidone (PVP) molecules, the TiN–polyvinyl-pyrrolidone NPs (designated TiN–PVP NPs) exhibited high stability under physiological conditions, guaranteeing their further evaluation and application in vitro and in vivo. With a broad band absorption covering the entire UV-vis-NIR range from 300 to 1200 nm, the photothermal-conversion efficiency of the TiN–PVP NPs utilising the NIR-II (1064 nm) biowindow was calculated to be as high as 22.8%, which is sufficiently high for tumor photo-ablation. Furthermore, for comparison with the NIR-I biowindow, in vitro evaluation of the laser tissue-penetration depth using the NIR-II biowindow and investigation of the corresponding thermal effect on the viability of tumor cells have also been performed, demonstrating that working with the NIR-II biowindow can provide a larger maximum permissible exposure (MPE) and desirable tissue-penetration depth of the light. Especially, highly effective in vivo photoacoustic and photothermal therapies induced by the TiN–PVP NPs have been successfully demonstrated using the NIR-II biowindow.
Results and discussion
Synthesis and characterization of the TiN–PVP NPs
The extinction coefficient (ε) and photothermal-conversion efficiency (η) are the two main parameters to consider for desirable photothermal nanoagents. The extinction coefficient indicates the light-absorption performance of the agents and the corresponding photothermal-conversion efficiency displays their ability in converting the absorbed light into heat. TiN has shown a high optical absorption (about 95%) over a broad range and has exhibited plasmonic resonance in the biological-transparency window and high heating efficiencies in the NIR-I biowindow.63 Therefore, it is expected that TiN NPs would also be endowed with a high photothermal-conversion ability and potential for photothermal hyperthermia using the NIR-II biowindow where irradiation using a NIR-II (e.g., 1064 nm) laser would provide higher tissue penetration. To improve the stability of TiN NPs under physiological conditions, polyvinyl-pyrrolidone (PVP), as a broadly explored biocompatible organic molecule, was employed to engineer the surface of TiN NPs (Fig. 1a and b). The as-constructed TiN–PVP NPs with high biosafety, and a desirable extinction coefficient and photothermal-conversion efficiency, could be used to achieve NIR-II-activated PA imaging-guided PTT for in vivo photothermal tumor ablation (Fig. 1c and d).
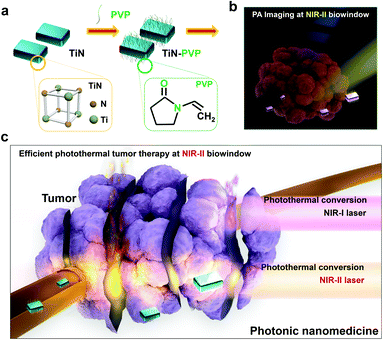 |
| Fig. 1 (a) The microstructure and surface modification of TiN using organic PVP molecules. (b) Schematic illustration of in vivo NIR-II-induced PA imaging employing TiN–PVP NPs as the CA. (c) Schematic illustration of the theranostic function of TiN–PVP NPs, i.e., NIR-II-excited PA imaging-guided efficient PT ablation of a tumor. | |
The SEM image of the obtained TiN NPs shows an irregular elliptical morphology with an average diameter of 25 nm (Fig. 2a). TEM images at different magnifications exhibit the small egg-like topology of these nanoagents (Fig. 2b and c). The high-resolution TEM (HRTEM) image and corresponding selected area electron diffraction (SAED) pattern clearly show the crystalline lattice and corresponding cubic TiN phase (Fig. 2d). The bright-field, dark-field and secondary electron SEM images (Fig. 2e–g) of the TiN NPs further evidence their small-sized nanostructure and slightly irregular topology. X-ray energy dispersive spectroscopy (EDS) of the TiN NPs confirmed the presence of Ti and N elements (Fig. 2h). The X-ray diffraction (XRD) pattern for the TiN NPs is shown in Fig. 2i. The characteristic diffraction peaks at 36.7, 42.5, 61.9, 74.1 and 78.04° are indexed to the (111), (200), (220), (311) and (222) crystal faces of TiN, respectively, further indicating the well-crystalline microstructure and the formation of a cubic TiN phase. X-ray photoelectron spectroscopy (XPS) was carried out to analyze the chemical state of the elements in the TiN NPs. The XPS results further indicate the presence of typical Ti and N signals (Fig. 2j). The high resolution XPS spectrum for Ti 2p in air-exposed TiN evidenced the presence of a thin oxidized overlayer on the TiN NPs. The peaks around 464.5 and 458.9 eV for Ti 2p were indexed to Ti–N and Ti–O bonds (Fig. 2k),65 respectively, which agrees with the co-existence of TiN and TiO2.
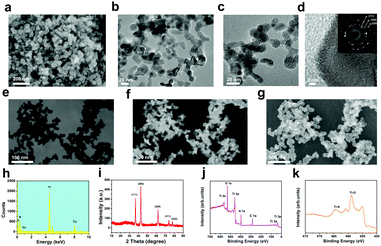 |
| Fig. 2 Structure, morphology and composition characterization of the TiN NPs. (a) SEM image of the TiN NPs. (b and c) TEM images of the TiN NPs at different magnifications. (d) High-resolution TEM (HRTEM) image of the TiN NPs. Inset shows the SAED pattern for the TiN NPs. (e) Dark-field, (f) bright-field, and (g) secondary electron SEM images of the TiN NPs. (h) X-ray energy dispersive spectroscopy (EDS) spectrum of the TiN NPs. (i) XRD pattern for the TiN NPs. (j) X-ray photoelectron spectroscopy (XPS) results for the TiN NPs. (k) High-resolution XPS spectrum for Ti 2p of the TiN NPs. | |
In addition, the TiN NPs showed high hydrophilicity and dispersity in water because of the presence of surface oxygenic TiO2 components, which resulted in the easy formation of hydrophilic groups on the surface. However, the TiN NPs easily aggregated in physiological solutions, which would possibly result in fast removal out of the body by the reticular phagocytic system (RES). To address this critical issue, typical biocompatible organic molecules, PVP, were utilized to engineer the surface of the TiN NPs, which bestowed these TiN NPs with excellent stability and high dispersity in various physiological environments, including in phosphate buffer saline (PBS), simulated body fluid (SBF) and Dulbecco's Modified Eagle's Medium (DMEM), which is mainly attributed to the steric hindrance of the organic chains (Fig. 3a). The hydrodynamic size of the TiN NPs, as determined by dynamic light scattering (DLS), was around 150 nm (Fig. S1, ESI†), indicating slight aggregation of these NPs. Fortunately, after surface modification with PVP, the hydrodynamic size of the TiN–PVP NPs was substantially reduced to about 70 nm because of the improved dispersity (Fig. 3b). The changes in zeta potential after PVP modification further confirmed successful grafting of the PVP molecules on the surface of the TiN NPs (Fig. S2, ESI†).
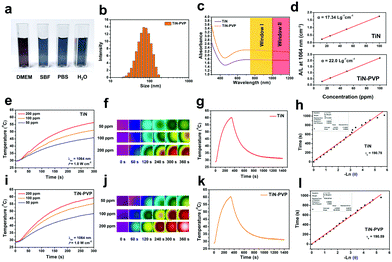 |
| Fig. 3 (a) Digital images of the TiN–PVP NPs dispersed in different physiological solutions. (b) DLS of the TiN–PVP NPs in aqueous solution. (c) UV-vis spectra of the TiN NPs and TiN–PVP NPs dispersed in aqueous solution at a concentration of 100 ppm. (d) Normalized absorbance intensity at λ = 1064 nm divided by the characteristic length of the cell (A/L) at elevated concentrations of the TiN and TiN–PVP NPs. (e and i) The photothermal-heating curves and (f and j) the corresponding temperature and thermal images of the TiN NPs and TiN–PVP NPs, respectively, at elevated concentrations (50, 100, 200 ppm) under 1064 nm laser irradiation at a power density of 1.0 W cm−2. (g and k) Photothermal effect of the aqueous dispersions of TiN and TiN–PVP NPs under 1064 nm laser irradiation (1.0 W cm−2). (h and l) Time constant plots for heat transfer with the TiN and TiN–PVP NPs. | |
Optical absorption and photothermal performance of the TiN–PVP NPs
Typically, desirable photothermal-conversion nanoagents for PTT have a strong absorption of NIR light and high photothermal-conversion efficiency. Based on this fact, we initially obtained the extinction coefficient (ε) of the TiN and TiN–PVP NPs for the NIR-II biowindow. It is shown (Fig. S3 (ESI†) and Fig. 3c) that both the TiN and TiN–PVP NPs have a broad band in the NIR region. The extinction coefficient of TiN at 1064 nm was calculated to be as high as 17.34 L g−1 cm−1 (Fig. 3d), which is much higher than that of traditional Au nanorods at 808 nm (13.9 L g−1 cm−1)66 or GO (3.6 L g−1 cm−1).30 After PVP modification, the extinction coefficient of TiN–PVP at 1064 nm reached an even higher value of 22.0 L g−1 cm−1, mainly attributed to the improved dispersity of the NPs in aqueous solution through PVP modification.
Then, the in vitro photothermal performance of the TiN and TiN–PVP NPs was assessed (Fig. 3e, f, i, j and Fig. S4, ESI†). The results exhibited the concentration/laser power-dependent photothermal features of the TiN–PVP NPs. The maximum temperature of the aqueous solution containing the TiN NPs reached 56 °C at a power density of 1.0 W cm−2 after 300 s of irradiation and reached about 59 °C after PVP modification under the same conditions, indicating PVP modification only had a slight influence on the photothermal performance of the TiN NPs. The photothermal stability of the TiN–PVP NPs under 1064 nm laser irradiation at a power density of 1.0 W cm−2 was further evaluated. No obvious changes were observed on elevating the temperature after 5 cycles (Fig. S4, ESI†). According to the results for the maximum temperature in the steady environment and the time constant for heat transfer during the cooling period, the photothermal-conversion efficiency (η) values of TiN and TiN–PVP under 1064 nm laser irradiation were calculated to be 22.7% and 22.8%, respectively, both of which are higher than those of representative Au nanorods (21%)67 and Cu2−xSe NCs (22%)68 for the NIR-I biowindow (Fig. 3g–i and k). These results demonstrate the strong NIR absorption and high photothermal-conversion efficiency (η) of the TiN–PVP NPs and that surface modification with PVP exhibited no significant effect on the photothermal-conversion efficiency (η).
The photothermal performance of the TiN–PVP NPs and the tissue-penetrating capability using the NIR-I and NIR-II biowindows were further assessed by determining the heating curve and the residual laser power density after penetrating the tissues under NIR-I and NIR-II laser irradiation, where chicken breast tissues with a varied thickness (0, 2.5 mm and 5 mm) were used as the model biological tissue (Fig. S5, ESI†). 808 nm and 980 nm laser irradiations were used as NIR-I laser sources and 1064 nm laser irradiation was used as a typical NIR-II laser source. An aqueous solution of TiN–PVP NPs (200 ppm) and chicken breast muscles of varying thicknesses (0, 2.5 mm and 5 mm) were analyzed, with different laser sources (808 nm, 980 nm and 1064 nm), using continuous laser irradiation (2.0 W cm−2, 5 min). The results showed that the maximum temperature of the TiN–PVP aqueous solution under 808 nm, 980 nm and 1064 nm laser irradiation reached rapidly up to nearly 76 °C, 76 °C and 74 °C, respectively (2.0 W cm−2, 5 min; Fig. 4a–c). When the penetration depth through the chicken breast tissues was set at 5 mm, the maximum temperature of the TiN–PVP aqueous solution under 808 nm, 980 nm and 1064 nm laser irradiation was 35.5 °C, 38.8 °C and 43.2 °C, respectively, under the same conditions, which is a notable decrease (Fig. 4d). The corresponding power density after penetration through the tissue of 5 mm thickness dropped from 2.0 W cm−2 to 0.20 W cm−2, 0.22 W cm−2 and 0.33 W cm−2 under 808 nm, 980 nm and 1064 nm laser irradiation, respectively (Fig. 4e), indicating that less attenuation of the photothermal heating occurred under NIR-II laser irradiation than under NIR-I laser irradiation after penetrating through the same thickness of chicken breast tissue. In addition, when the incident power density was decreased to 1.5 W cm−2, the corresponding residual power density after penetration through the tissue of 5 mm thicknesses dropped from 1.5 W cm−2 to 0.15 W cm−2, 0.14 W cm−2 and 0.19 W cm−2 under 808 nm, 980 nm and 1064 nm laser irradiation, respectively (Fig. S6, ESI†). Furthermore, we also evaluated the in vitro photothermal performance of the TiN–PVP NPs under 1064 nm laser irradiation at various concentrations with laser power densities of 1.5 and 2.0 W cm−1. Fig. S7 (ESI†) shows that the TiN–PVP NPs in aqueous solution exhibited concentration/laser power-dependent photothermal features.
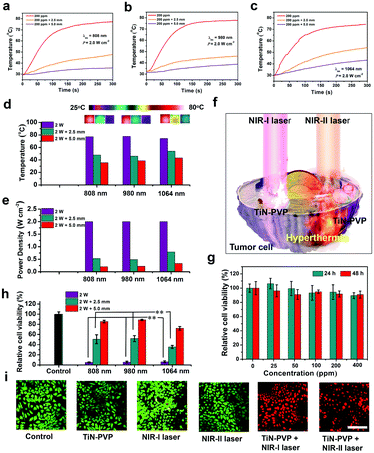 |
| Fig. 4 Photothermal heating curves for penetrating through chicken breast tissues of varied thickness (0, 2.5 mm and 5 mm) using (a) 808 nm, (b) 980 nm and (c) 1064 nm laser irradiation (2.0 W cm−2). (d) The highest temperature and (e) residual power density on penetrating through chicken breast tissues of varied thickness (0, 2.5 mm and 5 mm) using 808 nm, 980 nm and 1064 nm laser irradiation (2.0 W cm−2). (f) 3D schematic illustration of using TiN–PVP NPs for 4T1 cell ablation after exposure to NIR-I and NIR-II laser irradiation. (g) Relative viabilities of the 4T1 cells after incubation with increased concentrations of TiN–PVP NPs for 24 h and 48 h. (h) Relative viabilities of the 4T1 cells incubated with TiN–PVP NPs after penetrating through chicken breast tissues of varied thickness (0, 2.5 mm and 5 mm) using 808 nm, 980 nm and 1064 nm laser irradiation (2.0 W cm−2). Error bars are based on the standard deviation (s.d.) of five parallel samples (**P < 0.01). (i) CLSM images of 4T1 cancer cells stained using calcein AM and PI after different treatments. Images share the same scale bar of 50 μm. | |
In vitro photothermal cell ablation and endocytosis
The in vitro cell cytotoxicity of the TiN–PVP NPs as photothermal agents was tested using a standard CCK-8 assay. A 4T1 breast cancer cell line was used as the cell model. Initially, the 4T1 cells were incubated with the TiN–PVP NPs at different increasing concentrations (0, 25, 50, 100, 200 and 400 μg mL−1) for 24 and 48 h. These TiN–PVP NPs showed no obvious toxicity that affected the survival of the 4T1 cells (Fig. 4g). Then, the TiN–PVP NPs were assessed as photothermal agents for in vitro 4T1 cell ablation by exposure to 1064 nm laser irradiation. After 4 h of co-incubation of the 4T1 cells with TiN–PVP NPs (100 μg mL−1), the cancer cells were treated with 1064 nm laser irradiation at different power densities (0, 0.5, 1.0, 1.5 and 2.0 W cm−2) (Fig. S8a, ESI†). The results exhibited that enhanced 4T1 cell ablation was achieved with the 1064 nm laser and elevated power density. In addition, the photothermal ablation efficiency of the TiN–PVP NPs for 4T1 cell ablation at different concentrations from 0 to 180 μg mL−1 was also assessed upon 1064 nm laser irradiation (1.0 W cm−2) for 5 min (Fig. S8b, ESI†). It was shown that with increase of the concentration of the TiN–PVP NPs, the viability of the 4T1 cells sharply declined to 28.9% after 1064 nm laser irradiation.
Furthermore, in order to quantitatively assess the effect of the attenuation difference between NIR-I and NIR-II lasers on the viability of 4T1 tumor cells, we used the aforementioned model of chicken breast tissues of varying thicknesses (0, 2.5 mm and 5 mm). Fig. 4h shows that the viability of the 4T1 tumor cells under 808 nm, 980 nm and 1064 nm laser irradiation decreased to about 5.0%, 5.5% and 6.2%, respectively, in the presence of TiN–PVP NPs (2.0 W cm−2, 5 min). When covered with the tissue of 5 mm thickness, the inhibition rate for the 4T1 tumor cells treated with 1064 nm laser irradiation reached 27.9%, which is much higher than the inhibition rate for the 4T1 tumor cells treated with 808 nm (11.4%) or 980 nm (14.6%) laser irradiation, indicating that NIR-II laser irradiation resulted in stronger NIR tissue penetration than NIR-I laser irradiation, and could efficiently ablate more tumor cells which is beneficial for treating deep-seated tumor tissues.
Additionally, confocal fluorescence images of the 4T1 cancer cells were used to further confirm effective photothermal 4T1 cell death in the presence of TiN–PVP NPs after 808 nm and 1064 nm laser irradiation. The live and dead 4T1 cells were distinguished using calcein-AM and PI co-staining, respectively. After exposure to 808 nm and 1064 nm laser irradiation, it was clearly observed that a large amount of 4T1 cells, after incubation with the TiN–PVP NPs, were killed under both 808 nm and 1064 nm laser irradiation. In contrast, the viability of the 4T1 cells in the other groups displayed no obvious change.
PA-imaging performance of the TiN–PVP NPs using the NIR-II biowindow
The photoacoustic (PA) effect is the physical basis for PA imaging which refers to the generation of acoustic waves through the absorption of light (Fig. 5a). PA imaging which combines ultrasonic resolution with a high light contrast can break through the penetration limitation of traditional optical imaging based on its low tissue-attenuation coefficient.21,51,58 Unlike ionizing X-ray radiation, it can afford real-time detection of an in vivo biological structure and functional information in a non-invasive and non-ionizing manner. NIR-II laser-excited PA imaging can potentially provide deeper in vivo detection of biological tissue, which has seldom been reported so far. Owing to their high photothermal-conversion performance, the TiN–PVP NPs have shown a high potential for use as contrast-enhanced PA nanoagents. Therefore, we used 808 nm laser irradiation as the NIR-I laser source and 1280 nm laser irradiation as the NIR-II laser source to investigate. Fig. 5b and c show that different wavelengths of excitation light within the NIR-I biowindow and NIR-II biowindow were used to collect the PA signals of the TiN–PVP NPs. The excitation wavelengths in the NIR-I and NIR-II biowindows were optimized and fixed at 680 nm and 1270 nm, respectively. The PA images from a series of aqueous solutions of the TiN–PVP NPs (62.5, 125, 250 and 500 ppm) under 808 nm and 1280 nm light excitation were obtained and clearly exhibited that the PA signals of the TiN–PVP NPs have a linear relationship with the TiN concentration (Fig. 5d and e).
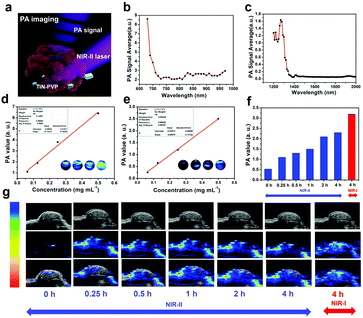 |
| Fig. 5 (a) 3D schematic illustration of in vivo PA imaging. (b) Different wavelengths (from 680 to 970 nm) of excitation light were used to collect the NIR-I PA signals of the TiN–PVP NPs. (c) Different wavelengths (from 1200 to 2000 nm) of excitation light were used to collect the NIR-II PA signals of the TiN–PVP NPs. (d) In vitro concentration-dependent PA signals of the TiN–PVP NPs under NIR-I laser irradiation. Inset: PA imaging of TiN–PVP NPs in buffer solution under NIR-I laser irradiation. (e) In vitro concentration-dependent PA signals of the TiN–PVP NPs under NIR-II laser irradiation. Inset: PA imaging of TiN–PVP NPs in buffer solution under NIR-II laser irradiation. (f) In vivo PA signal values and (g) NIR laser-exited PA imaging of 4T1 tumor-bearing mice after intravenous administration of TiN–PVP NPs over prolonged time intervals. | |
Based on the desirable in vitro results, we employed 4T1 tumor-bearing mice as an animal model to assess the in vivo PA-imaging performance of the TiN–PVP NPs using 1280 nm light excitation. After the tumor volume reached about 50 mm3, the mice were intravenously administered with TiN–PVP NPs (20 mg kg−1), and PA images were acquired at different time points after the injection (Fig. 5f and g). It was obviously exhibited that the intensity of the PA signal increased quickly from 0.51 (a.u.) to 1.1 (a.u.) 15 min after injection, under 1280 nm light excitation, mainly because of the efficient accumulation of nanosized TiN–PVP NPs in the tumor region via a typical EPR effect. The value reached nearly 2.3 (a.u.) 4 h after injection, indicating the high efficiency of TiN–PVP NPs as desirable contrast agents for PA imaging in the NIR-II biowindow. Furthermore, compared to the NIR-I-induced PA signals for tumor imaging, the PA imaging using the NIR-II biowindow showed a higher signal-to-noise ratio.
Efficient in vivo photothermal ablation of tumors using the TiN–PVP NPs
The biodistribution of TiN–PVP NPs in the main organs and tumor was investigated before the in vivo PTT experiments were performed (Fig. S9, ESI†). The results show that the intratumor passive accumulation efficiency of the TiN–PVP NPs was quantified as 1.51% and 0.47% ID g−1 after 6 h and 36 h of intravenous injection with 4T1 tumor-bearing mice, respectively. After that, motivated by the high in vitro PTT efficacy, we further investigated the feasibility of using the TiN–PVP NPs as photothermal agents for both the NIR-I and NIR-II windows for in vivo photothermal hyperthermia. A 4T1 breast tumor-bearing mouse xenograft was established as the animal model. After the tumor volumes reached nearly ∼80 mm3, the 4T1 tumor-bearing mice were randomly divided into six groups to receive different treatments (n = 5 per group). The groups were: (1) control group, (2) TiN–PVP group, (3) NIR-I laser group, (4) NIR-II laser group, (5) TiN–PVP + NIR-I laser group and (6) TiN–PVP + NIR-II laser group. 808 nm laser excitation was used as the NIR I laser source and 1064 nm laser excitation was employed as the NIR II laser source. Four hours after injection, the NIR-I laser group, NIR-II laser group, TiN–PVP + NIR-I laser group and TiN–PVP + NIR-II laser group were irradiated using a NIR-I or NIR-II laser (1.5 W cm−2, 10 min). Fig. 6a and b exhibit that after intravenous administration of the TiN–PVP NPs, the temperature of the tumors in group (5) increased to ∼55 °C within 10 min, whereas the temperature of the tumors in group (6), under 1064 nm laser irradiation at the same power density of 1.5 W cm−2, reached as high as 60 °C. In contrast, the tumor temperature for mice treated with only 808 nm or 1064 nm laser irradiation only increased slightly by 7–10 °C. After the various treatments, the tumor volumes and body weights of the six groups were recorded every two days (Fig. 6c and d) and digital photos of the tumor sites of each group were taken every four days (Fig. 6f and Fig. S10, ESI†). After the various treatments, the tumors in group (5) and (6) were eradicated without any recurrence and the mice survived over 50 days post treatment (Fig. 6e). In comparison, the tumor volumes of the other four groups increased rapidly and mice death occurred continuously after 20 days post treatment. The average lifespans of the mice in these four groups were remarkably shortened. All the mice showed no obvious weight fluctuation, confirming the negligible adverse effects of these received treatments on the health of mice.
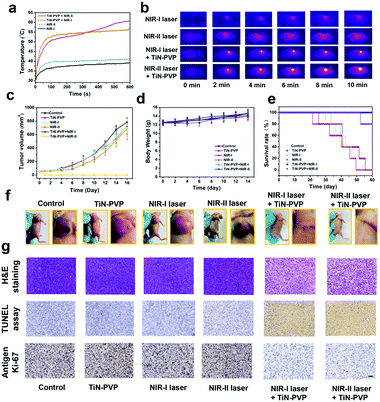 |
| Fig. 6 (a) Temperature curves for the tumor sites of 4T1 tumor-bearing nude mice under 808 nm and 1064 nm laser irradiation after intravenous injection of TiN–PVP nanoagents, and (b) corresponding IR thermal images of 4T1 tumor-bearing nude mice with or without intravenous injection of TiN–PVP nanoagents under 808 nm and 1064 nm laser irradiation (1.5 W cm−2) taken at different time intervals. (c) Time-dependent tumor-growth curves for the six groups after receiving different treatments. (d) Time-dependent body-weight curves for the 4T1 tumor-bearing nude mice from six experimental groups after receiving different treatments. (e) Survival rates of the 4T1 tumor-bearing mice within the feeding period after different treatments (n = 5). (f) Digital images of the tumors from each group at the end of the various treatments. (g) H&E staining, TUNEL staining and Antigen Ki-67 immunofluorescence staining of tumor tissues from each group after the different treatments. All the scale bars are 50 μm. | |
For further revealing the mechanism of the photothermal effect, hematoxylin–eosin (H&E) staining, TdT-mediated dUTP Nick-End Labeling (TUNEL) staining and Ki-67 antibody staining of tumor tissues from each group of mice 24 h after the various treatments were conducted. A high level of necrosis of the tumor cells was observed from micrographs of the H&E tumor slices from the TiN–PVP + NIR-I group and TiN–PVP + NIR-II group (Fig. 6g), which is also in accordance with the results from TUNEL staining. Comparatively, only insignificant abnormal features were observed in the other four groups. Ki-67 antibody staining was performed to assess the proliferative activities of the tumor cells. Significantly suppressed proliferative activities of the cancer cells from group (5) and group (6) were observed, while the other four groups presented no obvious suppression of tumor-cell proliferation. These results indicate that the TiN–PVP NPs with a high photothermal ablation effect hold high potential for ablating tumors when employing NIR-I or NIR-II laser irradiation for efficient tumor photo-hyperthermia.
In vivo biocompatibility assay of the TiN NPs
An in-depth investigation of the in vivo biocompatibility and biosafety of the TiN–PVP NPs was systematically conducted to guarantee their further potential for clinical application. Twenty-four healthy Kunming mice were randomly divided into four groups (n = 5). The groups were: (1) control group, (2) 5 mg kg−1 of TiN–PVP group, (3) 10 mg kg−1 of TiN–PVP group and (4) 20 mg kg−1 of TiN–PVP group. During the four weeks of testing and feeding, no obvious weight variation or abnormal behavior was observed in these four groups (Fig. S11, ESI†). Afterwards, these mice were sacrificed. The major organs from the various groups of mice were collected for H&E staining and pathological characterization (Fig. 7). No obvious shrunken malignant cells, loss of contact or nucleus damage were observed in the examined tissue sections from all four groups, indicating that no significant chronic pathological toxicity or adverse effects were found after injection of the TiN–PVP NPs during the one-month feeding period. A series of blood indexes for all the groups were measured at one month post injection. All the indexes in the three TiN–PVP-treated groups exhibited no obvious abnormality compared to those of the control group without any treatment and the changes among these four groups were of no statistical significance (Fig. 8). As evidenced by the desirable in vivo biosafety results, these TiN–PVP NPs show high promise for further bio-application and possibly further clinical translation.
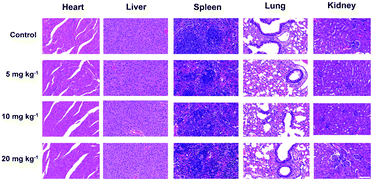 |
| Fig. 7 H&E-stained tissue sections from the major organs of mice after intravenous injection with different doses of TiN–PVP and after one-month of feeding. All the scale bars are 100 μm. | |
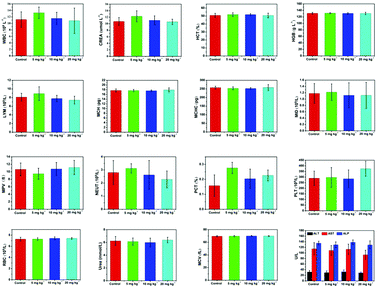 |
| Fig. 8 The related indexes for mice from each group with different TiN–PVP doses of 0 mg kg−1, 5 mg kg−1, 10 mg kg−1 and 20 mg kg−1 after intravenous administration and a further one-month of feeding. | |
Experimental section
Synthesis of the TiN–PVP NPs
The TiN nanoparticles (NPs) were obtained from Beijing Dk Nano Technology Co. Firstly, the pristine TiN samples were dispersed in deionized water for 12 h of sonication to obtain ultrasmall TiN NPs. To further improve the dispersity of the TiN NPs under physiological conditions, the TiN NPs (0.4 g) were dissolved in 60 mL of aqueous solution containing PVP (0.8 g, polyvinylpyrrolidone, average MW 40 000) with gentle stirring at 60 °C for 24 h. The as-prepared TiN–PVP NPs were collected by centrifugation and washed with deionized water and ethanol several times to remove residual PVP molecules.
Characterization
Transmission electron microscopy (TEM) images and the corresponding X-ray energy dispersive X-ray spectroscopy (EDS) spectrum were acquired using a JEM-2100F electron microscope operated at 200 kV. Scanning electron microscopy (SEM) images were obtained using a field emission Magellan 400 microscope (FEI Company). X-ray diffraction (XRD) was performed on a Rigaku D/MAX-2200 PC XRD system (parameters were as follows: Cu Kα, λ = 1.54 Å, 40 mA, and 40 kV). The X-ray photoelectron spectroscopy (XPS) spectrum was obtained with ESCAlab250 (Thermal Scientific). UV-vis-NIR absorption spectra were recorded using a UV-3600 Shimadzu UV-vis-NIR spectrometer at room temperature. Size and zeta potential measurements were measured using a Zetasizer Nanoseries (Nano ZS90, Malvern Instrument Ltd). The quantitative analysis of Ti element concentration was performed using inductively coupled plasma-optical emission spectrometry (ICP-OES, Agilent 725, Agilent Technologies, USA). The confocal laser scanning microscopy (CLSM) images were collected using a FV1000 (Olympus Company, Japan). The thermal images were acquired using an infrared thermal imaging instrument (FLIR A325SC camera). The NIR-I laser irradiation was performed by utilizing an 808 nm or 980 nm laser pump (Shanghai Connect Fiber Optics Co.). The NIR-II laser irradiation was carried out by utilizing a 1064 nm laser pump (Shanghai Connect Fiber Optics Co.).
In vitro photothermal performance of the TiN–PVP NPs
To investigate the in vitro photothermal performance of the TiN–PVP NPs, aqueous solutions (0.1 mL) of TiN–PVP with different TiN concentrations (0, 22.5, 45, 90 and 180 ppm) were measured and analyzed by continuously irradiating with an 1064 nm NIR laser (Shanghai Connect Fiber Optics Company) at a power density of 1.0 W cm−2 for 5 min, respectively. The temperature after different time durations was recorded using a FLIR™ A325SC camera. The detailed calculation for the photothermal-conversion efficiency is provided in the ESI.†
Deep-tissue photothermal performance of the TiN–PVP NPs for both the NIR-I and NIR-II biowindows
Using the NIR-II biowindow (1000–1350 nm) for phototherapy can achieve an improved penetration depth and larger maximum permissible exposure (MPR), attributed to lower absorption and scattering when passing through biological tissues. Thus, we evaluated the tissue penetration performance using NIR-I and NIR-II laser irradiation by measuring the heat curve and the residual laser energy density after penetrating chicken breast muscles of varied thickness (0, 2.5 mm and 5 mm), which were used as model biological tissues. 808 nm and 980 nm laser irradiations were used to represent a NIR-I light source and 1064 nm laser irradiation represented a NIR-II light source. The deep tissue photothermal performance was evaluated using TiN–PVP NPs with the above chicken breast tissues of different thicknesses of 0, 2.5 mm and 5.0 mm under 808 nm, 980 nm and 1064 nm laser irradiation. 100 μL aliquots of a TiN–PVP NPs aqueous solution in 96 wells covered with chicken breast tissues of different thicknesses were treated with 808 nm, 980 nm and 1064 nm laser irradiation at a power density of 2.0 W cm−2 for 5 min, and the infrared thermographs were obtained using a thermal imaging camera.
In vitro cytotoxicity assay
A murine breast cancer 4T1 cell line (4T1 cells; Cell Bank of Shanghai Institutes for Biological Sciences, Chinese Academy of Sciences) was used as the model cell type to assess the in vitro cytotoxicity of the TiN–PVP NPs. The 4T1 cells were maintained at 37 °C under 5% CO2 in Dulbecco's modified Eagle's medium (DMEM, high glucose, GIBCO, Invitrogen) and supplemented with 1% penicillin/streptomycin and 10% fetal bovine serum (FBS) in a humidified incubator. Firstly, the cells (1 × 105 cells per well) were generally plated in 96-well plates for 24 h to allow the cells to adhere, and then the culture medium was replaced with fresh culture medium containing TiN–PVP at different increasing concentrations (0, 25, 50, 100, 200 and 400 μg mL−1). After 24 and 48 h of incubation, the CCK-8 assay was performed to access the viability of the cancer cells (n = 5).
In vitro photothermal ablation of cancer cells
4T1 cells (1 × 105 cells per well) were initially cultivated in 96-well plates in DMEM at 37 °C in the presence of 5% CO2 over 24 h. Afterwards, the culture medium was discarded and then fresh medium containing TiN–PVP NPs at a TiN concentration of 100 μg mL−1 was dispersed into the wells. After 4 h of co-incubation, the above culture medium was discarded and the cells were rinsed several times with PBS (Runcheng Biotech Co., Ltd, Shanghai). After that, the cells were placed under 1064 nm laser irradiation at different power densities (0, 0.5, 1.0, 1.5 and 2.0 W cm−2) for 5 min. Finally, a standard CCK-8 assay was performed to assess the viability of the cells (n = 5).
In vitro deep-tissue photothermal ablation of cancer cells using both NIR-I and NIR-II biowindows
4T1 cells (1 × 105 cells per well) were initially cultivated in 96-well plates in DMEM at 37 °C in the presence of 5% CO2 over 24 h. Then, the culture medium was replaced with fresh medium containing TiN–PVP NPs at a TiN concentration of 200 μg mL−1. After 4 h of incubation, the in vitro viability of the cells was investigated under NIR-I and NIR-II laser irradiation, where 808 nm and 980 nm laser pumps were used as a NIR-I light source and a 1064 nm laser pump was used as the NIR-II light source. Chicken breast tissues of different thicknesses of 0 mm, 2.5 mm and 5 mm were used as model biological tissues.
In vitro PTT effect as observed using CLSM
4T1 cancer cells were initially seeded in CLSM-specific culture dishes for 12 h to adhere overnight at 37 °C in humidified 5% CO2. Then, the medium was discarded and replaced with fresh medium containing the TiN–PVP NPs (200 μg mL−1). After 4 h of incubation, the cells were then exposed to different treatments, including a control group, TiN–PVP only, NIR-I laser only, NIR-II laser only, TiN–PVP + NIR-I laser, and TiN–PVP + NIR-II laser. After that, the cell culture medium was removed and replaced by calcein-AM (100 μL) and PI solution (100 μL, Dojindo Molecular Technologies, Inc.) for another 20 min. Finally, the medium was removed and the cells were rinsed with PBS twice and observed using confocal laser scanning microscopy (CLSM, FV 1000, Olympus), where dead cells were stained in red while live cells were stained in green.
In vitro and in vivo photoacoustic imaging
For in vitro PA imaging, the PA signals for the NIR-I and NIR-II biowindows were obtained using different wavelengths of excitation light from 680 to 970 nm and 1200 to 2000 nm, respectively. Then, TiN–PVP NPs were dispersed into deionized water at different increasing concentrations (0.0625, 0.125, 0.25, and 0.5 mg mL−1) and were utilized for PA signal detection to assess the linearity of the PA signals as a function of TiN–PVP NP concentration. 808 nm laser irradiation was used as the NIR-I excitation light source and 1280 nm laser irradiation was used as the NIR-II excitation light source. Imaging parameters: the region of interest was 20 mm; PA gain, 25 dB; 2D gain, 13 dB; wavelength, 808 nm for NIR-I biowindow, 1280 nm for NIR-II biowindow.
For in vivo PA imaging, 4T1 tumor-bearing mice were intravenously administered with TiN–PVP NPs (20 mg kg−1) after the tumor size reached nearly 50 mm3. PA imaging of the 4T1 tumor-bearing mice using both the NIR-I and NIR-II biowindows was performed after intravenous injection of the TiN–PVP NPs. The PA signal before injection was used as the control. The in vitro and in vivo PA imaging experiments were performed using a Fujifilm VisualSonics/VEVO LAZR-X PA Imaging System. Imaging parameters: the region of interest was 20 mm; PA gain, 46 dB; 2D gain, 16 dB; wavelength, 808 nm for NIR-I biowindow, 1280 nm for NIR-II biowindow.
In vivo toxicity assay
The animal experiment procedures were in agreement with the guidelines of the Regional Ethics Committee for Animal Experiments and the care regulations approved by the Administrative Committee of Laboratory Animals of Fudan University. Healthy female Kunming mice (22 g) were obtained and raised at the Laboratory Animal Center, Shanghai Medical College of Fudan University. Twenty mice (4 weeks old) were divided into four groups, followed by the intravenous administration of TiN–PVP NPs at different doses. The groups were: (1) control group (only intravenously injected with saline), (2) 5 mg kg−1 of TiN–PVP group, (3) 10 mg kg−1 of TiN–PVP group and (4) 20 mg kg−1 of TiN–PVP group. The body weight of the mice was recorded every two days. After intravenous administration of the TiN–PVP NPs, on the 30th day, the mice were scarified and blood samples were then collected to conduct a serum biochemistry assay and complete blood panel test. The blood indexes were measured. The major organs of the mice were collected, fixed in 10% formalin, embedded into paraffin, and stained with hematoxylin and eosin (H&E) for histological analysis.
Biodistribution assay of the TiN–PVP NPs
The biodistribution of the TiN–PVP NPs in main organs and tumors was assessed using 4T1-tumor-bearing mice (n = 3). The 4T1-tumor-bearing mice were intravenously administered with TiN–PVP NPs at a dose of 20 mg kg−1 (0.1 mL). Mice were dissected after 6 h and 36 h after treatment. The dissected organs and tumors were weighed and dissolved with strong acid. The TiN–PVP NP distributions in different tissues were calculated as the percentage of injected dose per gram of tissue.
In vivo photothermal therapy using both the NIR-I and NIR-II biowindows
Thirty 4 week old female Balb/c nude mice were obtained for in vivo therapeutic evaluation. These nude mice were injected subcutaneously with 4T1 cells (1 × 106 cells per site) suspended in PBS (100 μL) to develop the tumor model. When the tumor volume reached about 80 mm3, these mice were randomly divided into six groups. The groups were: (1) control group, (2) TiN–PVP group (only intravenously injected with TiN–PVP NPs), (3) NIR-I group (only exposed to NIR-I laser irradiation), (4) NIR-II group (only exposed to NIR-II laser irradiation), (5) TiN–PVP + NIR-I laser group (intravenously injected with TiN–PVP NPs and exposed to NIR-I laser irradiation), (6) TiN–PVP + NIR-II laser group (intravenously injected with TiN–PVP NPs and exposed to NIR-II laser irradiation). 808 nm laser irradiation was used as the NIR-I light source and 1064 nm laser irradiation was used as the NIR-II light source. 4 h after administration of the TiN–PVP NPs, all the mice were anesthetized and in vivo photothermal ablation was performed. The tumor volumes of the mice were recorded every 2 days according to the following formula: tumor volume = (tumor length) × (tumor width)2/2 and corresponding photographs were taken every 4 days. Afterwards, the tumors were collected from each group and fixed in 10% formalin, followed by H&E, TUNEL, and Ki-67 staining for histological analysis.
Statistical analysis
The data are presented as mean ± s.d., and the significance of the data in this work was analyzed according to a Kruskal–Wallis test (**P < 0.01).
Conclusions
In summary, titanium nitride (TiN) NPs with a high photothermal-conversion efficiency and desirable biocompatibility have been successfully developed, for the first time, as an alternative but highly efficient theranostic agent for NIR-II laser-excited PA imaging-guided photothermal hyperthermia of tumors. These TiN–PVP NPs exhibited a broadband absorption covering the entire UV-vis-NIR range from 300 to 1200 nm. After surface PVP functionalization, the TiN–PVP NPs featured high dispersity and excellent biocompatibility under physiological conditions. Especially and importantly, the photothermal-conversion efficiency for the NIR-II (1064 nm) biowindow was calculated to be 22.8%, which is sufficiently high for photo-ablation of tumors. Additionally, the advantages of working with the NIR-II biowindow of a larger maximum permissible exposure (MPE) and desirable penetration depth of the light were evidenced. Especially, the capability of the TiN NPs as a contrast agent for PA imaging using the NIR-II biowindow has been demonstrated both in vitro and in vivo, and their high photothermal-conversion performance using the NIR-II biowindow successfully eradicated tumors without reoccurrence from a 4T1 breast cancer xenograft. Therefore, this work not only reveals the high potential of the NIR-II biowindow for photonic cancer nanomedicine, but also provides a novel theranostic nanoplatform (TiN in this work) for efficient cancer phototherapies using the NIR-II biowindow.
Conflicts of interest
The authors declare no conflicts of interest.
Acknowledgements
We greatly acknowledge financial support through grants from the National Key R&D Program of China (Grant No. 2016YFA0203700), National Natural Science Foundation of China (Grant No. 81670067, 51672303 and 81501444), Excellent Young Scientist Foundation of NSFC (Grant No. 51722211), Program of Shanghai Subject Chief Scientist (Grant No. 18XD1404300), Shanghai Science and Technology Commission Medical Guidance Project of China (15411962300) and Shanghai Key Medical Discipline for Critical Care Medicine (2017ZZ02012-2).
Notes and references
- J. A. Barreto, W. O'Malley, M. Kubeil, B. Graham, H. Stephan and L. Spiccia, Adv. Mater., 2011, 23, H18–H40 CrossRef CAS PubMed.
- M. Ferrari, Nat. Rev. Cancer, 2005, 5, 161–171 CrossRef CAS PubMed.
- S. Nazir, T. Hussain, A. Ayub, U. Rashid and A. J. MacRobert, Nanomed. Nanotechnol., 2014, 10, 19–34 CrossRef CAS PubMed.
- R. Misra, S. Acharya and S. K. Sahoo, Drug Discovery Today, 2010, 15, 842–850 CrossRef CAS PubMed.
- X. Ai, C. J. Ho, J. Aw, A. B. Attia, J. Mu, Y. Wang, X. Wang, Y. Wang, X. Liu, H. Chen, M. Gao, X. Chen, E. K. Yeow, G. Liu, M. Olivo and B. Xing, Nat. Commun., 2016, 7, 10432 CrossRef CAS PubMed.
- Z. Zhou, J. Song, L. Nie and X. Chen, Chem. Soc. Rev., 2016, 45, 6597–6626 RSC.
- W. Zhou, X. Gao, D. Liu and X. Chen, Chem. Rev., 2015, 115, 10575–10636 CrossRef CAS PubMed.
- Q. Chen, L. Feng, J. Liu, W. Zhu, Z. Dong, Y. Wu and Z. Liu, Adv. Mater., 2016, 28, 7129–7136 CrossRef CAS PubMed.
- M. Chen, S. Tang, Z. Guo, X. Wang, S. Mo, X. Huang, G. Liu and N. Zheng, Adv. Mater., 2014, 26, 8210–8216 CrossRef CAS PubMed.
- H. Lin, X. Wang, L. Yu, Y. Chen and J. Shi, Nano Lett., 2017, 17, 384–391 CrossRef CAS PubMed.
- Z. Zhang, J. Wang and C. Chen, Adv. Mater., 2013, 25, 3869–3880 CrossRef CAS PubMed.
- Q. Chen, L. Xu, C. Liang, C. Wang, R. Peng and Z. Liu, Nat. Commun., 2016, 7, 13193 CrossRef CAS PubMed.
- Q. Chen, C. Liang, C. Wang and Z. Liu, Adv. Mater., 2015, 27, 903–910 CrossRef CAS PubMed.
- Y. Wang, Y. Deng, H. Luo, A. Zhu, H. Ke, H. Yang and H. Chen, ACS Nano, 2017, 11, 12134–12144 CrossRef CAS PubMed.
- H. Luo, Q. Wang, Y. Deng, T. Yang, H. Ke, H. Yang, H. He, Z. Guo, D. Yu, H. Wu and H. Chen, Adv. Funct. Mater., 2017, 27, 1702834 CrossRef.
- S. Ye, J. Rao, S. Qiu, J. Zhao, H. He, Z. Yan, T. Yang, Y. Deng, H. Ke, H. Yang, Y. Zhao, Z. Guo and H. Chen, Adv. Mater., 2018, e1801216 CrossRef PubMed.
- H. Liu, T. Liu, X. Wu, L. Li, L. Tan, D. Chen and F. Tang, Adv. Mater., 2012, 24, 755–761 CrossRef CAS PubMed.
- J. Kim, S. Park, J. E. Lee, S. M. Jin, J. H. Lee, I. S. Lee, I. Yang, J.-S. Kim, S. K. Kim, M.-H. Cho and T. Hyeon, Angew. Chem., Int. Ed., 2006, 118, 7918–7922 CrossRef.
- M. S. Yavuz, Y. Cheng, J. Chen, C. M. Cobley, Q. Zhang, M. Rycenga, J. Xie, C. Kim, K. H. Song, A. G. Schwartz, L. V. Wang and Y. Xia, Nat. Mater., 2009, 8, 935–939 CrossRef CAS PubMed.
- L. Cheng, J. Liu, X. Gu, H. Gong, X. Shi, T. Liu, C. Wang, X. Wang, G. Liu, H. Xing, W. Bu, B. Sun and Z. Liu, Adv. Mater., 2014, 26, 1886–1893 CrossRef CAS PubMed.
- X. Qian, S. Shen, T. Liu, L. Cheng and Z. Liu, Nanoscale, 2015, 7, 6380–6387 RSC.
- S. S. Chou, B. Kaehr, J. Kim, B. M. Foley, M. De, P. E. Hopkins, J. Huang, C. J. Brinker and V. P. Dravid, Angew. Chem., Int. Ed., 2013, 52, 4160–4164 CrossRef CAS PubMed.
- Y. Yong, L. Zhou, Z. Gu, L. Yan, G. Tian, X. Zheng, X. Liu, X. Zhang, J. Shi, W. Cong, W. Yin and Y. Zhao, Nanoscale, 2014, 6, 10394–10403 RSC.
- C. Sun, L. Wen, J. Zeng, Y. Wang, Q. Sun, L. Deng, C. Zhao and Z. Li, Biomaterials, 2016, 91, 81–89 CrossRef CAS PubMed.
- G. Yang, Z. Liu, Y. Li, Y. Hou, X. Fei, C. Su, S. Wang, Z. Zhuang and Z. Guo, Biomaterials, 2017, 5, 2048–2055 RSC.
- Y. Li, Z. Liu, Y. Hou, G. Yang, X. Fei, H. Zhao, Y. Guo, C. Su, Z. Wang, H. Zhong, Z. Zhuang and Z. Guo, ACS Appl. Mater. Interfaces, 2017, 9, 25098–25106 CrossRef CAS PubMed.
- H. Lin, Y. Chen and J. Shi, Adv. Sci., 2018, 1800518 CrossRef PubMed.
- H. Lin, Y. Wang, S. Gao, Y. Chen and J. Shi, Adv. Mater., 2018, 30, 1703284 CrossRef PubMed.
- K. Yang, S. Zhang, G. Zhang, X. Sun, S. T. Lee and Z. Liu, Nano Lett., 2010, 10, 3318–3323 CrossRef CAS PubMed.
- J. T. Robinson, S. M. Tabakman, Y. Liang, H. Wang, H. S. Casalongue, D. Vinh and H. Dai, J. Am. Chem. Soc., 2011, 133, 6825–6831 CrossRef CAS PubMed.
- X. Wang, C. Wang, L. Cheng, S. T. Lee and Z. Liu, J. Am. Chem. Soc., 2012, 134, 7414–7422 CrossRef CAS PubMed.
- Y. Yong, L. Zhou, S. Zhang, L. Yan, Z. Gu, G. Zhang and Y. Zhao, NPG Asia Mater., 2016, 8, e273 CrossRef CAS.
- K. Yang, J. Wan, S. Zhang, B. Tian, Y. Zhang and Z. Liu, Biomaterials, 2012, 33, 2206–2214 CrossRef CAS PubMed.
- K. Yang, L. Hu, X. Ma, S. Ye, L. Cheng, X. Shi, C. Li, Y. Li and Z. Liu, Adv. Mater., 2012, 24, 1868–1872 CrossRef CAS PubMed.
- J. Li, C. Xie, J. Huang, Y. Jiang, Q. Miao and K. Pu, Angew. Chem., Int. Ed., 2018, 57, 3995–3998 CrossRef CAS PubMed.
- X. Zhen, C. Xie and K. Pu, Angew. Chem., Int. Ed., 2018, 57, 3938–3942 CrossRef CAS PubMed.
- H. Lin, S. Gao, C. Dai, Y. Chen and J. Shi, J. Am. Chem. Soc., 2017, 139, 16235–16247 CrossRef CAS PubMed.
- X. Zhu, W. Feng, J. Chang, Y. W. Tan, J. Li, M. Chen, Y. Sun and F. Li, Nat. Commun., 2016, 7, 10437 CrossRef CAS PubMed.
- J. Conde, N. Oliva, Y. Zhang and N. Artzi, Nat. Mater., 2016, 15, 1128–1138 CrossRef CAS PubMed.
- L. Lin, L. Liu, B. Zhao, R. Xie, W. Lin, H. Li, Y. Li, M. Shi, Y. G. Chen, T. A. Springer and X. Chen, Nat. Nanotechnol., 2015, 10, 465–471 CrossRef CAS PubMed.
- Z. Liu, F. Pu, S. Huang, Q. Yuan, J. Ren and X. Qu, Biomaterials, 2013, 34, 1712–1721 CrossRef CAS PubMed.
- X. Huang, G. Deng, L. Liao, W. Zhang, G. Guan, F. Zhou, Z. Xiao, R. Zou, Q. Wang and J. Hu, Nanoscale, 2017, 9, 2626–2632 RSC.
- Z. Li, J. Liu, Y. Hu, K. A. Howard, Z. Li, X. Fan, M. Chang, Y. Sun, F. Besenbacher, C. Chen and M. Yu, ACS Nano, 2016, 10, 9646–9658 CrossRef CAS PubMed.
- W. Zhang and C. Gao, Sci. Bull., 2015, 60, 1973–1979 CrossRef CAS.
- C. Dai, S. Zhang, Z. Liu, R. Wu and Y. Chen, ACS Nano, 2017, 11, 9467–9480 CrossRef CAS PubMed.
- C. Dai, Y. Chen, X. Jing, L. Xiang, D. Yang, H. Lin, Z. Liu, X. Han and R. Wu, ACS Nano, 2017, 11, 12696–12712 CrossRef CAS PubMed.
- H. S. Choi, S. L. Gibbs, J. H. Lee, S. H. Kim, Y. Ashitate, F. Liu, H. Hyun, G. Park, Y. Xie, S. Bae, M. Henary and J. V. Frangioni, Nat. Biotechnol., 2013, 31, 148–153 CrossRef CAS PubMed.
- S. Gioux, H. S. Choi and J. V. Frangioni, Mol. Imaging, 2010, 9, 237–255 CrossRef CAS PubMed.
- G. Hong, J. C. Lee, J. T. Robinson, U. Raaz, L. Xie, N. F. Huang, J. P. Cooke and H. Dai, Nat. Med., 2012, 18, 1841–1846 CrossRef CAS PubMed.
- P. Wang, Y. Fan, L. Lu, L. Liu, L. Fan, M. Zhao, Y. Xie, C. Xu and F. Zhang, Nat. Commun., 2018, 9, 2898 CrossRef PubMed.
- M. Xu and L. V. Wang, Rev. Sci. Instrum., 2006, 77, 041101 CrossRef.
- X. Wang, X. Xie, G. Ku, L. V. Wang and G. Stoica, J. Biomed. Opt., 2006, 11, 024015 CrossRef PubMed.
- Y. J. Liu, L. M. Nie and X. Y. Chen, Trends Biotechnol., 2016, 34, 420–433 CrossRef CAS PubMed.
- P. Beard, Interface Focus, 2011, 1, 602–631 CrossRef PubMed.
- S. Wang, J. Lin, T. F. Wang, X. Y. Chen and P. Huang, Theranostics, 2016, 6, 2394–2413 CrossRef CAS PubMed.
- K. Cheng, H. Chen, C. H. Jenkins, G. L. Zhang, W. Zhao, Z. Zhang, F. Han, J. Fung, M. Yang, Y. X. Jiang, L. Xing and Z. Cheng, ACS Nano, 2017, 11, 12276–12291 CrossRef CAS PubMed.
- L. Y. Cui and J. H. Rao, Wires. Nanomed. Nanobi., 2017, 9, e1418 CrossRef PubMed.
- L. V. Wang and S. Hu, Science, 2012, 335, 1458–1462 CrossRef CAS PubMed.
- A. De La Zerda, C. Zavaleta, S. Keren, S. Vaithilingam, S. Bodapati, Z. Liu, J. Levi, B. R. Smith, T. J. Ma, O. Oralkan, Z. Cheng, X. Y. Chen, H. J. Dai, B. T. Khuri-Yakub and S. S. Gambhir, Nat. Nanotechnol., 2008, 3, 557–562 CrossRef CAS PubMed.
- A. Agarwal, S. W. Huang, M. O'Donnell, K. C. Day, M. Day, N. Kotov and S. Ashkenazi, J. Appl. Phys., 2007, 102, 064701 CrossRef.
- T. N. Erpelding, C. Kim, M. Pramanik, L. Jankovic, K. Maslov, Z. Guo, J. A. Margenthaler, M. D. Pashley and L. V. Wang, Radiology, 2010, 256, 102–110 CrossRef PubMed.
- G. R. Li, F. Wang, Q. W. Jiang, X. P. Gao and P. W. Shen, Angew. Chem., Int. Ed., 2010, 49, 3653–3656 CrossRef CAS PubMed.
- D. R. Clements, Y. Kim, S. A. Gujar and P. W. K. Lee, OncoImmunology, 2016, 5(1), e1057674 CrossRef PubMed.
- Y. Yue, P. Han, X. He, K. Zhang, Z. Liu, C. Zhang, S. Dong, L. Gu and G. Cui, J. Mater. Chem., 2012, 22, 4938 RSC.
- W. He, K. Ai, C. Jiang, Y. Li, X. Song and L. Lu, Biomaterials, 2017, 132, 37–47 CrossRef CAS PubMed.
- J. T. Robinson, K. Welsher, S. M. Tabakman, S. P. Sherlock, H. Wang, R. Luong and H. Dai, Nano Res., 2010, 3, 779–793 CrossRef CAS PubMed.
- J. Zeng, D. Goldfeld and Y. Xia, Angew. Chem., Int. Ed., 2013, 52, 4169–4173 CrossRef CAS PubMed.
- C. M. Hessel, V. P. Pattani, M. Rasch, M. G. Panthani, B. Koo, J. W. Tunnell and B. A. Korgel, Nano Lett., 2011, 11, 2560–2566 CrossRef CAS PubMed.
Footnotes |
† Electronic supplementary information (ESI) available. See DOI: 10.1039/c8nh00299a |
‡ These authors contributed equally to this work. |
|
This journal is © The Royal Society of Chemistry 2019 |
Click here to see how this site uses Cookies. View our privacy policy here.