DOI:
10.1039/C8NH00373D
(Minireview)
Nanoscale Horiz., 2019,
4, 579-591
Are lanthanide-doped upconversion materials good candidates for photocatalysis?
Received
21st October 2018
, Accepted 26th November 2018
First published on 26th November 2018
Abstract
The inability to harvest the broad spectrum of solar irradiation has constrained the practical applications of photocatalysis in the environmental and energy sectors. Although the importance of largely improving visible photon utilization is well recognized, the opinion on photon capture in the near-infrared (NIR) region of the solar spectrum is somewhat controversial due to the low energy of NIR photons and relatively more photon absorption by water in this range with respect to the visible regime. In addition, the viability of enhancing the utilization of NIR light for photocatalysis via lanthanide-doped upconversion (UC) particles is also under debate because of their low UC quantum yield and preference for lasers with specific, limited wavelengths as excitation sources, etc. Facing these challenges, several groups have made efforts toward the investigation of NIR-light photocatalysis enabled by UC particles. The achieved great advances in enhancing the light absorption of UC particles by coupling them with plasmonic metals render this emerging area, UC-enhanced photocatalysis, quite promising. Herein, we review the most recent advances of UC particles from the perspective of synthetic methods, luminescence enhancement strategies and their applications in photocatalysis. In particular, we intend to draw attention to the recent progress in designing efficient and broadband photocatalysts by integrating traditional semiconductor photocatalysts and/or recently emerging plasmon-semiconductor photocatalysts with lanthanide-doped UC materials. We would like to highlight that the involvement of plasmonic nanomaterials opens up a new avenue for the use of UC nanomaterials in photocatalysis. The current challenges in and perspectives toward this dynamic field are also provided.
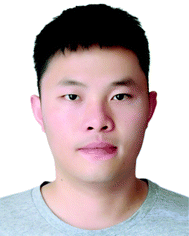
Qingzhe Zhang
| Qingzhe Zhang is currently a PhD candidate under the supervision of Prof. Dongling Ma and Prof. Mohamed Chaker at INRS, Canada. He received his master's degree from Shandong University (China) in 2015 and was awarded a 4 year pursuing PhD degree scholarship from the China Scholarship Council (CSC) and a Doctoral Research Scholarship from Fonds de recherche du Québec – Nature et technologies (FRQNT). His current research interest focuses on the synthesis of functional nanomaterials for applications in heterogeneous catalysis, including plasmon and upconversion enhanced photocatalytic degradation of pollutants and solar water splitting. |
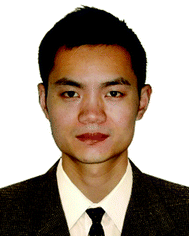
Fan Yang
| Fan Yang received his BSc and MSc degrees in Materials Science and Engineering from the China University of Geosciences in 2010 and 2013, respectively. He is currently a PhD candidate under the supervision of Prof. Dongling Ma and Prof. Fiorenzo Vetrone at INRS-EMT, and Prof. Xinyu Liu at the University of Toronto. His current research interest focuses on the design and development of multifunctional nanoplatforms based on superparamagnetic nanoparticles, near-infrared excited quantum dots and lanthanide-doped nanoparticles, and their application in cancer diagnostics and therapeutics. |
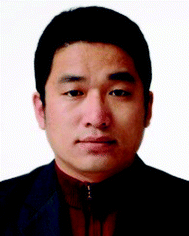
Zhenhe Xu
| Zhenhe Xu is currently an associate professor at Shenyang University of Chemical Technology. He received his PhD degree (inorganic chemistry) from the Changchun Institute of Applied Chemistry (CIAC), Chinese Academy of Sciences, in 2011. He was awarded the “ZhuLiYueHua Scholarship” by Chinese Academy of Sciences. He became a postdoctoral fellow in Prof. Ma's group at the center of Énergie, Matériaux et Télécommunications, Institut national de la recherche scientifique, the University of Quebec (Canada) in 2013. His current research interests include the development of nanostructured materials and multifunctional composite materials mainly for catalytic applications. |
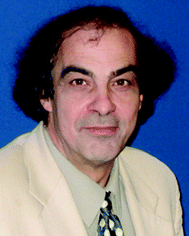
Mohamed Chaker
| Mohamed Chaker has been a professor at INRS since 1989. As a holder of Tier-I Canada Research Chair on “Plasma applied to micro- and nano-manufacturing technologies”, he is leading a research program in plasma-based synthesis and etching of innovative materials at the nanoscale for the fabrication of RF and photonic devices. In his career, his research work has resulted in 245 articles and over 300 conference presentations (including 50 invited presentations). From 1999 to 2005, he was the director of the Center Energy, Materials and Telecommunications of INRS. |
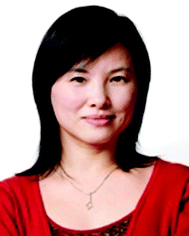
Dongling Ma
| Dongling Ma is currently a professor at INRS. Her main research interest consists in the development of various nanomaterials (e.g., quantum dots, catalytic nanoparticles, plasmonic nanostructures, and different types of nanohybrids) for applications in energy, catalysis and biomedical sectors. Before joining INRS in July 2006, she was awarded Natural Sciences and Engineering Research Council Visiting Fellowships and worked at the National Research Council of Canada from 2004 to 2006. She received her PhD degree from Rensselaer Polytechnic Institute (USA) in 2004. |
1. Introduction
Photocatalysis has maintained a strong foothold in potentially tackling the environmental pollution and energy crisis via directly utilizing solar energy, since the emergence of the pioneering study involving TiO2 in the 1970s.1–5 During the past few decades, the development of effective semiconductor photocatalysts for converting sunlight into chemical energy has been an immense research topic and residing at the frontier of sustainable energy exploration.6 Through the absorption of light with energy greater than the bandgap of the semiconductor, the electrons in the valence band (VB) are excited to the conduction band (CB), leaving behind the positively charged holes in the VB. The photoinduced charge carriers can either directly participate in photocatalysis, like in the case of water splitting, or first react with OH−/H2O and O2 to generate active species, which then play a pivotal role in photocatalytic decomposition of organic contaminants.7–18
Currently, restricted by their bandgap, most of the conventional photocatalysts can only respond to a small fraction of solar light mainly in the ultraviolet (UV) region and partially in the visible region.19 Near-infrared (NIR) photons, constituting ∼50% of the incident solar irradiation, remain underutilized.20 This undoubtedly imposes intrinsic limitations to the maximum achievable solar energy conversion efficiency, which is far from satisfactory for practical applications. Therefore, developing strategies to broaden the range of light absorption to the NIR region is desirable. But on the other hand, it is argued that NIR light cannot contribute significantly to photocatalysis due to its lower energy than UV and visible light. Moreover, many photocatalytic reactions, such as wastewater treatment and water splitting, need to be carried out in an aqueous system, which absorbs more NIR photons than visible photons.
Literature reports regarding NIR photocatalysts still remain quite limited as compared to visible photocatalysts. To date, NIR photocatalysis has been known to typically involve the use of noble metals, and a few available NIR dyes, which are usually of high-cost and/or suffer from thermal or other instability issues. A recently emerging and also debatable candidate for NIR photocatalysis is upconversion (UC) materials, which are stable, non-toxic, environmentally benign and of lower-cost with respect to noble metals. Photon UC is essentially a nonlinear optical process and has been observed in most notably lanthanide-doped crystals. It is an anti-Stokes emission process, whereby sequential absorption of two or more excitation photons with lower energy results in high-energy luminescence emissions.21–25 The rich energy level pattern of lanthanides originating from their split 4f electronic configurations by electronic repulsion and spin–orbit coupling renders them an ideal candidate for achieving efficient photon UC. By modulating lanthanide dopants, the lower-energy NIR photons can be upconverted to higher-energy emissions precisely, with wavelengths spanning from the UV to visible regions, which can then be absorbed by UV- or visible-absorbing photocatalytic materials, thereby allowing the “indirect” utilization of NIR solar energy for photocatalysis. But it is not question-free regarding this two-step process. Although fundamentally interesting, the low UC quantum yield (UCQY) and absorption preference (lasers with specific, limited wavelengths) of the UC materials raise doubts about their actual use in NIR photocatalysis from a practical point of view. In principle, strong absorption over a broad NIR range and a high UCQY are both essential for high-efficiency NIR photocatalysis. Of course the efficient use of upconverted, emitted photons by photocatalytic materials is also of paramount importance.
This Minireview focuses on the very latest advances in the field of lanthanide-doped UC materials, with particular emphasis on their emerging application in photocatalytic reactions. We begin our discussion by describing the synthetic methods used to prepare UC particles, and the strategies used to enhance the UCQY, and then summarize their application in photocatalysis mainly during the past three years. Finally, some future trends and perspectives in this field are discussed. We hope this review can initiate further thinking and discussion of readers about the role of lanthanide-doped UC materials in photocatalysis, and provide insights into the rational design of efficient broadband photocatalysts based on UC materials for future work.
2. Synthesis of lanthanide-doped UC materials
Lanthanide-doped UC materials typically consist of an inorganic crystalline host matrix (such as NaYF4) and trivalent lanthanide ions, acting as sensitizers (such as Yb3+) or activators (such as Er3+ and Tm3+), embedded in the host lattice.22 The luminescence properties of the UC materials, such as emission and excitation wavelengths, lifetimes and QYs, depend not only on their composition, but also on their crystallinity, size and shape as well as the defect concentration in the host.26,27 Subtly tuning the experimental conditions can substantially affect the UC luminescence properties. Hence, by selecting a well-defined synthetic method, it is possible to design UC structures that possess specific features for targeted applications. In this section, the latest studies are summarized to highlight the different synthetic routes, including thermal decomposition,28–36 hydro/solvothermal,37–42 and co-precipitation methods,43–48 and other emerging strategies for the synthesis of lanthanide-doped UC materials.
2.1. Thermal decomposition method
Thermal decomposition is the most effective method to prepare monodisperse and phase-pure lanthanide-doped UC nanocrystals with high crystallinity. This method involves the dissolving of organic precursors and surfactants in solvents with high boiling points, and the decomposition of the precursors at an elevated temperature. It is recognized that the selection of appropriate organic precursors and solvents is crucial for the successful synthesis of final products. For example, Wang et al. synthesized cubic phase NaYF4 and NaYF4@NaYbF4:Tm@NaYF4 core@shell@shell UC nanoparticles (UCNPs) by using metal trifluoroacetates as precursors for thermal decomposition in a solvent mixture of oleic acid (OA), oleylamine (OM), and octadecene (ODE) at 260 °C (Fig. 1a and b).30 Most recently, oleate-capped tetragonal-phase LiYF4:Tm/Yb NPs have been synthesized by Capobianco et al. with an average size of 81.1 ± 7.6 nm (Fig. 1c).49 The method has since become very popular for the preparation of high-quality (well-defined, highly crystalline, monodisperse and narrow size distribution) UCNPs; strictly controlled experimental conditions, such as temperature, time and pH,50 are the key to the success of synthesis. However, the synthesis procedure is complicated, and moreover, because the UCNPs obtained from this method are generally hydrophobic, further modification is necessary to make them hydrophilic for many applications, including aqueous phase photocatalysis.
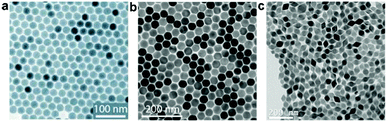 |
| Fig. 1 Transmission electron microscopy (TEM) images of the as-synthesized (a) NaYF4 and (b) NaYF4@NaYbF4:Tm@NaYF4 core@shell@shell UCNPs. Reprinted with permission from ref. 30. Copyright 2016, Nature Publishing Group. (c) TEM image of LiYF4:Tm/Yb UCNPs. Reprinted with permission from ref. 49. Copyright 2018, The Royal Society of Chemistry. | |
2.2. Hydro/solvothermal method
The hydro/solvothermal method is facile, environmentally benign and user-friendly, and has been used for the synthesis of hydrophobic or hydrophilic UC particles, which are difficult or impossible to achieve at atmospheric pressure and temperature limited by the boiling point of solvents. Prior to undergoing hydro/solvothermal treatment, a mixture is prepared by dissolving lanthanide nitrate/chloride precursors and fluoride precursors (HF, KF, NaF, NH4F, or NH4HF2) in surfactants and solvents, such as ODE, OA, OM, ethanol and water. Then, the mixture is normally introduced into a Teflon-lined autoclave, wherein it undergoes heating with a temperature above the boiling point of the solvent. Generally, in this method, the growth of the UC particles can be affected by many factors, such as the type of surfactants and lanthanide ions, their concentration, and the reaction temperature and time, as well as the pH value of the reaction mixture. For example, Liu and Huang et al. used ethylenediaminetetraacetic acid (EDTA) sodium salt and citric acid as surfactants and successfully prepared NaYF4 nanodisks (Fig. 2a) and nanorods (Fig. 2b) by regulating the orientation of epitaxial growth.37 The controlled growth can lead to the creation of multi-colour-emitting UC crystals at the level of a single particle. By using hydro/solvothermal approaches, various UC particles with different morphologies, crystal phases and hosts (such as NaLuF4,37,39 NaGdF4,40 NaYF4,37,38,51 NaYbF4,37 and CaF242) were synthesized by manipulating the interfacial reactions between different phases. Superior to the thermal decomposition method, the solvothermal strategy can realize the one-pot synthesis of hydrophilic UC materials directly with the addition of hydrophilic surfactants, such as citrates, polyethyleneimine (PEI), polyvinylpyrrolidone (PVP), cetyltrimethylammonium bromide (CTAB), binary acids, and poly(ethylene glycol) (PEG).37,50,52 However, the expensive autoclaves increase the cost of this method. Moreover, the invisible reaction process and the difficult instantaneous sampling at different time intervals render the formation mechanism of UC particles synthesized by this method rarely understood.
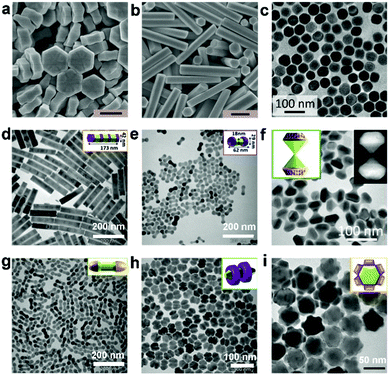 |
| Fig. 2 Scanning electron microscopy (SEM) images of (a) NaYF4:Yb/Tm nanodisks and (b) NaYF4:Yb/Er microrods synthesized by a solvothermal method (scale bar: 500 nm). Reprinted with permission from ref. 37. Copyright 2016, Wiley-VCH Verlag GmbH & Co. KGaA. (c) TEM image of NaYF4:Yb/Tm NPs prepared by a co-precipitation method. Reprinted with permission from ref. 47. Copyright 2018, American Association for the Advancement of Science. TEM images of three-dimensional UCNPs in (d) seven-section bamboo, (e) dumbbell, (f) hourglass, (g) round-end dumbbell, (h) double-ring and (i) flower shapes. Reprinted with permission from ref. 44. Copyright 2016, Nature Publishing Group. | |
2.3. Co-precipitation process
The co-precipitation method is essentially a multi-step method, and can be carried out in aqueous solution and organic solution.
For co-precipitation in aqueous solution, Ln(NO3)3 and LnCl3 are usually used to provide Ln3+ ions, and F− ions are provided by NaF and NH4F. A mixture of ethanol or methanol and water is always adopted as a solvent to assist the synthesis. Compared with the aforementioned two methods, the co-precipitation method in an aqueous system has been recognized as one of the most promising strategies in industrial applications for the synthesis of lanthanide-doped UC particles due to the convenient synthesis process, mild operating conditions, and use of inexpensive equipment. One of the limitations related to this method is that the synthesized particles show relatively low UC photoluminescence (UCPL) efficiency compared with those prepared by the thermal decomposition strategy. To solve this problem, a post-annealing treatment at high temperature is generally required to decrease the defects and generate high-crystallinity UC materials. After being subjected to such a kind of thermal treatment, some capping reagents can be carbonized, and thereby the hydrophilicity of the UC particles is greatly reduced. Furthermore, the morphologies of the UC particles synthesized by co-precipitation in aqueous solution are irregular and difficult to control.
Co-precipitation in organic solution involves dissolving a precipitator and cationic salts such as Ln(NO3)3 and LnCl3 in a high-boiling-point solvent mixture of OA/OM and ODE, and then they undergoe nucleation and growth, precipitation, filtration and calcination. NaOH and NH4F are normally used as precursors of Na+ and F− ions. Most recently, McHugh et al. synthesized uniform NaYF4:Yb/Tm UCNPs via a co-precipitation method (Fig. 2c).47 Also by using this method, Liu and Jin et al. prepared monodisperse particles with various three-dimensional shapes (Fig. 2d–i).44 It has been verified that the crystallographic facets of the particles can be directionally inhibited, promoted or etched by controlling the ratio of OA molecules and oleate anions. In addition, different types of UCNPs with different hosts, such as NaYF4,45,53 NaGdF4,54 LiYbF4,55 NaxZryFz,56 and KMnF3,48 have been prepared directly through this method during the past several years. Notably, the UC particles synthesized by this co-precipitation method in organic solution are monodisperse and uniform.
2.4. Other emerging methods
In addition to the abovementioned classic methods, some other approaches have been recently reported for the synthesis of UC particles, such as the sol–gel approach,57 micro-emulsion method,58 and microwave-assisted strategy.59,60 For instance, Zhou et al. synthesized NaLuF4:Gd,Yb,Er UCNPs by a one-step reverse micro-emulsion method for the first time.58 Although the obtained UCNPs show less perfect morphology than those prepared by other methods, they exhibited good hydrophilicity thanks to the surface –COOH group, and can be readily used in various biological and photocatalytic processes. Zhang et al. developed an ultrafast and environmentally benign strategy to synthesize NaBiF4:Yb/Ln (Ln = Er, Ho, and Tm) UC particles at room temperature.61 A mixture of nitrates, including Ln(NO3)3 (Ln = Yb/Er, Ho, and Tm), Bi(NO3)3 and NaNO3, ethylene glycol (EG) and NH4F underwent 1 min of vigorous stirring at room temperature, and then the final products were obtained. Microwave-assisted thermolysis was also explored to yield homogeneous particles, thanks to the uniform heating feature offered by microwave irradiation, in a very short time frame.62 In our group, we successfully prepared ultra-small NaGdF4:Tm,Yb@NaGdF4:Er,Yb core@shell and NaGdF4:Tm,Yb@NaGdF4:Er,Yb@NaGdF4 core@shell@shell NPs via this method.59 Obviously, the last two rapid-synthesis methods show great potential for the industrial, large-scale synthesis of UC particles.
3. Strategies for enhancing UC luminescence
As mentioned above, to realize efficient NIR photocatalysis, both highly efficient photon absorption and UC are critical. UC particles consist of an inorganic host matrix, and sensitizer and activator ions. The sensitizer ions can sequentially absorb two or more low-energy photons and transfer energy to activator ions to emit a higher-energy photon.50 Major underlying mechanisms are: excited state absorption (ESA), energy transfer UC (ETU), cooperative sensitization UC (CSU), energy migration-mediated UC (EMU) and photon avalanche (PA) processes.22 ETU generally occurs between a proximal sensitizer and an activator via dipole–dipole interactions and plays a dominating role in the generation of UC luminescence. In comparison, ESA is a basic process and the least efficient process for the generation of UC luminescence, while PA is dependent on pump power and exhibits a slow response to excitation. In the absence of physically existent intermediate states, UC can be realized by CSU or by EMU within a core@shell particle.22,50,63 The optical properties are thus largely governed by the type and concentration of the doped ions (i.e., activators and sensitizers), the crystal phase and crystallinity of the host, and the particle size and shape.50 For instance, poorer crystallinity results in a large number of defects and thus a lower UCQY.26
For absorption, usually specific-wavelength lasers are required and still UC particles exhibit relatively low absorption. Straightforwardly one would think that the absorption can be easily enhanced by increasing the doping levels. However, when a UC particle is doped with high concentrations of activators (e.g. Er3+ > 2 mol%) or sensitizers (Yb3+ > 20 mol%), the quenching of UCPL is inevitable and severe due to surface defects or cross-relaxation, eventually resulting in a low UCQY.24,64,65 To alleviate the detrimental quenching effect, the rational design of heterogeneously doped, multi-layered UC particles has been recently proposed, which allows us to precisely control the EMU process and increase the UCQY of highly doped UC particles.66
Although the UCQY is an important indicator of the optical quality of UC materials and highly relevant for many applications, including photocatalysis, most of the reports in this field do not provide QY data for their developed UC particles. This is possibly due to the difficulty in finding suitable references for measuring relative QYs,67 or the lack of access to integrating spheres for absolute QY measurements. The lack of measurement standards is another issue. Overall this situation makes the comparison and application of UC particles challenging. Among several groups who did investigations on the UCQY, Dionne et al. reported that, for sub-25 nm un-shelled NPs, the highest measured NIR-to-visible UCQY of 0.074% under an excitation power density of 63 W cm−2 UC was attained by β-NaY0.8–2xGdxLuxF4:Yb0.18Er0.02 NPs.68 Obviously, with such a low UCQY it is not possible to achieve efficient NIR photon harvesting and NIR photocatalysis. Strategies need to be developed to improve UCPL. To this end, in addition to developing highly-doped UC particles, four major strategies have been explored during the past several years (Fig. 3).
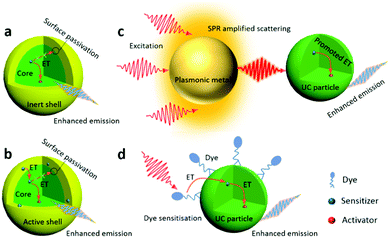 |
| Fig. 3 Strategies to enhance UC emission by constructing (a) core@inert-shell and (b) core@active-shell structures, by (c) coupling with plasmonic structures and by (d) sensitization with synthetic dyes. The dotted circles in (a and b) denote the non-radiative recombination site. | |
3.1. Constructing active-core@inert-shell structures
An effective solution to boosting the UCQY is to construct core@inert-shell (without any active dopants) structures, where the epitaxial shell can largely minimize the surface quenching of the UC particles to alleviate non-radiative decay (Fig. 3a).22 Recently, a series of UC core@shell structures have been developed, such as NaYF4:Yb/Tm@NaYF4,69,70 NaYF4:Yb/Er@NaGdF4, NaYF4:Yb/Er@NaYF4,71 NaYF4:Yb/Er@NaLuF4,72 NaYF4:Tm@NaYF4,73 NaYbF4:Ho@NaYF4,74 NaGdF4:Yb/Tm@NaGdF4,75 NaGdF4:Nd@NaGdF4,76 NaGdF4:Yb/Er@NaYF4,77 NaErF4:Ho@NaYF4,36 and NaErF4:Tm@NaYF4 UC particles.46 Huang and Liu et al. synthesized NaErF4:Tm@NaYF4 UCNPs by coating a NaYF4 shell onto the Er-enriched NaErF4:Tm core.46 The growth of the shell largely enhanced the emission at 654 nm by a factor of 708 via suppressing the concentration quenching as compared with that of the UC core. Alivisatos et al. measured an UCQY value of 4.0 ± 0.5% at 63 W cm−2 for β-NaYF4:Yb/Er@β-NaLuF4 nanocrystals with a shell thickness of 13 nm, which was about 57 times higher than that of the core-only particle.78 In 2017, Almutairi et al. synthesized NaErF4@NaLuF4 core@shell nanocrystals, and the measured UCQY of 5.2 ± 0.3% under 980 nm excitation at only 10 W cm−2 irradiance was considered to be the highest NIR-to-visible UCQY for irradiance below 100 W cm−2.64,79 Most recently, Haase et al. have reported a 45 nm-sized NaYF4:Yb/Er@NaYF4 NP with an inert shell thickness being equal to the radius of the core, which exhibited a UCQY value of 9% under NIR excitation (43 W cm−2).71 Thanks to all their efforts, the increase of UCQY from <1% to close to 10% represents a significant advancement of UC materials.
3.2. Constructing active-core@active-shell structures
In the above-mentioned examples, the shell is un-doped and inert, and its sole role is to protect the core from non-radiative decay caused by surface defects, or solvent molecules and ligands with high energy vibrations. It is reported that an active shell coating on the UC core particles can also enhance the UCPL intensity.80 For instance, it was found that a Yb3+-doped active shell can not only protect the core from non-radiative decay, but also facilitate the transfer of the absorbed NIR photons from the pump source to the core, leading to a higher energy transfer efficiency and UCQY (Fig. 3b). NaYF4:Yb/Er@NaLuF4:Yb/Tm microdisks developed by Huang and Liu et al. were such an example.37 The epitaxial growth of the NaLuF4:Yb/Tm shell greatly suppressed the surface quenching effect and a 30% enhancement was observed in the UCPL intensity of a single core/shell particle compared with that of NaYF4:Yb/Er. Li and Lin et al. designed and synthesized NaYF4:Yb,Er@NaYF4:Yb@NaNdF4:Yb@NaYF4:Yb core-multi-shell UCNPs, which showed an absolute QY of 0.18% at 540 nm (excitation: 808 nm, 31 W cm−2).81 The obtained QY was ∼12.8 times higher than that of NaYF4:Yb/Er@NaYF4:Yb@NaNdF4:Yb NPs. Recently, the use of this strategy has been extended to various UC particles, such as NaYbF4:Tb@NaYF4:Eu,82 NaYF4:Er@NaYF4@NaYF4:Yb/Tm@NaYF4,83 NaYbF4:Tb@NaYbF4@NaYbF4:Nd,84 and NaYbF4:Tm/Nd@CaF2:Nd@CaF2:Nd/Yb/Er,85 as well as NaGdF4:Yb,Er@NaYF4:Yb@NaGdF4:Yb,Nd@NaYF4@NaGdF4:Yb,Tm@NaYF4 UC particles.86
3.3. Coupling with plasmonic metals
The UCPL can also be enhanced by harnessing the surface plasmon resonance (SPR) effect (Fig. 3c). Many schemes have been developed to tune and optimize the interaction between the plasmonic metal and UC particles.87–89 These two components can be combined directly with direct, intimate contact via constructing core@shell or layer-on-layer structures. For example, Ko et al. fabricated an SPR-enhanced UC structure by depositing Ag nanowires directly onto a UCNP monolayer, in which the UCPL was enhanced by several tens of times with respect to that of the reference sample, due to the SPR-induced concentration of NIR light.88 Gordon et al. used Au nanorods (25 nm in diameter) with SPR peaks at 980 nm and 808 nm to selectively enhance the UC emission of NaYF4:Er UCNPs at 980 nm and at 808 nm, respectively, under excitation at >1200 nm.89 Plasmonic metal and UC particles can also be integrated “indirectly” by means of another material (such as TiO2,90 SiO2,91 graphene,92 and a metal–organic framework93) as a bridge in between them. In our group, an UC particle@TiO2 core@shell composite was firstly synthesized, and then plasmonic Au NPs were loaded onto the porous TiO2 shell. Note that the intensity of the SPR-enhanced UCPL can be estimated using eqn (1) and (2):where Φ is the photon flux of the excitation, δS is the absorption cross section of the sensitizer, ηET is the efficiency of the energy transfer from the sensitizer to the activator, φA is the UCQY of the activator, and E is the SPR-induced local electric field.
3.4. Dye sensitization
The sensitization amplification with synthetic NIR dyes is also a notable strategy to enhance UCPL (Fig. 3d).25,79,94–99 Due to the higher extinction coefficient and broader absorption band of NIR dyes, they exhibit a much higher absorption than Ln3+ ion sensitizers. IR 783,100 IR 806,97,101 IR 808,97,98 IR 820,97 IR 845,100 indocyanine green,94,95 and Cyto 840 dyes102 are the commonly-used dye sensitizers for UC particles, as their emission bands overlap with the absorption spectra of Ln3+ ion sensitizers, Yb3+ (∼980 nm) or Nd3+ ions (∼740/808 nm). Generally speaking, the anchored NIR dyes can strongly harvest NIR excitation light, and then trigger a multistep directional non-radiative energy transfer to excite Ln3+ ions to realize a more efficient UC process. Prasad et al. used a NIR dye (IR-808) to sensitize NaYbF4:Tm@NaYF4:Nd core/shell NPs, and a high UCQY of ∼4.8% was obtained at 10 W cm−2 of 800 nm.97 Most recently, Yang and Lin et al. synthesized IR 808-sensitized NaGdF4:Yb/Er@NaGdF4:Nd/Yb core@shell UCNPs.98 As the dominating emission peak in the NIR range of IR-808 overlaps very well with the absorption of both Nd3+ and Yb3+ ions, the IR 808-sensitized UCNPs with the Nd/Yb doped shell showed greatly enhanced UCPL upon excitation with an 808 nm laser. In addition, Li et al. synthesized water-dispersible Cy7 dye-sensitized NaYF4:Yb/Nd/Er@NaYF4:Nd UCNPs with about 17 times enhancement of UCPL.103 The enhanced UCPL arose from the combined advantages of both the core@shell structure and the dye sensitization. The NIR-driven photocatalysis will definitely benefit from the enhancement of UCPL.
4. Application in photocatalysis
For the UC enhanced photocatalysis, the integration of UC materials with semiconductor photocatalysts, such as TiO2,90,104,105 C3N4,106 CdS,107–109 and BiVO4,110 to form composites is one of the most used strategies to achieve NIR photocatalytic activity. The selection of lanthanide ions (or semiconductor materials) is dictated by the absorption feature of the semiconductor (or the UC emission feature of UC materials) in the composites. Specifically, the upconverted emissions by the lanthanide-doped UC particles should be capable of exciting the proximal semiconductor photocatalyst to produce charge carriers for targeted photocatalytic reactions (Fig. 4).
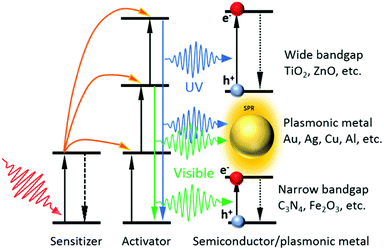 |
| Fig. 4 Illustration of the UC enhanced photocatalysis. | |
The most commonly used lanthanide ions in NIR photocatalysis are Yb3+, Tm3+ and Er3+. Yb3+ can strongly absorb at 980 nm, and usually serves as a sensitizer. It only possesses one excited state and once excited, its energy can be transferred to Tm3+ and Er3+ ions, which act as activators to generate UV, blue and visible emissions via the successive energy transfer processes. As Yb3+ ions are almost exclusively required, we categorize the most recent advancements in UC enhanced photocatalysis based on the involvement of different activators, i.e. Tm3+ ions,104,105,108,110–114 Er3+ ions,109,115–119 and their combination.90,93,106,120,121 Some latest representative photocatalysts involving lanthanide-doped UC materials and their application in specific photocatalytic reactions are summarized in Table 1.
Table 1 Summary of representative photocatalysts involving lanthanide-doped UC particles for photocatalysis
Photocatalysts |
Photochemical reactionsa |
Ref. |
RhB (rhodamine B), MB (methyl blue), MO (methyl orange) dyes.
|
NaYF4:Yb/Tm-Cu2ZnSnS4 |
Photodegradation of RhB |
113
|
NaYF4:Yb/Tm-BiOCl |
Photodegradation of MB |
111
|
NaYF4:Yb/Er@TiO2-Ag6Si2O7 |
Photodegradation of MB |
117
|
NaYF4:Yb/Er-Au-CdS |
Photoreforming bioethanol |
109
|
NaYF4:Yb/Er/Tm@TiO2-Au |
Photodegradation of MO |
90
|
NaYF4:Yb/Er/Tm-C3N4-Au |
Photodegradation of MO |
106
|
NaYF4:Yb/Tm/Er-Pt@MOF-Au |
Solar water splitting |
93
|
4.1. Yb3+ and Tm3+ doped UC particles
The UC materials co-doped with Yb3+ and Tm3+ ions show strong emissions in the UV (291, 346 and 361 nm), visible (451, 475, 696 and 726 nm) and NIR (800 nm) regions. The UC UV emissions can excite most of the conventional photocatalysts, including TiO2,104,105 CdS,108 BiOCl,111 TiO2/CdS,107 ZnO/Cu2ZnSnS4 and ZnO/Cu2ZnSnSe4 hybrids,113 ZnO/CeO2 heterostructures,122 and BiPO4/BiVO4 nanocomposites,110 and recently emerging catalysts, such as C3N4,112 CeO2,123 SnO2,124 and In2S3.114 For instance, NaYF4:Yb/Tm UCNPs loaded on different facets of BiOCl nanoplates were synthesized by Bai et al.111 The {110} facets of BiOCl were found to show stronger absorption for the UV emissions from NaYF4:Yb/Tm UCNPs under 980 nm laser irradiation than the {001} facets, resulting in a higher photocatalytic performance of NaYF4:Yb,Tm-{110}BiOCl in the degradation of rhodamine B (RhB) and methylene blue (MB) dyes. They achieved >6 times higher degradation rate than pristine BiOCl without UCNP loading. Very recently, Zhang et al. reported Yb/Tm co-doped mesoporous hollow CeO2 UCNPs (Ce-UCNPs) (Fig. 5a), in which the UC UV emissions can excite the CeO2 matrix to produce charge carriers (Fig. 5b).123 The photo-induced electrons and holes in CeO2 effectively reacted with O2 and H2O to generate super oxide (˙O2−) and hydroxyl (˙OH) radicals, respectively, for the degradation of methyl orange (MO) (Fig. 5c). The absence of UV emissions in the UCPL spectrum of Ce-UCNPs indicated that the UC UV light was absorbed by CeO2 to generate photoinduced charge carriers (Fig. 5d). Contrarily, the blue emissions cannot be used to excite CeO2 due to its wide bandgap (2.89 eV), resulting in low energy transfer efficiency from UCNPs. To efficiently utilize the UC emissions both in the UV and blue regions from Tm3+, some suitable narrow-bandgap semiconductors (aminobenzonitrile-doped C3N4112 and In2S3114), composites (TiO2/CdS,107 ZnO/Cu2ZnSnS4 and ZnO/Cu2ZnSnSe4 hydrids113) and plasmonic nanostructures (SnO2@Ag124) have been developed and coupled with Yb/Tm-doped UC particles.
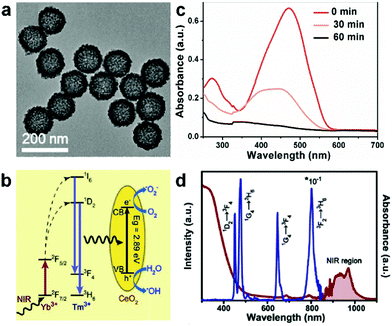 |
| Fig. 5 (a) TEM image of mesoporous hollow Ce-UCNPs after calcination. (b) Schematic illumination of the UC, photocatalysis, and enzyme-like catalysis mechanism of the Ce-UCNPs (from left to right). (c) UV-vis absorption spectra of MO solution treated with Ce-UCNPs under 980 nm NIR irradiation with different time periods. (d) The UV-Vis-NIR absorption and UC luminescence of the Ce-UCNPs. Reprinted with permission from ref. 123. Copyright 2018, Wiley-VCH Verlag GmbH & Co. KGaA. | |
4.2. Yb3+ and Er3+ doped UC particles
Yb/Er-doped UC particles are known for their UC emissions in the green (515–565 nm) and red (640–675 nm) regions, but without any considerable emission in the UV range.36 This requires the corresponding photocatalyst to be able to strongly absorb visible light in order to take good advantage of UC. For example, Jiang et al. integrated the Z-scheme TiO2-Ag6Si2O7 photocatalyst with UC microplates by synthesizing a β-NaYF4:Yb/Er core@TiO2-Ag6Si2O7 shell (NaYF4@T-ASO) nanostructure.117 The Z-scheme NaYF4@T-ASO composite can not only promote charge transfer and retain the respective strong redox abilities of the constituent components, but also capture the UC visible emissions from NaYF4:Yb/Er UC microplates which was verified by the quenching of the UC emission following the combination of other components (Fig. 6a and b). Thus, the synthesized NaYF4@T-ASO showed NIR photocatalytic activity in the degradation of MB (Fig. 6c). In addition, under simulated solar light irradiation, the advantages of the NaYF4@Z-scheme structure can be fully taken and a greatly enhanced photocatalytic performance was achieved, which was more than 10 times higher than that of P25 and ASO (Fig. 6d). Liu et al. utilized the SPR effect of Au NPs (SPR from ∼500 nm to ∼700 nm) and narrow-bandgap CdS (absorption edge: 537 nm) to reabsorb the UC visible photons.109 The as-prepared NaYF4:Yb/Er-Au-CdS samples showed enhanced NIR-driven photocatalytic activity in the H2 evolution from bio-ethanol. It is noteworthy that no H2 was detected in the presence of NaYF4:Yb/Er-CdS without loading Au NPs under NIR light, confirming that the introduction of the SPR effect of Au can facilitate the utilization of UC visible emissions from NaYF4:Yb/Er particles to activate the photocatalytic process. Obviously, the efficient application of the Yb/Er-doped UC materials requires the integration with narrow-bandgap semiconductors, plasmonic materials and/or composites which can absorb the UC green and red emissions.
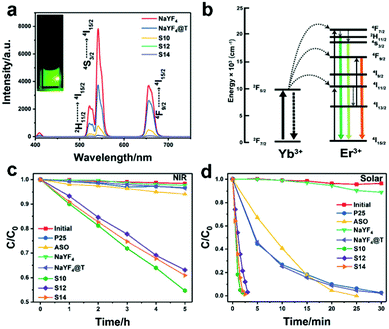 |
| Fig. 6 (a) The UC spectra of the NaYF4 UC microplates, NaYF4@T, and NaYF4@T-ASO prepared with pump rates of AgNO3 solution at 10 (S10), 12 (S12), and 14 rpm (S14) under 980 nm irradiation (inset shows the image of the NaYF4 UC microplate colloids). (b) The schematic of the UCPL process of NaYF4:Yb3+, Er3+ UC microplates. The photocatalytic activities of the as-obtained samples under (c) NIR and (d) solar light irradiation. Reprinted with permission from ref. 117. Copyright 2017, The Royal Society of Chemistry. | |
4.3. Yb3+, Tm3+ and Er3+ doped UC particles
The UC emissions in the UV and visible regions can be achieved simultaneously by the UC materials co-doped with Yb3+, Tm3+ and Er3+ ions, and are expected to increase the QY and the photocatalytic activity under 980 nm light illumination. Our group prepared the β-NaYF4:Yb/Er/Tm (NYF) particles via a hydrothermal method, and then integrated them with plasmonic Au NPs and TiO2 to get NYF core@porous TiO2-Au shell (NYF@TiO2-Au) microspheres (Fig. 7a–d).90 The obtained NYF@TiO2-Au samples exhibited enhanced absorption in the UV, visible and NIR regions. The quenching of the UV emission peaks with the growth of the TiO2 shell and the quenching of green emission with the loading of Au NPs in the UC spectra strongly supported the absorption of TiO2, and Au NPs due to their interband and SPR excitations and the energy transfer from the UC cores (Fig. 7e). To confirm our understanding of the optical properties of the NYF@TiO2-Au microspheres, optical modelling was performed for the emission of the core@shell structure. The TiO2-AuNP shell and TiO2 nanosheet shell were modelled as a mixture of their corresponding media (air) using the conventional Maxwell-Garnett method. The UC emission spectrum of the NYF particles was multiplied separately by the calculated transmission coefficient of the two shells. The obtained UCPL spectra were quite qualitatively consistent with the experimental ones. The high NIR photocatalytic activity of NYF@TiO2-Au was observed in the degradation of MO. It was also found that the coating of the TiO2 shell on the NYF core led to the increase of MO degradation from 0% to 53%, and further to 75% after subsequently loading 1 wt% of Au NPs, indicating that the energy transfer from UC particles to TiO2 doubles that from NYF to Au NPs in this specific system. Furthermore, excellent UV and visible photocatalytic performances were also achieved by the prepared NYF@TiO2-Au samples, greatly better than the benchmark P25 TiO2 (Fig. 7f). This can be attributed to the rational structure design of the NYF core@porous TiO2-Au shell: (i) the close contact between each component led to efficient charge and energy transfer and (ii) the porous shell reduced charge carrier recombination. Specifically, under UV excitation the electrons are transferred from TiO2 to Au NPs, and under visible irradiation the SPR-induced hot electrons are injected from Au to TiO2, leading to a longer charge carrier lifetime and enhanced UV and visible light photocatalytic activities, respectively.
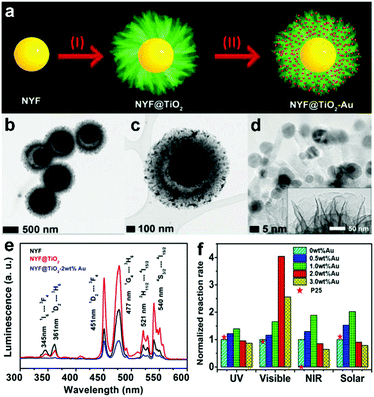 |
| Fig. 7 (a) Schematic illustration of the formation process of the NYF@TiO2-Au core@shell microspheres. (b–d) TEM images of the microspheres in different magnifications. The inset in (d) shows the thin nanosheet structure of the shell. (e) UCPL spectra of NYF, NYF@TiO2, and NYF@TiO2-Au samples. (f) Photocatalytic degradation rate of MO in the presence of the NYF@TiO2 loaded with varying Au contents and P25 TiO2 under different light irradiations. Reprinted with permission from ref. 90. Copyright 2015, Wiley-VCH Verlag GmbH & Co. KGaA. | |
To circumvent the less efficient utilization of UC blue emissions by NYF@TiO2-Au, a photocatalyst (Au-NYF/g-C3N4) composed of Au NPs and β-NaYF4:Yb/Er/Tm microspheres loaded on UV/blue-absorbing graphitic C3N4 (g-C3N4) nanosheets was subtly designed and synthesized by our group.106 The first reported in situ synthesis of NYF on g-C3N4 nanosheets resulted in high NYF yields and high coupling efficiency between the two components and the synthesis is also highly reproducible. The absorption of g-C3N4 due to a narrower bandgap (∼2.7 eV, absorbing up to ∼460 nm) and SPR excitation (from ∼500 to ∼700 nm) of Au NPs well overlap the UC emissions ranging from the UV to visible region (Fig. 8a and b). In addition, the very thin g-C3N4 nanosheets decreased the diffusion distance of charge carriers, made them directly accessible to reactants, and thereby largely suppressed their recombination. As a result, Au-NYF/g-C3N4 exhibited enhanced photocatalytic performance in the degradation of MO under the irradiation range from UV, to visible to NIR. The SPR effect of the Au NPs contributed to the enhanced NIR-photoactivity of Au-NYF/g-C3N4, and the obtained reaction rate was over 2 times higher than that of the NYF/g-C3N4 sample. Furthermore, the underlying charge transfer pathways and possible mechanisms were proposed in detail under different light irradiations, as clearly shown in Fig. 8c–e.
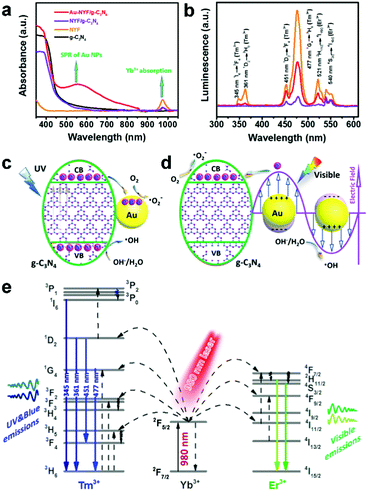 |
| Fig. 8 (a) UV-vis-NIR absorption spectra and (b) UCPL spectra of the obtained samples. The inset in (a) is the absorption spectrum of the Au NPs in water. The proposed photocatalytic mechanism of the Au-NYF/g-C3N4 photocatalyst under (c) UV and (d) visible light (λ > 475 nm) irradiation, respectively. (e) Schematic energy level diagrams, upconversion excitation, and UV-vis light emissions for the NYF microspheres under NIR light (980 nm laser) illumination. Reprinted with permission from ref. 106. Copyright 2017, American Chemical Society. | |
Through a similar strategy, integrating SPR, UC and semiconductors into one architecture for photocatalysis, most recently, Jiang et al. have reported a UCNPs-Pt@metal–organic framework (MOF)/Au composite for photocatalytic H2 evolution.93 Similarly, NaYF4:Yb, Tm, Er UC particles were used to upconvert NIR to UV and visible photons, which can be reabsorbed by MOF and Au NPs for photocatalysis (Fig. 9a and b). Pt NPs acted as a co-catalyst by efficiently capturing photoinduced electrons from the system for water reduction to produce H2. As a result, H2 was detected in the presence of the prepared UCNPs-Pt@MOF/Au composite with the separate irradiation of UV, visible and NIR light (Fig. 9c). The introduction of the SPR effect of the Au NPs not only made UCNPs-Pt@MOF/Au visible-light-responsive, but also enhanced its NIR-light photocatalytic activity with the H2 production rate of 34 μmol g−1 h−1, which was more than 2 times higher than that of the UCNPs-Pt@MOF sample. Furthermore, the composite with optimized Au loading (0.3 wt%) exhibited a high H2 generation rate (280 μmol g−1 h−1) and good recycling performance under simulated solar light illumination (Fig. 9d).
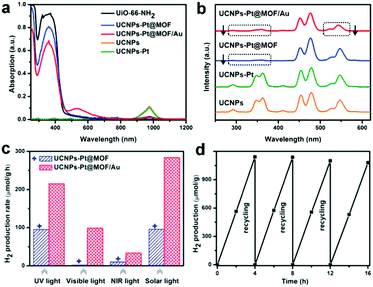 |
| Fig. 9 (a) UV-vis-NIR absorption spectra, and (b) UCPL spectra under excitation at 980 nm for different samples. (c) Comparison of the H2 production rates of UCNPs-Pt@MOF and UCNPs-Pt@MOF/Au under UV (200–400 nm), visible (420–800 nm), NIR (980 nm), and simulated solar light irradiation. (d) Recycling performance of UCNPs-Pt@MOF/Au under simulated solar light. Reprinted with permission from ref. 93. Copyright 2018, Wiley-VCH Verlag GmbH & Co. KGaA. | |
4.4. Other lanthanide ion-doped UC particles
In addition to the above mentioned UC materials, some other lanthanide ion-doped UC particles/semiconductor composites, such as Er-YAlO3/TiO2,125 Pr-Y2SiO5/Pr-CaTiO3/Pt,126 and CaTiO3/Pr-Y2SiO5/reduced graphene oxide,127 have been reported to upconvert visible light to UV emissions, which can be used to excite the coupled semiconductor for photocatalysis.
5. Conclusions and perspectives
Lanthanide-doped UC materials have attracted extensive attention in bio-applications, such as multimodal imaging, sensing, and photodynamic and photo-thermal therapies. Their application in photocatalysis has just started, representing an emerging field filled with many exciting possibilities as well as challenges. In this review, we have summarized the very latest advances in the synthetic methods, strategies for enhancing UCPL and photocatalytic applications of UC particles.
Although UC materials have shown great potential in broadening the optical response of photocatalysts from UV and visible to NIR regimes, there are still a number of constraints in the practical application of UC particles for photocatalytic reactions. One of the major restrictions is the low absorption capacity of lanthanide-doped UC materials. As mentioned above, Yb3+ ions are typically required to act as a sensitizer, which only shows a single narrow absorption band centred at 980 nm. Recently, other sensitizers (such as Nd3+ ions) with strong absorption at 808 nm have attracted much attention due to their large absorption cross sections and also much less absorption by water at 808 nm as compared with other excitation wavelengths. It is attractive to explore diverse UC particles sensitized by different types of dopants in water-based photocatalysis. Moreover, using a mixture of UC particles containing different types of sensitizer dopants also deserves future investigation in order to broaden effective light absorption. Nevertheless, lasers with limited choices or concentrated sunlight are still required to act as an irradiation source. Efficient interaction of natural sunlight with UC particles represents a big challenge.
To enhance the light absorption ability, developing UC materials which can be excited by broadband irradiations through introducing some materials (e.g. synthetic dyes, transition metals and quantum dots) with large absorption cross sections appears very feasible. However, for the composite UC photocatalyst, the energy transfer mechanisms inside UC particles, between UC particles and sensitizers, and between UC particles and photocatalysts are sophisticated and remain enigmatic and need to be further explored. The introduction of the SPR effect can concentrate the excitation light source and thus effectively enhance light absorption also. But sometimes decreased UCPL was measured, which may be due to the non-radiative energy transfer process. To reveal the exciton dynamics and better understand the complicated energy transfer processes, the direction and the time scale of energy flow should be identified. Finding answers to these questions requires the development of in situ and ultrafast characterization techniques, systematic experiments and further parallel theoretical studies which can take all possible processes, absorption and radiative or non-radiative energy transfer, into consideration.
Another challenge that the UC community faces is the low QY of the UC particles. Thus far, the activators for an efficient UC process have been mainly restricted to Tm3+, Er3+ and Tm3+/Er3+ ions, with the highest internal QY being estimated as 7.6% for LiLuF4:Yb/Tm@LiLuF4 NPs under a light irradiance of 127 W cm−2. The low energy transfer efficiency from the sensitizer to the activator, and the quenching of UCPL caused by cross-relaxation and the energy losses due to surface defects lead to a low UCQY.
To boost the QY of the UC particles, as we have demonstrated, recent efforts have been mainly made toward the investigations of broadband material sensitization, surface plasmon coupling, surface passivation, etc. In addition to enhancing light absorption, coupling with plasmonic metals and sensitization with dyes can also increase the energy transfer rate and efficiency, and thus results in an enhanced UCQY. Another approach towards enhancing the UCQY is to suppress the non-radiative decay of the UCPL. One of the most effective ways is to construct core@shell UC structures, which can largely mitigate the cross-relaxation and the energy losses due to surface defects. For a long time, although UCQY is an important indicator of the optical quality of UC materials and highly relevant for many applications, most of the studies in this field did not provide QY data. The lack of a suitable reference renders the relative QY measurement very difficult. The absolute QY measured by using integrating spheres has been provided in some literature studies, but employed methodologies for the measurement are not always identical. Since the UCQY is power-dependent, the value is always provided at a specific pump power density. To compare the UCQY in different UC systems in a fair and consistent way, a reliable and standard measurement method and rigorous evaluation and analysis systems should be constructed.
To make full utilization of UC emissions, the rational structure design of UC particle–semiconductor (and/or -plasmonic) photocatalysts is of great significance. For example, the upconverting-core@semiconductor-shell structure allows UC photons in all directions to have a chance of being absorbed, and the close contact between the UC materials and semiconductors greatly facilitates the energy transfer between them. The selection of suitable photocatalysts and dopants, enabling as large a spectral overlap as possible between the UC emissions and semiconductor absorption, is very important in harvesting the emissions from UC particles. Plasmonic metal NPs, such as Al, Cu, Ag and Au NPs, with different SPR excitation wavelengths (from UV to visible) provide additional channels for capturing UC emissions, including the UV part (can be used to excite Al NP plasmons). Currently, most of the studies on plasmon and UC enhanced photocatalysis focus on plasmonic metals (e.g. Au, Ag and Cu NPs) with SPR wavelengths in the visible range, while reports on UV-active plasmonic nanostructures are rare. Actually, UC particles doped with some lanthanide ions, such as Tm3+, Gd3+ and Ho3+, can generate UV emissions under the excitation of NIR light. These UV emissions can be used to excite the SPR of UV-active plasmonic nanostructures (mainly referring to Al NPs), which can, in turn, enhance the generated UV emissions. It is known that UV photons possess much higher energy than visible and NIR photons, favourable for photocatalysis, and the energy transfer from UC particles to UV-active materials could also be more effective than that to visible-active materials. Considering these factors, plasmonic Al NPs show great potential in UC particle-enabled photocatalysis. Thanks to the well-studied synthetic methods of plasmonic metal NPs the SPR effect has great potential in the enhancement of UC-involved photocatalysis.
On another note, most of the reported UC photocatalysts are used in photocatalytic degradation reactions, and only a few have been used for H2 evolution from water splitting, and even much less for artificial photosynthesis. Facing the growing serious energy crisis and global warming issues, more attention is urgently needed on photocatalytic water splitting and CO2 reduction by using UC photocatalysts, albeit challenging.
In short, recent progress in high-quality UC particle synthesis, rational composite material design, and introduction of plasmonic nanomaterials into photocatalysis makes the use of UC particles in NIR photocatalysis increasingly more attractive than ever before, although intensive work is still required before a sound conclusion can be made on how useful UC particles can really be and for dramatic advancements and even breakthroughs in NIR photocatalysis, at a meaningful level from a practical perspective.
Conflicts of interest
There are no conflicts to declare.
Acknowledgements
Financial support from the Natural Sciences and Engineering Research Council of Canada (NSERC) in the context of NSERC-Discovery Grant and NSERC-Strategic Grant (with the support of Canadian Solar Inc.), and le Fonds de recherche du Quebec-Nature et technologies (FRQNT) is greatly appreciated. M. C. is also grateful to the Canada Research Chairs Program. Q. Z. acknowledges the support from the China Scholarship Council (CSC, no. 201506220152).
Notes and references
- A. Fujishima and K. Honda, Nature, 1972, 238, 37–38 CrossRef CAS PubMed.
- W. J. Ong, L. L. Tan, Y. H. Ng, S. T. Yong and S. P. Chai, Chem. Rev., 2016, 116, 7159–7329 CrossRef CAS PubMed.
- M. Q. Yang and Y. J. Xu, Nanoscale Horiz., 2016, 1, 185–200 RSC.
- Z. Xu, M. G. Kibria, B. AlOtaibi, P. N. Duchesne, L. V. Besteiro, Y. Gao, Q. Zhang, Z. Mi, P. Zhang, A. O. Govorov, L. Mai, M. Chaker and D. Ma, Appl. Catal., B, 2018, 221, 77–85 CrossRef CAS.
- Q. Zhang, X. Jin, Z. Xu, J. Zhang, U. F. Rendon, L. Razzari, M. Chaker and D. Ma, J. Phys. Chem. Lett., 2018, 9, 5317–5326 CrossRef CAS PubMed.
- X. Cao, Z. Chen, R. Lin, W.-C. Cheong, S. Liu, J. Zhang, Q. Peng, C. Chen, T. Han, X. Tong, Y. Wang, R. Shen, W. Zhu, D. Wang and Y. Li, Nat. Catal., 2018, 1, 704–710 CrossRef.
- Q. Zhang, N. Bao, X. Wang, X. Hu, X. Miao, M. Chaker and D. Ma, Sci. Rep., 2016, 6, 38066 CrossRef CAS PubMed.
- H. Hussain, G. Tocci, T. Woolcot, X. Torrelles, C. Pang, D. Humphrey, C. Yim, D. Grinter, G. Cabailh and O. Bikondoa, Nat. Mater., 2016, 16, 461–466 CrossRef PubMed.
- H. Park, H. I. Kim, G. H. Moon and W. Choi, Energy Environ. Sci., 2016, 9, 411–433 RSC.
- S. K. Cushing and N. Q. Wu, J. Phys. Chem. Lett., 2016, 7, 666–675 CrossRef CAS PubMed.
- Z. H. Xu, Y. L. Liu, F. Q. Ren, F. Yang and D. L. Ma, Coord. Chem. Rev., 2016, 320, 153–180 CrossRef.
- G. J. Ma, S. S. Chen, Y. B. Kuang, S. Akiyama, T. Hisatomi, M. Nakabayashi, N. Shibata, M. Katayama, T. Minegishi and K. Domen, J. Phys. Chem. Lett., 2016, 7, 3892–3896 CrossRef CAS PubMed.
- P. V. Kamat, Acc. Chem. Res., 2017, 50, 527–531 CrossRef CAS PubMed.
- S. K. Cushing, F. K. Meng, J. Y. Zhang, B. F. Ding, C. K. Chen, C. J. Chen, R. S. Liu, A. D. Bristow, J. Bright, P. Zheng and N. Q. Wu, ACS Catal., 2017, 7, 1742–1748 CrossRef CAS.
- Z. X. Zhou, Y. Y. Zhang, Y. F. Shen, S. Q. Liu and Y. J. Zhang, Chem. Soc. Rev., 2018, 47, 2298–2321 RSC.
- Y. Gao, J. Lin, Q. Zhang, H. Yu, F. Ding, B. Xu, Y. Sun and Z. Xu, Appl. Catal., B, 2018, 224, 586–593 CrossRef CAS.
- R. He, D. Xu, B. Cheng, J. Yu and W. Ho, Nanoscale Horiz., 2018, 3, 464–504 RSC.
- G. Wang, Q. Zhang, Q. Chen, X. Ma, Y. Xin, X. Zhu, M. Dong, C. Cui, J. Zhang and Z. Xiao, Chem. Eng. J., 2019, 358, 1083–1090 CrossRef CAS.
- Q. Zhang, D. Thrithamarassery Gangadharan, Y. Liu, Z. Xu, M. Chaker and D. Ma, J. Materiomics, 2017, 3, 33–50 CrossRef.
- F. Liu, M. Zeng, Y. Z. Li, Y. Yang, M. Y. Mao and X. J. Zhao, Adv. Funct. Mater., 2016, 26, 4518–4526 CrossRef CAS.
- F. Auzel, Chem. Rev., 2004, 104, 139–173 CrossRef CAS PubMed.
- B. Zhou, B. Y. Shi, D. Y. Jin and X. G. Liu, Nat. Nanotechnol., 2015, 10, 924–936 CrossRef CAS PubMed.
- X. G. Liu, C. H. Yan and J. A. Capobianco, Chem. Soc. Rev., 2015, 44, 1299–1301 RSC.
- Y. J. Liu, Y. Q. Lu, X. S. Yang, X. L. Zheng, S. H. Wen, F. Wang, X. Vidal, J. B. Zhao, D. M. Liu, Z. G. Zhou, C. S. Ma, J. J. Zhou, J. A. Piper, P. Xi and D. Y. Jin, Nature, 2017, 543, 229–233 CrossRef CAS PubMed.
- B. Xue, D. Wang, L. P. Tu, D. P. Sun, P. T. Jing, Y. L. Chang, Y. L. Zhang, X. M. Liu, J. Zuo, J. Song, J. L. Qu, E. J. Meijer, H. Zhang and X. G. Kong, J. Phys. Chem. Lett., 2018, 9, 4625–4631 CrossRef CAS PubMed.
- X. Qin, X. W. Liu, W. Huang, M. Bettinelli and X. G. Liu, Chem. Rev., 2017, 117, 4488–4527 CrossRef CAS PubMed.
- Y. Li, M. Gecevicius and J. R. Qiu, Chem. Soc. Rev., 2016, 45, 2090–2136 RSC.
- Y. Li, J. L. Tang, D. X. Pan, L. D. Sun, C. Y. Chen, Y. Liu, Y. F. Wang, S. Shi and C. H. Yan, ACS Nano, 2016, 10, 2766–2773 CrossRef CAS PubMed.
- X. H. Zhang, B. Blasiak, A. J. Marenco, S. Trudel, B. Tomanek and F. C. J. M. van Veggel, Chem. Mater., 2016, 28, 3060–3072 CrossRef CAS.
- X. Chen, L. M. Jin, W. Kong, T. Y. Sun, W. F. Zhang, X. H. Liu, J. Fan, S. F. Yu and F. Wang, Nat. Commun., 2016, 7, 10304 CrossRef CAS PubMed.
- L. Feng, F. He, B. Liu, G. Yang, S. Gai, P. Yang, C. Li, Y. Dai, R. Lv and J. Lin, Chem. Mater., 2016, 28, 7935–7946 CrossRef CAS.
- Z. Zhuo, Y. S. Liu, D. J. Liu, P. Huang, F. L. Jiang, X. Y. Chen and M. C. Hong, Chem. Sci., 2017, 8, 5050–5056 RSC.
- H. Dong, L. D. Sun, L. D. Li, R. Si, R. Liu and C. H. Yan, J. Am. Chem. Soc., 2017, 139, 18492–18495 CrossRef CAS PubMed.
- J. T. Xu, W. Han, Z. Y. Cheng, P. P. Yang, H. T. Bi, D. Yang, N. Niu, F. He, S. L. Gai and J. Lin, Chem. Sci., 2018, 9, 3233–3247 RSC.
- H. T. Bi, Y. L. Dai, P. P. Yang, J. T. Xu, D. Yang, S. L. Gai, F. He, B. Liu, C. N. Zhong, G. H. An and J. Lin, Small, 2018, 14, 1703809 CrossRef PubMed.
- L. Liu, S. Wang, B. Zhao, P. Pei, Y. Fan, X. Li and F. Zhang, Angew. Chem., Int. Ed., 2018, 57, 7518–7522 CrossRef CAS PubMed.
- Y. H. Zhang, L. Huang and X. G. Liu, Angew. Chem., Int. Ed., 2016, 55, 5718–5722 CrossRef CAS PubMed.
- P. Rodriguez-Sevilla, Y. H. Zhang, N. de Sousa, M. I. Marques, F. Sanz-Rodriguez, D. Jaque, X. G. Liu and P. Haro-Gonzalez, Nano Lett., 2016, 16, 8005–8014 CrossRef CAS PubMed.
- X. J. Zhu, W. Feng, J. Chang, Y. W. Tan, J. C. Li, M. Chen, Y. Sun and F. Y. Li, Nat. Commun., 2016, 7, 10437 CrossRef CAS PubMed.
- X. W. Liu, Y. Wang, X. Y. Li, Z. G. Yi, R. R. Deng, L. L. Liang, X. J. Xie, D. T. B. Loong, S. Y. Song, D. Y. Fan, A. H. All, H. J. Zhang, L. Huang and X. G. Liu, Nat. Commun., 2017, 8, 899 CrossRef PubMed.
- X. W. Liu, X. Y. Li, X. Qin, X. J. Xie, L. Huang and X. G. Liu, Adv. Mater., 2017, 29, 1702315 CrossRef PubMed.
- M. Quintanilla, Y. Zhang and L. M. Liz-Marzan, Chem. Mater., 2018, 30, 2819–2828 CrossRef CAS.
- S. Y. Han, X. Qin, Z. F. An, Y. H. Zhu, L. L. Liang, Y. Han, W. Huang and X. G. Liu, Nat. Commun., 2016, 7, 13059 CrossRef CAS PubMed.
- D. M. Liu, X. X. Xu, Y. Du, X. Qin, Y. H. Zhang, C. S. Ma, S. H. Wen, W. Ren, E. M. Goldys, J. A. Piper, S. X. Dou, X. G. Liu and D. Y. Jin, Nat. Commun., 2016, 7, 10254 CrossRef CAS PubMed.
- S. Han, A. Samanta, X. J. Xie, L. Huang, J. J. Peng, S. J. Park, D. B. L. Teh, Y. Choi, Y. T. Chang, A. H. All, Y. M. Yang, B. G. Xing and X. G. Liu, Adv. Mater., 2017, 29, 1700244 CrossRef PubMed.
- Q. S. Chen, X. J. Xie, B. L. Huang, L. L. Liang, S. Y. Han, Z. G. Yi, Y. Wang, Y. Li, D. Y. Fan, L. Huang and X. G. Liu, Angew. Chem., Int. Ed., 2017, 56, 7605–7609 CrossRef CAS PubMed.
- S. Chen, A. Z. Weitemier, X. Zeng, L. M. He, X. Y. Wang, Y. Q. Tao, A. J. Y. Huang, Y. Hashimotodani, M. Kano, H. Iwasaki, L. K. Parajuli, S. Okabe, D. B. L. Teh, A. H. All, I. Tsutsui-Kimura, K. F. Tanaka, X. G. Liu and T. J. McHugh, Science, 2018, 359, 679–683 CrossRef CAS PubMed.
- S. Shi, L. D. Sun, Y. X. Xue, H. Dong, K. Wu, S. C. Guo, B. T. Wu and C. H. Yan, Nano Lett., 2018, 18, 2964–2969 CrossRef CAS PubMed.
- G. A. Mandl, P. A. Rojas-Gutierrez and J. A. Capobianco, Chem. Commun., 2018, 54, 5847–5850 RSC.
- L. Sun, R. Wei, J. Feng and H. Zhang, Coord. Chem. Rev., 2018, 364, 10–32 CrossRef CAS.
- S. S. Lucky, N. M. Idris, Z. Q. Li, K. Huang, K. C. Soo and Y. Zhang, ACS Nano, 2015, 9, 191–205 CrossRef CAS PubMed.
- M. K. Tsang, W. W. Ye, G. J. Wang, J. M. Li, M. Yang and J. H. Hao, ACS Nano, 2016, 10, 598–605 CrossRef CAS PubMed.
- C. D. S. Brites, X. J. Xie, M. L. Debasu, X. Qin, R. F. Chen, W. Huang, J. Rocha, X. G. Liu and L. D. Carlos, Nat. Nanotechnol., 2016, 11, 851–856 CrossRef CAS PubMed.
- J. Liu, G. Y. Chen, S. W. Hao and C. H. Yang, Nanoscale, 2017, 9, 91–98 RSC.
- Q. L. Zou, P. Huang, W. Zheng, W. W. You, R. F. Li, D. T. Tu, J. Xu and X. Y. Chen, Nanoscale, 2017, 9, 6521–6528 RSC.
- H. Fu, P. Peng, R. Li, C. Liu, Y. Liu, F. Jiang, M. Hong and X. Chen, Nanoscale, 2018, 10, 9353–9359 RSC.
- O. A. Savchuk, J. J. Carvajal, C. D. S. Brites, L. D. Carlos, M. Aguilo and F. Diaz, Nanoscale, 2018, 10, 6602–6610 RSC.
- L. Wang, C. Gao, K. Y. Liu, Y. X. Liu, L. Y. Ma, L. D. Liu, X. X. Du and J. Zhou, Adv. Funct. Mater., 2016, 26, 3480–3489 CrossRef CAS.
- M. Quintanilla, F. Q. Ren, D. L. Ma and F. Vetrone, ACS Photonics, 2014, 1, 662–669 CrossRef CAS.
- S. Som, S. Das, C. Y. Yang and C. H. Lu, Opt. Lett., 2016, 41, 464–467 CrossRef CAS PubMed.
- P. P. Lei, R. An, S. Yao, Q. S. Wang, L. L. Dong, X. Xu, K. M. Du, J. Feng and H. J. Zhang, Adv. Mater., 2017, 29, 1700505 CrossRef PubMed.
- F. Guzzetta, A. Roig and B. Julian-Lopez, J. Phys. Chem. Lett., 2017, 8, 5730–5735 CrossRef CAS PubMed.
- H. Dong, L. D. Sun and C. H. Yan, Chem. Soc. Rev., 2015, 44, 1608–1634 RSC.
- N. J. J. Johnson, S. He, S. Diao, E. M. Chan, H. J. Dai and A. Almutairi, J. Am. Chem. Soc., 2017, 139, 3275–3282 CrossRef CAS PubMed.
- Y. Wang, K. Zheng, S. Song, D. Fan, H. Zhang and X. Liu, Chem. Soc. Rev., 2018, 47, 6473–6485 RSC.
- S. H. Wen, J. J. Zhou, K. Z. Zheng, A. Bednarkiewicz, X. G. Liu and D. Y. Jin, Nat. Commun., 2018, 9, 2415 CrossRef PubMed.
- M. S. Meijer, P. A. Rojas-Gutierrez, D. Busko, I. A. Howard, F. Frenzel, C. Wurth, U. Resch-Genger, B. S. Richards, A. Turshatov, J. A. Capobianco and S. Bonnet, Phys. Chem. Chem. Phys., 2018, 20, 22556–22562 RSC.
- M. D. Wisser, S. Fischer, P. C. Maurer, N. D. Bronstein, S. Chu, A. P. Alivisatos, A. Salleo and J. A. Dionne, ACS Photonics, 2016, 3, 1523–1530 CrossRef CAS.
- R. R. Deng, J. Wang, R. F. Chen, W. Huang and X. G. Liu, J. Am. Chem. Soc., 2016, 138, 15972–15979 CrossRef CAS PubMed.
- L. M. Jin, X. Chen, C. K. Siu, F. Wang and S. F. Yu, ACS Nano, 2017, 11, 843–849 CrossRef CAS PubMed.
- C. Homann, L. Krukewitt, F. Frenzel, B. Grauel, C. Wurth, U. Resch-Genger and M. Haase, Angew. Chem., Int. Ed., 2018, 57, 8765–8769 CrossRef CAS PubMed.
- A. Lay, C. Siefe, S. Fischer, R. D. Mehlenbacher, F. Ke, W. L. Mao, A. P. Aliyisatos, M. B. Goodman and J. A. Dionne, Nano Lett., 2018, 18, 4454–4459 CrossRef CAS PubMed.
- J. J. Peng, A. Samanta, X. Zeng, S. Y. Han, L. Wang, D. D. Su, D. T. B. Loong, N. Y. Kang, S. J. Park, A. H. All, W. X. Jiang, L. Yuan, X. G. Liu and Y. T. Chang, Angew. Chem., Int. Ed., 2017, 56, 4165–4169 CrossRef CAS PubMed.
- B. Chen, Y. Liu, Y. Xiao, X. Chen, Y. Li, M. Y. Li, X. S. Qiao, X. P. Fan and F. Wang, J. Phys. Chem. Lett., 2016, 7, 4916–4921 CrossRef CAS PubMed.
- C. Yao, P. Y. Wang, X. M. Li, X. Y. Hu, J. L. Hou, L. Y. Wang and F. Zhang, Adv. Mater., 2016, 28, 9341–9348 CrossRef CAS PubMed.
- P. Y. Wang, Y. Fan, L. F. Lu, L. Liu, L. L. Fan, M. Y. Zhao, Y. Xie, C. J. Xu and F. Zhang, Nat. Commun., 2018, 9, 2898 CrossRef PubMed.
- C. Wurth, S. Fischer, B. Grauel, A. P. Alivisatos and U. Resch-Genger, J. Am. Chem. Soc., 2018, 140, 4922–4928 CrossRef PubMed.
- S. Fischer, N. D. Bronstein, J. K. Swabeck, E. M. Chan and A. P. Alivisatos, Nano Lett., 2016, 16, 7241–7247 CrossRef CAS PubMed.
- M. D. Wisser, S. Fischer, C. Siefe, A. P. Alivisatos, A. Salleo and J. A. Dionne, Nano Lett., 2018, 18, 2689–2695 CrossRef CAS PubMed.
- F. Vetrone, R. Naccache, V. Mahalingam, C. G. Morgan and J. A. Capobianco, Adv. Funct. Mater., 2009, 19, 2924–2929 CrossRef CAS.
- B. Liu, Y. Y. Chen, C. X. Li, F. He, Z. Y. Hou, S. S. Huang, H. M. Zhu, X. Y. Chen and J. Lin, Adv. Funct. Mater., 2015, 25, 4717–4729 CrossRef CAS.
- B. Zhou, W. F. Yang, S. Y. Han, Q. Sun and X. G. Liu, Adv. Mater., 2015, 27, 6208–6212 CrossRef CAS PubMed.
- K. Z. Zheng, S. Y. Han, X. Zeng, Y. M. Wu, S. Y. Song, H. J. Zhang and X. G. Liu, Adv. Mater., 2018, 30, 1801726 CrossRef PubMed.
- K. Prorok, M. Pawlyta, W. Strek and A. Bednarkiewicz, Chem. Mater., 2016, 28, 2295–2300 CrossRef CAS.
- S. W. Hao, G. Y. Chen, C. H. Yang, W. Shao, W. Wei, Y. Liu and P. N. Prasad, Nanoscale, 2017, 9, 10633–10638 RSC.
- X. M. Li, Z. Z. Guo, T. C. Zhao, Y. Lu, L. Zhou, D. Y. Zhao and F. Zhang, Angew. Chem., Int. Ed., 2016, 55, 2464–2469 CrossRef CAS PubMed.
- D. L. Zhou, D. L. Liu, W. Xu, Z. Yin, X. Chen, P. W. Zhou, S. B. Cui, Z. G. Chen and H. W. Song, ACS Nano, 2016, 10, 5169–5179 CrossRef CAS PubMed.
- K. Park, K. Jung, S. J. Kwon, H. S. Jang, D. Byun, I. K. Han and H. Ko, Adv. Funct. Mater., 2016, 26, 7836–7846 CrossRef CAS.
- M. S. Shariatdoust, A. L. Frencken, A. Khademi, A. Alizadehkhaledi, F. C. J. M. van Veggel and R. Gordon, ACS Photonics, 2018, 5, 3507–3512 CrossRef CAS.
- Z. H. Xu, M. Quintanilla, F. Vetrone, A. O. Govorov, M. Chaker and D. L. Ma, Adv. Funct. Mater., 2015, 25, 2950–2960 CrossRef CAS.
- F. W. Kang, J. J. He, T. Y. Sun, Z. Y. Bao, F. Wang and D. Y. Lei, Adv. Funct. Mater., 2017, 27, 1701842 CrossRef.
- K. Park, M. Park, H. S. Jang, J. H. Park, J. Kim, Y. Cho, I. K. Han, D. Byun and H. Ko, Adv. Funct. Mater., 2018, 28, 1800369 CrossRef.
- D. Li, S. H. Yu and H. L. Jiang, Adv. Mater., 2018, 30, 1707377 CrossRef PubMed.
- W. Shao, G. Y. Chen, A. Kuzmin, H. L. Kutscher, A. Pliss, T. Y. Ohulchanskyy and P. N. Prasad, J. Am. Chem. Soc., 2016, 138, 16192–16195 CrossRef CAS PubMed.
- W. Wei, G. Y. Chen, A. Baev, G. S. He, W. Shao, J. Damasco and P. N. Prasad, J. Am. Chem. Soc., 2016, 138, 15130–15133 CrossRef CAS PubMed.
- X. D. Wang, R. R. Valiev, T. Y. Ohulchanskyy, H. Agren, C. H. Yang and G. Y. Chen, Chem. Soc. Rev., 2017, 46, 4150–4167 RSC.
- G. Y. Chen, J. Damasco, H. L. Qiu, W. Shao, T. Y. Ohulchanskyy, R. R. Valiev, X. Wu, G. Han, Y. Wang, C. H. Yang, H. Agren and P. N. Prasad, Nano Lett., 2015, 15, 7400–7407 CrossRef CAS PubMed.
- J. T. Xu, P. P. Yang, M. D. Sun, H. T. Bi, B. Liu, D. Yang, S. L. Gai, F. He and J. Lin, ACS Nano, 2017, 11, 4133–4144 CrossRef CAS PubMed.
- J. T. Xu, A. Gulzar, Y. H. Liu, H. T. Bi, S. L. Gai, B. Liu, D. Yang, F. He and P. P. Yang, Small, 2017, 13, 1701841 CrossRef PubMed.
- X. Wu, H. Lee, O. Bilsel, Y. W. Zhang, Z. J. Li, T. Chen, Y. Liu, C. Y. Duan, J. Shen, A. Punjabi and G. Han, Nanoscale, 2015, 7, 18424–18428 RSC.
- J. Lee, B. Yoo, H. Lee, G. D. Cha, H. S. Lee, Y. Cho, S. Y. Kim, H. Seo, W. Lee, D. Son, M. Kang, H. M. Kim, Y. I. Park, T. Hyeon and D. H. Kim, Adv. Mater., 2017, 29, 1603169 CrossRef PubMed.
- C. D. LaBoda and C. L. Dwyer, Adv. Funct. Mater., 2016, 26, 2866–2874 CrossRef CAS.
- X. M. Zou, M. Xu, W. Yuan, Q. H. Wang, Y. B. Shi, W. Feng and F. Y. Li, Chem. Commun., 2016, 52, 13389–13392 RSC.
- W. J. Wang, Y. C. Li, Z. W. Kang, F. Wang and J. C. Yu, Appl. Catal., B, 2016, 182, 184–192 CrossRef CAS.
- Z. L. Qiu, J. Shu and D. P. Tang, Anal. Chem., 2018, 90, 1021–1028 CrossRef CAS PubMed.
- Q. Z. Zhang, J. J. Deng, Z. H. Xu, M. Chaker and D. L. Ma, ACS Catal., 2017, 7, 6225–6234 CrossRef CAS.
- F. Zhang, C. L. Zhang, W. N. Wang, H. P. Cong and H. S. Qian, ChemSusChem, 2016, 9, 1449–1454 CrossRef CAS PubMed.
- M. J. Tou, Y. Y. Mei, S. Bai, Z. G. Luo, Y. Zhang and Z. Q. Li, Nanoscale, 2016, 8, 553–562 RSC.
- W. H. Feng, L. L. Zhang, Y. Zhang, Y. Yang, Z. B. Fang, B. Wang, S. Y. Zhang and P. Liu, J. Mater. Chem. A, 2017, 5, 10311–10320 RSC.
- S. Ganguli, C. Hazra, M. Chatti, T. Samanta and V. Mahalingam, Langmuir, 2016, 32, 247–253 CrossRef CAS PubMed.
- L. J. Bai, W. Y. Jiang, C. X. Gao, S. X. Zhong, L. H. Zhao, Z. Q. Li and S. Bai, Nanoscale, 2016, 8, 19014–19024 RSC.
- X. F. Li, H. Ren, Z. J. Zou, J. J. Sun, J. Y. Wang and Z. H. Liu, Chem. Commun., 2016, 52, 453–456 RSC.
- Y. W. Yang, W. X. Que, X. Y. Zhang, X. T. Yin, Y. L. Xing, M. D. Que, H. Y. Zhao and Y. P. Du, Appl. Catal., B, 2017, 200, 402–411 CrossRef CAS.
- Z. B. Wu, X. Z. Yuan, G. M. Zeng, L. B. Jiang, H. Zhong, Y. C. Xie, H. Wang, X. H. Chen and H. Wang, Appl. Catal., B, 2018, 225, 8–21 CrossRef CAS.
- H. Jia, C. Ping, C. Xu, J. J. Zhou, X. W. Sang, J. C. Wang, C. Liu, X. F. Liu and J. R. Qiu, J. Mater. Chem. A, 2015, 3, 5917–5922 RSC.
- D. Wang, L. Zhu, J. F. Chen and L. M. Dai, Angew. Chem., Int. Ed., 2016, 55, 10795–10799 CrossRef CAS PubMed.
- Q. Y. Tian, W. J. Yao, Z. H. Wu, J. Liu, L. Liu, W. Wu and C. Z. Jiang, J. Mater. Chem. A, 2017, 5, 23566–23576 RSC.
- J. M. Zhang, Y. Huang, L. Jin, F. Rosei, F. Vetrone and J. P. Claverie, ACS Appl. Mater. Interfaces, 2017, 9, 8142–8150 CrossRef CAS PubMed.
- S. P. Sahu, S. L. Cates, H. I. Kim, J. H. Kim and E. L. Cates, Environ. Sci. Technol., 2018, 52, 2973–2980 CrossRef CAS PubMed.
- S. Q. Huang, H. M. Wang, N. W. Zhu, Z. Y. Lou, L. Li, A. D. Shan and H. P. Yuan, Appl. Catal., B, 2016, 181, 456–464 CrossRef CAS.
- W. N. Wang, C. X. Huang, C. Y. Zhang, M. L. Zhao, J. Zhang, H. J. Chen, Z. B. Zha, T. T. Zhao and H. S. Qian, Appl. Catal., B, 2018, 224, 854–862 CrossRef CAS.
- C. Cui, M. J. Tou, M. H. Li, Z. G. Luo, L. B. Xiao, S. Bai and Z. Q. Li, Inorg. Chem., 2017, 56, 2328–2336 CrossRef CAS PubMed.
- C. Yao, W. X. Wang, P. Y. Wang, M. Y. Zhao, X. M. Li and F. Zhang, Adv. Mater., 2018, 30, 1704833 CrossRef PubMed.
- Q. Y. Tian, W. J. Yao, W. Wu, J. Liu, Z. H. Wu, L. Liu, Z. G. Dai and C. Z. Jiang, ACS Sustainable Chem. Eng., 2017, 5, 10889–10899 CrossRef CAS.
- S. S. Dong, S. S. Dong, X. D. Tian, Z. X. Xu, D. M. Ma, B. Cui, N. Q. Ren and B. E. Rittmann, J. Hazard. Mater., 2016, 302, 386–394 CrossRef CAS PubMed.
- W. Gao, W. Y. Zhang and G. X. Lu, Appl. Catal., B, 2017, 212, 23–31 CrossRef CAS.
- W. Gao, W. Y. Zhang, B. Tian, W. L. Zhen, Y. Q. Wu, X. Q. Zhang and G. X. Lu, Appl. Catal., B, 2018, 224, 553–562 CrossRef CAS.
|
This journal is © The Royal Society of Chemistry 2019 |
Click here to see how this site uses Cookies. View our privacy policy here.