A multiple target chemosensor for the sequential fluorescence detection of Zn2+ and S2− and the colorimetric detection of Fe3+/2+ in aqueous media and living cells†
Received
17th September 2018
, Accepted 15th October 2018
First published on 15th October 2018
Abstract
A novel multiple target sensor, (E)-5-((4-(diethylamino)-2-hydroxybenzyldene)amino)-1H-imidazole-4-carboxamide (DHIC), was synthesized for fluorescence detection of Zn2+ and S2− and colorimetric detection of Fe3+/2+ in aqueous media. DHIC can operate as a turn “on–off” sequential fluorescent sensor for Zn2+ and S2−. Detection limits (1.59 μM and 8.03 μM) for Zn2+ and S2− are below the WHO standards (76.0 μM and 14.7 μM). The DHIC–Zn2+ complex could be reversibly reused with ethylenediaminetetraacetic acid. Importantly, DHIC could image sequentially Zn2+ and S2− in living cells. Moreover, DHIC displayed a discriminatory color change from pale yellow to orange yellow to Fe3+/2+. The detection limit of DHIC for Fe3+/2+ (0.73 μM and 1.11 μM) is far below the EPA drinking water standard (5.37 μM). The sensor DHIC could be applied to analyze Fe3+ in real samples.
1. Introduction
Zinc is one of the transition metal ions present in the body and has various roles in many physiological and biological processes like cell apoptosis, signal transduction, cellular metabolism, and DNA synthesis.1–4 However, imbalance of Zn2+ in the human body can lead to fatal, serious diseases, including epilepsy, Alzheimer's disease, ischemic stroke, Parkinson's disease, and some types of carcinoma.5–7 To control the Zn level in biological systems, therefore, fluorogenic chemosensors have been developed.8 Although many Zn2+ chemosensors have been reported, it's still a difficult task to discriminate Zn2+ and Cd2+,9–11 since these elements have analogous photo-physical properties.12,13 For these reasons, the development of easily synthesized, highly selective and sensitive zinc chemosensors has attracted considerable attention in biological and chemical areas.14–18
Hydrogen sulfide (H2S), the simplest biothiol, is generated by enzymes like mercaptopyruvate sulfurtransferase, cystathionine-β-synthase, and cysteine lyase.19–22 H2S belongs to the family of endogenous gasotransmitters such as CO and NO.23–27 It contributes to a broad range of physiological processes, like oxygen sensing, reducing blood pressure, vasodilation, anti-inflammation and apoptosis.28–31 However, excess of H2S in cells causes various diseases like Alzheimer's disease, liver cirrhosis and Menkes disease.28,32–36
Iron performs pivotal roles in many biological systems, including electron transfer, oxygen storage and oxygen carrier function in hemoglobin.37–41 Its deficiency or superabundance not only causes some dysfunction of organs like the kidney, heart, and liver, but also leads to several diseases like anemia and diabetes.42–44 Thus, the development of tools to analyze iron in biological and environmental fields has been of great interest.45–48
Schiff bases that have donor atoms such as N and O form strong complexes with transition metal ions.49–51 Thus, Schiff bases have often been used as chemosensors for detecting metal ions. The imidazole moiety with fluorogenic properties could be used as a part of a metal-ion sensor,42,45,52 and the 4-diethylaminosalicylaldehyde moiety is also water-soluble and fluorogenic.53,54 Thus, we designed and synthesized a novel Schiff base sensor, DHIC, on the basis of the condensation reaction of the imidazole and 4-diethylaminosalicylaldehude moieties; it is water-soluble and has the potential to detect multiple analytes. While many chemosensors for single analytes have been reported, single chemosensors targeting multiple analytes have been relatively less developed.55–58 Since multi-functional chemosensors are cost-effective and convenient compared to single-response chemosensors, it is of great importance to develop multi-functional chemosensors.59,60
Herein, we present a multiple target chemosensor, DHIC, (E)-5-((4-(diethylamino)-2-hydroxybenzyldene)amino)-1H-imidazole-4-carboxamide, which showed consecutive fluorescence turn “on–off” for Zn2+ and S2−. In addition, it could image consecutively Zn2+ and S2− in living cells. The sensor DHIC could also detect Fe3+/2+ by a change of color from pale yellow to orange yellow in a near-perfect aqueous medium (Bis–Tris buffer
:
DMF = 997
:
3). Importantly, among the chemosensors reported to date, DHIC is the first chemosensor that can recognize the four analytes, Zn2+, S2−, and Fe3+/2+.
2. Experiment
Materials and instrumentation
Chemical reagents were obtained commercially. ESI-MS and NMR data were collected using a Thermo Finnigan ion trap machine and a Varian spectrometer. Spectral data of emission and absorption were obtained using spectrometers (LS45 and Lambda 2S UV/Vis) from PerkinElmer.
Synthesis of the receptor DHIC ((E)-5-((4-(diethylamino)-2-hydroxybenzyldene)amino)-1H-imidazole-4-carboxamide)
Triethylamine (1.2 mmol, 169 μL) and 5(4)-amino-4(5)-(aminocarbonyl)imidazole hydrochloride (1.0 mmol, 0.17 g) were dissolved in 15.0 mL of MeOH and stirred for 1.5 h. 4-Diethylaminosalicylaldehyde (1.0 mmol, 0.20 g) and a few drops of H3PO4 were added to the resulting solution, which was blended for an additional 7 h. A yellow powder was produced, which was gathered by filtration. Then, the yellow solid was washed twice with ether and dried under vacuum (0.19 g, 65.0%). 1H NMR (400 MHz, DMSO-d6): δ = 12.63 (s, 1H, NH, imidazole), 11.53 (s, 1H, OH), 9.12 (s, NCH, imine), 7.61 (s, 1H, CHNH), 7.51 (d, J = 8 Hz, 1H, m-phenyl), 7.41 (s, 2H, amide), 6.30 (d, J = 8 Hz, p-phenyl), 6.09 (s, o-phenyl), 3.36 (m, 4H, CH2CH3, ethyl), 2.41 (t, J = 12 Hz, 6H, CH3CH2, methyl); 13C NMR (100 MHz, DMSO-d6): 162.07 (1C), 161.65 (1C), 157.65 (1C), 152.02 (1C), 148.16 (1C), 135.96 (1C), 132.10 (1C), 116.24 (1C), 109.68 (1C), 104.74 (1C), 97.28 (1C), 44.39 (2C), 13.00 (2C); Low Resolution Mass Spectrometry (LRMS) (ESI): m/z calcd for C15H19N5O2 + H+: 302.16; found: 302.10.
Fluorescence detection
Fluorescence and UV-visible titrations of DHIC with Zn2+.
DMF (1.0 mL) was employed to dissolve the compound DHIC (0.9 mg, 3 × 10−3 mmol). 10.0 μL (3.0 mM) of DHIC was diluted with 2.99 mL of Bis–Tris buffer solution (10 mM, pH 7.0) to 10.0 μM. Bis–Tris buffer (5.0 mL) was employed to dissolve Zn(NO3)2 (0.1 mmol, 30.4 mg). 0–33 μL (2 × 10−2 M) of the Zn2+ solution were added to each diluted DHIC (3.0 mL, 10.0 μM). Fluorescence measurements were executed after mixing them. For UV-vis titration, 0–21 μL (20.0 mM) of the Zn2+ solution were added to the DHIC (3.0 mL, 10.0 μM) prepared above. UV-vis measurements were executed after mixing them.
Job plot of DHIC with Zn2+.
DMF (1.0 mL) was employed to dissolve the compound DHIC (3.01 mg, 1 × 10−2 mmol). 0–12.0 μL of DHIC was added to fluorescence cells. They were diluted with Bis–Tris buffer for preparing a total volume of 2.988 mL. Bis–Tris buffer (1.0 mL) was employed to dissolve Zn(NO3)2 (3.0 mg, 0.01 mmol). 0–12.0 μL of the zinc solution were added to each diluted DHIC. The fluorescence cell contained a total volume of 3 mL. Fluorescence measurements were executed after mixing them.
Competition test.
DMF (1.0 mL) was employed to dissolve the compound DHIC (0.9 mg, 3 × 10−3 mmol). 10.0 μL (3.0 mM) of DHIC was diluted with 2.990 mL of Bis–Tris buffer to 10 μM. Bis–Tris buffer (5.0 mL) was employed to dissolve 1 × 10−1 mmol of KNO3 or Fe(ClO4)2 or NaNO3 or M(NO3)3 (M = Cr, Fe and Al) or M(NO3)2 (M = Pb, Zn, Cd, Mg, Co, Cu, Mn, Ni, and Ca). 33 μL (2 × 10−2 M) of each cation solution was added to 3.0 mL each of DHIC (10 μM) to produce 21 equiv. 33.0 μL of Zn(NO3)2 was added to the mixture of DHIC and each cation solution to produce 21 equiv. Fluorescence measurements were executed after mixing them.
pH effect.
By mixing HCl with Bis–Tris buffer and NaOH solution, buffers having different pH values (2–12) were made. With the desired pH solutions, DHIC (0.9 mg, 3 × 10−3 mmol) was dissolved in 1.0 mL of DMF, and 10.0 μL (3.0 mM) of DHIC was diluted with 2.99 mL Bis–Tris buffer to 10 μM. Bis–Tris buffer (5.0 mL) was employed to dissolve Zn(NO3)2 (30.4 mg, 10−1 mmol). 33.0 μL (2 × 10−2 M) of Zn2+ was transferred to each DHIC (10.0 μM). Fluorescence measurements were executed after mixing them.
NMR titration of DHIC with Zn2+.
DMSO-d6 (700 μL) was employed to dissolve DHIC (0.1 mmol, 3.0 mg) in three NMR tubes, respectively. Three different equiv. (1, 0.5, and 0 equiv.) of Zn(NO3)2 dissolved in DMSO-d6 (300 μL) were transferred to DHIC. 1H NMR measurements were executed after mixing them.
Fluorescence and UV-vis titrations of DHIC–Zn2+ with S2−.
DMF (1.0 mL) was employed to dissolve DHIC (0.9 mg, 3 × 10−3 mmol). 10.0 μL of DHIC (3.0 mM) was diluted with 2.99 mL of Bis–Tris buffer to 10.0 μM. Bis–Tris buffer (5.0 mL) was employed to dissolve Zn(NO3)2 (0.1 mmol, 30.0 mg). 21.0 μL (20.0 mM) of Zn2+ solution was added to the DHIC solution (3.0 mL, 10.0 μM). Bis–Tris buffer (5.0 mL) was employed to dissolve Na2S·9H2O (5 × 10−1 mmol, 130 mg). 0–11.4 μL (0.1 M) of S2− was added to the complex solution. Fluorescence measurements were executed after mixing them. For UV-vis titration, 0–8.1 μL (0.1 M) of S2− was added to the complex solution. UV-vis measurements were executed after mixing them.
Job plot of DHIC–Zn2+ with S2−.
DMF (1.0 mL) was employed to dissolve DHIC (6.0 mg, 2 × 10−2 mmol) and Zn(NO3)2 (6.1 mg, 2 × 10−2 mmol), respectively. Two solutions were admixed to prepare the DHIC–Zn2+ complex. 0–30.0 μL of DHIC–Zn2+ were transferred to fluorescence cells. They were diluted with Bis–Tris buffer for preparing a total volume of 2.985 mL. Bis–Tris buffer (1.0 mL) was employed to dissolve Na2S·9H2O (4.8 mg, 2 × 10−2 mmol). 0–15.0 μL of S2− was added to diluted DHIC–Zn2+. The fluorescence cell contained a total volume of 3 mL. Fluorescence measurements were executed after mixing them.
Competition test.
DMF (1.0 mL) was employed to dissolve DHIC (0.9 mg, 3 × 10−3 mmol). 10 μL (3.0 mM) of DHIC was diluted with 2.990 mL of Bis–Tris buffer to 10 μM. Bis–Tris buffer (5.0 mL) was employed to dissolve Zn(NO3)2 (0.1 mmol, 30.0 mg). 21.0 μL (20.0 mM) of Zn2+ was added to DHIC solution (3.0 mL, 10.0 μM). 0.5 mmol of Na2S·9H2O, TBAX (tetrabutylammonium salts) (X = N3−, H2PO4−, OAc−, BzO−, SCN− and CN−), NaNO2, and TEAX (tetraethylammonium salts) (X = I−, F−, Br− and Cl−) was dissolved in Bis–Tris buffer (5.0 mL). 10.2 μL (0.1 M) of each anion solution was added to 3.0 mL of each DHIC–Zn2+ (10.0 μM) to produce 34 equiv. 10.2 μL (0.1 M) of Na2S was added to the mixture of DHIC–Zn2+ and each anion solution to produce 34 equiv. Fluorescence measurements were executed after mixing them.
pH effect on the DHIC–Zn2+ complex with S2−.
By mixing HCl with Bis–Tris buffer and NaOH solution, buffers having different pH values (2–12) were made. With the desired pH solutions, DHIC (0.9 mg, 3 × 10−3 mmol) was dissolved in DMF (1.0 mL), and 10.0 μL (3.0 mM) of the DHIC was diluted with 2.99 mL Bis–Tris buffer to give 10 μM. Bis–Tris buffer (5.0 mL) was employed to dissolve Zn(NO3)2 (0.1 mmol, 30.0 mg). 21.0 μL (20.0 mM) of Zn2+ was added to DHIC (10.0 μM, 3.0 mL). Bis–Tris buffer (5.0 mL) was employed to dissolve Na2S·9H2O (0.5 mmol, 130 mg). 10.2 μL (0.1 M) of S2− solution was transferred to each DHIC–Zn2+ (10.0 μM). Fluorescence measurements were executed after mixing them.
Imaging in living cells.
HeLa cells were maintained in media having 0.1 g mL−1 streptomycin, fetal bovine serum (10%), 102 U mL−1 penicillin, and Dulbecco's modified Eagle's medium. The cells were bred under a humidified atmosphere containing 5% CO2 at 37 °C. Cells were bred on a 12 well plate at a density of 104 cells per 0.1 mL and then incubated at 37 °C for 19 h. Cells were treated with DHIC (2 × 10−2 M) for 1 h before an additional incubation with Zn(NO3)2 (pH 7.43, 0.15 M NaCl; 600 μM) for 1 h. For the fluorescence quenching experiment, cells were treated with DHIC (20 μM) and Zn(NO3)2 (pH 7.43, 0.15 M NaCl; 600 μM) for 1 h each. Na2S (pH 7.43, 0.15 M NaCl; 400 μM) was treated with the cells for 1 h. An EVOS FL fluorescence microscope was applied for imaging (λex = 470 nm and λem = 510 nm).
MTT assay.
HeLa cells grew and were maintained at 37 °C under a humidified atmosphere with 5% CO2. Cell viability upon treatment with DHIC was determined by the MTT assay. Cells were seeded in a 96 well plate (2000 cells for 1 h incubation and 2000 cells for 24 h incubation in 100 μL per well). The cells were treated with DHIC at two concentrations (10 and 20 μM; 1% v/v final DMSO concentration). After 1 h or 24 h incubation, MTT [25 μL of 5 mg mL−1 in PBS (pH 7.4, GIBCO)] was added to each well and the plate was incubated for 4 h at 37 °C. Formazan produced by cells was solubilized using an acidic solution of dimethylformamide (DMF; pH 4.5, 50% v/v, aq.) and sodium dodecyl sulfate (SDS; 20% w/v) overnight at room temperature in the dark. The absorbance was measured at 600 nm by using a microplate reader. Cell viability was calculated relative to cells containing an equivalent amount of DMSO. The measurements were conducted in triplicate.
Colorimetric detection
UV-vis titrations of DHIC with Fe3+/2+.
DMF (1.0 mL) was employed to dissolve DHIC (0.9 mg, 3 × 10−3 mmol). 20 μL of DHIC (3 mM) was diluted with 2.98 mL Bis–Tris buffer (10 mM, pH 7.0) to 20 μM. Bis–Tris buffer (5.0 mL) was employed to dissolve Fe(NO3)3 (or Fe(ClO4)2) (0.1 mmol). 0–3.3 μL (2 × 10−2 M) of the Fe3+ solution (or 0–4.2 μL of the Fe2+) were transferred to DHIC (20.0 μM, 3.0 mL). UV-vis measurements were executed after mixing them.
Job plot measurements.
DMF (1.0 mL) was employed to dissolve DHIC (3.01 mg, 10−2 mmol). 6.0, 5.4, 4.8, 4.2, 3.6, 3.0, 2.4, 1.8, 1.2, 0.6 and 0 μL of DHIC were transferred to UV-visible cells. They were diluted with Bis–Tris buffer to produce a total volume of 2.994 mL. Bis–Tris buffer (1.0 mL) was employed to dissolve Fe(NO3)3 (or Fe(ClO4)2) (0.01 mmol). 0, 0.6, 1.2, 1.8, 2.4, 3.0, 3.6, 4.2, 4.8, 5.4 and 6.0 μL of iron were added to dilute DHIC. The UV-visible cell had a total volume of 3 mL. UV-vis measurements were executed after mixing them.
Competition test.
DMF (1.0 mL) was employed to dissolve DHIC (0.9 mg, 3 × 10−3 mmol). 20.0 μL (3.0 mM) of DHIC was diluted with 2.98 mL of buffer to 20 μM. Bis–Tris buffer (5.0 mL) was employed to dissolve 0.1 mmol of KNO3 or M(NO3)2 (M = Zn, Cd, Pb, Mg, Ca, Ni, Co, Mn and Cu) or Fe(ClO4)2 or NaNO3 or M(NO3)3 (M = Cr, Fe and Al), respectively. 3.9 μL (2 × 10−2 M) of each cation was added to 3.0 mL of DHIC (10 μM) to produce 1.3 equiv. 3.0 μL (2 × 10−2 M) of Fe(NO3)3 (or 3.9 μL of the Fe(ClO4)2) was added to the mixture of DHIC and each cation solution to produce 1.3 equiv. (or 1.0 equiv.). UV-vis measurements were executed after mixing them.
pH effect.
By mixing HCl with Bis–Tris buffer and NaOH solution, buffers having different pH values (2–12) were made. With the desired pH solutions, DHIC (0.9 mg, 3 × 10−3 mmol) was dissolved in DMF (1.0 mL), and 20.0 μL (3.0 mM) of DHIC was diluted with 2.98 mL Bis–Tris buffer to 20 μM. Bis–Tris buffer (5.0 mL) was employed to dissolve Fe(NO3)3 (or Fe(ClO4)2) (0.01 mmol). 3.0 μL (2 × 10−2 M) of Fe3+ solution or 3.9 μL of Fe2+ was transferred to each DHIC solution (20 μM). UV-vis measurements were executed after mixing them.
3. Results and discussion
The multiple target compound DHIC was produced by reaction of 5(4)-amino-4(5)-(aminocarbonyl)imidazole hydrochloride and 4-diethylaminosalicylaldehyde in MeOH (Scheme 1), and analyzed by 1H NMR, 13C NMR, and ESI-mass spectrometry.
 |
| Scheme 1 Synthesis procedure of DHIC. | |
Fluorescence sensing of DHIC for Zn2+
The fluorescence sensing capability of DHIC was checked with different metal ions (Mn2+, Cd2+, Ni2+, Fe3+, Pb2+, Cu2+, Fe2+, Co2+, Cr3+, Ca2+, Mg2+, Na+, Zn2+, Al3+ and K+) in Bis–Tris buffer (Fig. 1). The sensor DHIC showed a little fluorescence peak at 484 nm when excited at 420 nm. On the addition of 21 equiv. of the cations to DHIC, only zinc showed immediately a considerable fluorescence intensity change. Quantum yields (Φ) of 0.0041 and 0.0411 were calculated for DHIC and the DHIC–Zn2+ complex. These outcomes implied that the compound DHIC could operate as a “turn-on” fluorescent probe for Zn2+ and also definitely differentiate Zn2+ and Cd2+.
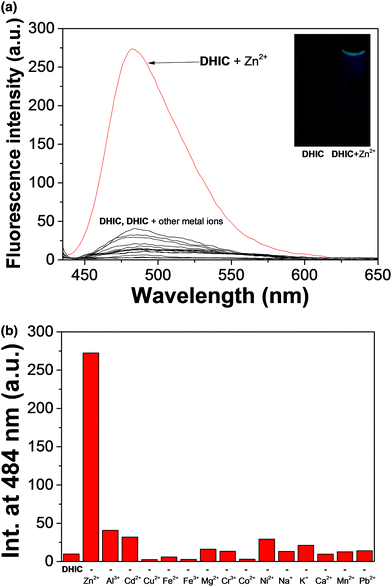 |
| Fig. 1 (a) Fluorescence selectivity of DHIC (10.0 μM) with different cations (21 equiv.) with excitation at 420 nm. (b) Bar graph displaying the relative emission of DHIC at 484 nm upon treatment with different cations. | |
Fluorescence titration was executed to examine the spectroscopic properties of DHIC for Zn2+ (Fig. 2). With the addition of Zn2+, fluorescence emission at 484 nm continuously increased up to 21 equiv. of Zn2+. The binding properties were also inspected by UV-vis titration experiment (Fig. S1†). The absorbance at 410 nm decreased, and the bands at 270 and 460 nm increased with an isosbestic point (345 nm), which means the production of a species upon treatment of DHIC with Zn2+.
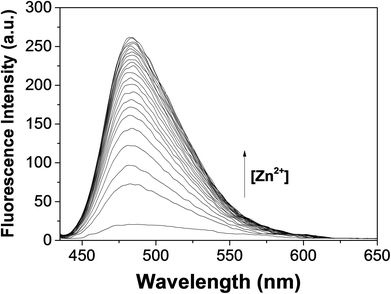 |
| Fig. 2 Fluorescence titration of DHIC (10 μM) with different Zn2+ concentrations. | |
The 1
:
1 interaction ratio between DHIC and Zn2+ was obtained by Job plot analysis (Fig. S2†), which was affirmed by ESI mass spectrometry (Fig. S3†). A peak at m/z = 364.10 was indicative of [DHIC − H+ + Zn2+] [calc., 364.07]. The results of fluorescence titration of DHIC–Zn2+ species gave a binding constant of 1.7 × 104 M−1 by Benesi–Hildebrand analysis (Fig. S4†).61 The detection limit (3σ/K) of DHIC for Zn2+ was analyzed to be 1.59 μM (Fig. S5†). It was far below the WHO standard (76.0 μM).62
The fluorescence variation of DHIC for Zn2+ was investigated to check the interference of other cations (Fig. S6†). Fe3+, Cu2+, Cr3+, Fe2+ and Co2+ caused fluorescence quenching (78%–100%), but other metal ions did not interfere. Their interference may be due to their inherent quenching character of fluorescence.63,64 Meanwhile, Cd2+ did not inhibit the fluorescence emission of DHIC–Zn2+. These outcomes indicated that the compound DHIC could be a selective and effective sensor for Zn2+ and differentiate Zn2+ from Cd2+ having analogous properties. The pH effect on the sensing ability of DHIC for Zn2+ was examined in the wide pH range of 2–12 (Fig. S7†). The fluorescence intensity of DHIC with Zn2+ was kept between pH 7 and 11.
To comprehend the detection by DHIC of Zn2+, 1H NMR titration was executed (Fig. 3). On the addition of Zn2+ to DHIC, the hydroxyl proton H5 disappeared. The imidazole proton H1 was shifted downfield, while the imine proton H4 was a little shifted to upfield. These outcomes implied that the imine nitrogen atom and the hydroxyl oxygen atom may bind to Zn2+. Further addition (>1.0 equiv.) of Zn2+ showed no change of the proton positions, which suggested the ratio 1
:
1 of DHIC–Zn2+. Based on ESI-mass spectrometry, 1H NMR titration and Job plot analysis, a possible structure of the zinc complex was suggested (Scheme 2).
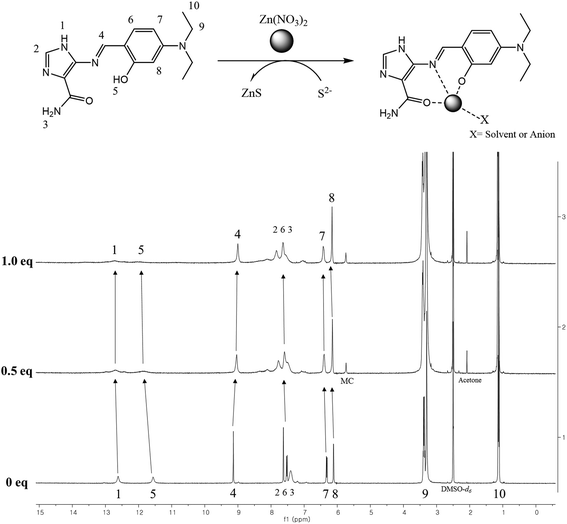 |
| Fig. 3
1H NMR titration of DHIC with Zn2+ in DMSO-d6. | |
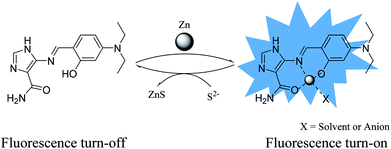 |
| Scheme 2 Proposed structure of the DHIC–Zn2+ complex. | |
To study the reversibility of DHIC toward Zn2+, ethylenediaminetetraacetic acid (EDTA, 21 equiv.) was added to DHIC–Zn2+ (Fig. S8†). Addition of EDTA decreased the fluorescence intensity of DHIC–Zn2+, which is indicative of regeneration of free DHIC. On the addition of Zn2+ again, fluorescence emission returned to the original intensity of DHIC–Zn2+ species. These results showed that DHIC can be recycled with appropriate reagents like EDTA. The practical application of DHIC for sensing Zn2+ in real water samples was investigated by using a calibration curve. The curve showed a satisfying linearity between Zn2+ concentration and the fluorescence emission of DHIC (Fig. S9†). On the basis of the curve, DHIC was applied to the water samples (Table 1). Good recoveries and R.S.D. values were achieved for the samples.
Table 1 Determination of Zn(II) in water samplesa
Sample |
Zn(II) added (μmol L−1) |
Zn(II) found (μmol L−1) |
Recovery (%) |
R.S.D. (n = 3) (%) |
Conditions: [DHIC] = 10 μmol L−1 in 10 mM Bis–Tris buffer–DMF solution (997 : 3, pH 7.0).
|
Drinking water |
0.00 |
0.00 |
— |
— |
5.00 |
4.96 |
99.2 |
3.53 |
Fluorescence response of DHIC–Zn2+ to S2−
Based on the high affinity of Zn and sulfide,64 a fluorescence spectral study of the DHIC–Zn2+ complex for a wide variety of anions (OAc−, I−, CN−, F−, S2−, SCN−, H2PO4−, Br−, B2O−, N3−, NO2− and Cl−) was performed in Bis–Tris buffer. With excitation at 420 nm, only S2− reduced immediately the fluorescence intensity (Fig. 4). These observations indicated that DHIC–Zn2+ could be a great selective probe for S2−. Just in case, other sulfur-containing species, such as cysteine (Cys), homocysteine (Hcy), and glutathione (GSH), were also tested, but they did not show any change.
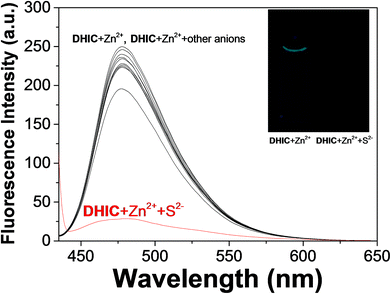 |
| Fig. 4 Fluorescence selectivity of DHIC–Zn2+ (10.0 μM) with different anions (34 equiv.) with excitation at 420 nm. | |
We performed fluorescence titration of DHIC–Zn2+ with S2− to study the sensing properties of DHIC–Zn2+ species (Fig. 5). On the incremental addition of S2− to DHIC–Zn2+, the fluorescence emission was decreased constantly and saturated with 34 equiv. of S2−. To examine the binding properties of DHIC–Zn2+ with S2−, UV-vis titration was performed (Fig. S10†). On the addition of S2− to DHIC–Zn2+, the absorbance at 300 and 500 nm increased, while the band at 450 nm declined with a distinct isosbestic point (472 nm).
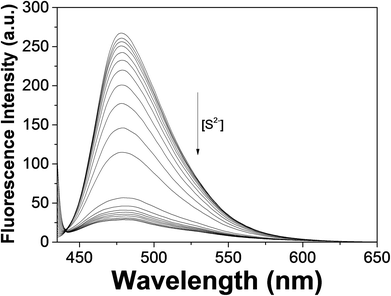 |
| Fig. 5 Fluorescence changes of DHIC–Zn2+ (10.0 μM) with different S2− concentrations. | |
To inspect the binding interaction between S2− and DHIC–Zn2+, a Job plot analysis was performed (Fig. S11†). It gave a 1
:
1 interaction ratio. In addition, their 1
:
1 ratio was affirmed by ESI-mass spectrometry. The positive-ion spectrum suggested that the peak at m/z = 302.10 was indicative of [DHIC + H+] (calc., 302.16) (Fig. S12†). The results assumed that the demetallation of the DHIC–Zn2+ complex occurred to form ZnS. With fluorescence titration, the association constant of 2.0 × 103 M−1 was afforded for DHIC–Zn2+ with sulfide by the Benesi–Hildebrand equation (Fig. S13†).61 The detection limit (3σ/K) was evaluated to be 8.03 μM, which is below the WHO standard (14.7 μM) (Fig. S14†).65
To study the preferential selectivity for Zn2+, a competition test was carried out (Fig. 6). With various anions (34 equiv.; SCN−, I−, OAc−, F−, N3−, Br−, H2PO4−, CN−, Cl−, B2O− and NO2−), no interference was observed for detecting S2−. These outcomes demonstrated that the DHIC–Zn2+ complex could be an excellent fluorescence probe for detecting S2− without the interference of other anions. To investigate the influence of pH, the fluorescence response to S2− was measured at a broad range of pH (2–12; Fig. S15†). Upon the addition of S2− to DHIC–Zn2+, the fluorescence emission showed a momentous decrease between pH 7 and 11, implying that DHIC–Zn2+ species could be used to detect S2− in a biological range of pH.
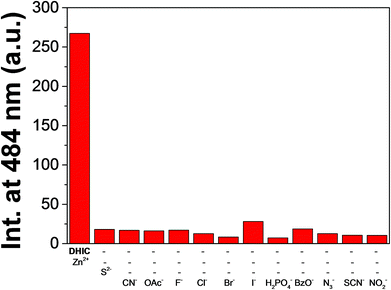 |
| Fig. 6 Competitive test of DHIC–Zn2+ (10.0 μM) to S2− (34 equiv.) with other anions (34 equiv.) with excitation at 420 nm. | |
Cell imaging tests of DHIC with Zn2+ and S2−
For the applicability of DHIC in biological systems, its cytotoxicity was examined with HeLa cells by the MTT assay (Fig. S16†). HeLa cells were incubated with DHIC (10 and 20 μM) for 1 h and 24 h, respectively. After the incubation, cell viability turned out to be ca. 95% (1 h incubation) and 90% (24 h incubation) at both concentrations, indicating that DHIC was nontoxic under our experimental conditions. To evaluate the practical application of detecting Zn2+ and S2− in living cells, fluorescence imaging was performed (Fig. 7). HeLa cells were bred with DHIC for 1 h and exposed to Zn2+ for another 1 h. Fluorescence in the cells did not appear without Zn2+. Contrastively, the presence of Zn2+ showed the increased fluorescence (Fig. 7(a)). Furthermore, the fluorescence of cells having DHIC–Zn2+ species exposed to S2− for 1 h disappeared absolutely (Fig. 7(b)). These observations suggested that the compound DHIC could recognize consecutively Zn2+ and S2− in living cells.
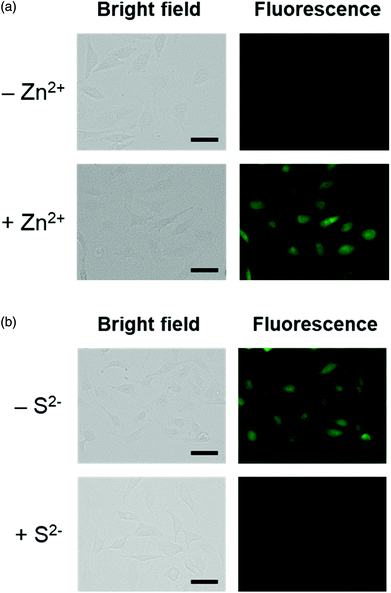 |
| Fig. 7 (a) Fluorescence imaging of HeLa cells incubated with DHIC followed by the addition of Zn2+. Cells were preincubated with DHIC for 1 h prior to treatment with Zn2+. Conditions: [DHIC] = 20 μM; [Zn2+] = 600 μM; 37 °C; 5% CO2. The scale bar is 50 μm. (b) Fluorescence imaging of HeLa cells incubated with DHIC and Zn2+ followed by the addition of S2−. Fluorescence of the cells treated with both DHIC and Zn2+ was quenched by introducing S2−. Conditions: [DHIC] = 20 μM; [Zn2+] = 600 μM; [S2−] = 400 μM; 37 °C; 5% CO2. The scale bar is 50 μm. | |
Colorimetric sensing of DHIC for Fe3+/2+
The colorimetric recognizing behavior of the compound DHIC with a wide range of metal ions (Pb2+, Mn2+, Fe2+, K+, Cu2+, Co2+, Mg2+, Cd2+, Cr3+, Na+, Ni2+, Zn2+, Ca2+, Al3+ and Fe3+) was also examined in Bis–Tris buffer (Fig. 8). DHIC showed immediate changes of color from pale yellow to orange yellow with an increase of the absorbance at 500 nm in the presence f Fe3+/2+ ions, while no change was shown with other cations. This result illustrated that DHIC could be an efficient colorimetric detector for Fe3+/2+ in aqueous media. Importantly, among the chemosensors reported to date, the compound DHIC is the first chemosensor that can recognize the four analytes, Zn2+, S2−, and Fe3+/2+.
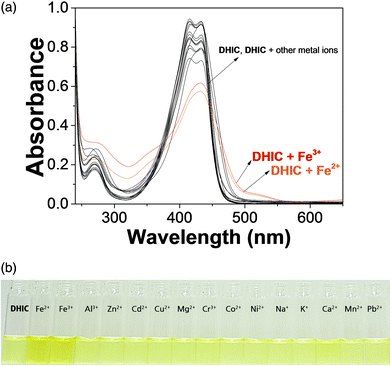 |
| Fig. 8 (a) UV-vis changes of DHIC (20.0 μM) upon the addition of various cations. (b) The color changes of DHIC (20.0 μM) with different cations (22 equiv.). | |
UV-vis titrations of DHIC for Fe3+ and Fe2+ were performed to investigate their binding characteristics. Upon the addition of Fe3+ ions (Fig. 9), the UV-vis absorption spectrum showed that the absorption at 500 nm constantly increased and the absorption at 420 nm declined with an isosbestic point of 446 nm. The point demonstrated that a species was produced from the complexation of DHIC with Fe3+. UV-vis variation of DHIC upon the addition of Fe2+ was analogous to that of DHIC with Fe3+ (Fig. S17†).
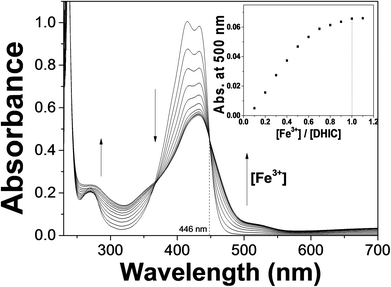 |
| Fig. 9 UV-vis absorption changes of DHIC (20.0 μM) with different Fe3+ concentrations (from 0 to 1.1 equiv.). Inset: Absorbance at 500 nm versus the number of equiv. of Fe3+ added. | |
Job plot analysis was performed to study the interaction modes between DHIC and Fe3+ and Fe2+. As shown in Fig. S18 and S19,† they showed 2
:
1 stoichiometries. Also, the interaction modes between DHIC and Fe3+/2+ were affirmed with ESI mass spectrometry (Fig. S20†). Positive-ion mass data of DHIC upon the addition of Fe3+ and Fe2+ were indicative of the formation of 2·DHIC + Fe3+ − 2H+ [m/z: 656.20; calc.: 656.23]. These outcomes drove us to assume that Fe2+ of DHIC–Fe2+ produced from the interaction of DHIC with Fe2+ may be quickly oxidized to Fe3+ in air (Scheme 3). To demonstrate our suggestion, we investigated the UV-vis variations for the complexation of DHIC with Fe2+ under anaerobic and aerobic conditions (Fig. S21†). When DHIC and Fe2+ interacted in the absence of oxygen molecules, there was little spectral change of DHIC. However, the spectrum of the DHIC–Fe2+ complex exposed to air was significantly varied, and finally, analogous to the spectrum of the Fe3+-2·DHIC complex. Additionally, the time-dependent variations for the interactions of DHIC with Fe3+/2+ displayed that the formation period (30 min) of the Fe3+-2·DHIC complex produced from the interaction of DHIC with Fe2+ was longer than that (12 min) afforded by the interaction of DHIC with Fe3+ (Fig. S22†). These observations indirectly proved our proposal. Based on the Job plots, time-dependence experiments, ESI-mass data, and degassing experiments, the possible structure of the iron complex is suggested in Scheme 3. On the other hand, the superoxide radical generated from the oxidation reaction of the DHIC–Fe2+ complex with air (O2) might react with another Fe2+ complex to produce the Fe3+-2·DHIC complex.
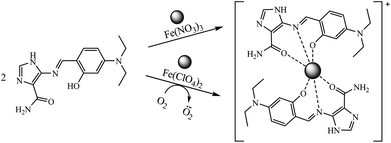 |
| Scheme 3 Proposed structure of the Fe3+-2·DHIC complex. | |
With the UV-vis titrations, the K values of DHIC with Fe3+/2+ were calculated to be 5.0 × 1010 M−1 and 1.0 × 1010 M−1 by using Li's equation (Fig. S23 and S24†).66 The detection limit (3σ/K) of DHIC for Fe3+ (or Fe2+) was calculated to be 0.73 μM (or 1.11 μM) (Fig. S25 and S26†). The detection limit of DHIC for iron was far below the EPA standard (5.37 μM).42
To assess the influence of other cations on the Fe3+/2+-2·DHIC, competitive tests were performed (Fig. 10 and S27†). There are no interferences with the detection of Fe3+/2+, except for Cu2+ ions. Cu2+ inhibited ca. 50% of the absorption peak. These facts indicated that DHIC could be a valuable chemosensor capable of detecting Fe3+/2+ without the interference of other cations. To investigate the influence of pH, pH tests of Fe3+/2+ detection by DHIC were performed in a broad range of pH (2–12). As shown in Fig. S28 and S29,† the absorption of DHIC at 500 nm with Fe3+ increased between pH 4 and 10. For Fe2+, the absorption of DHIC at 500 nm also increased between pH 7 and 10. These outcomes indicated that DHIC could recognize iron ions in a broad pH range.
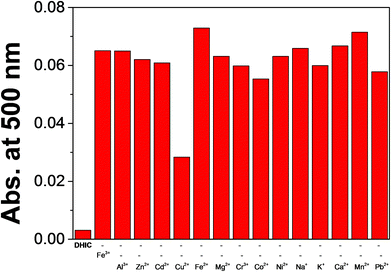 |
| Fig. 10 UV-vis competitive test of DHIC (20.0 μM) toward Fe3+ with other cations. | |
To investigate the efficient application of DHIC to Fe3+ in real samples, a calibration plot was established for the determination of Fe3+. The plot displayed a satisfying linearity between Fe3+ concentration and the absorbance of DHIC (Fig. S30†). Based on the plots, DHIC was applied to water samples (Table 2). Suitable recoveries and R.S.D. values are given for the samples. These outcomes implied that DHIC may be efficient at detecting Fe3+ in real water samples.
Table 2 Determination of Fe(III) in water samplesa
Sample |
Fe(III) added (μmol L−1) |
Fe(III) found (μmol L−1) |
Recovery (%) |
R.S.D. (n = 3) (%) |
Conditions: [DHIC] = 20 μmol L−1 in 10 mM Bis–Tris buffer–DMF solution (997 : 3, pH 7.0).
|
Drinking water |
0.00 |
0.00 |
— |
— |
5.00 |
5.03 |
100.6 |
3.53 |
4. Conclusion
We presented a novel fluorescent and colorimetric probe, DHIC, which showed great sensitivity and selectivity to Zn2+, S2−, and Fe3+/2+. DHIC could operate as a “turn on–off” fluorescent probe for the consecutive recognition of Zn2+ and S2−. The detection limit (1.59 μM) for Zn2+ ions was far below the WHO standard (76.0 μM). DHIC could be applied for drinking water samples for Zn2+ with satisfying R.S.D. values and recoveries. Significantly, DHIC can recognize consecutively Zn2+ and S2− in living cells. In addition, DHIC could selectively detect Fe3+/2+ by the naked-eye from pale yellow to orange yellow with very low detection limits (0.73 μM and 1.11 μM), which are far lower than the EPA standard (5.37 μM). The analytical methods such as the degassing test and ESI-mass spectrometry have illustrated that Fe2+ of the Fe2+-2·DHIC species was quickly converted to Fe3+ in air. Moreover, DHIC could quantify Fe3+ in real samples. Significantly, among the chemosensors reported to date, DHIC is the first chemosensor that can recognize the four analytes, Zn2+, S2−, and Fe3+/2+. Thus, we believe that the compound DHIC will contribute to the design of a new kind of fluorescent and colorimetric probe for multiple targets in biological and environmental systems.
Conflicts of interest
There are no conflicts to declare.
Acknowledgements
This work was kindly supported by the National Research Foundation of Korea (Grant No. NRF-2018R1A2B6001686 and NRF-2017R1A2B3002585).
References
- J. Zhu, Y. Zhang, Y. Chen, T. Sun, Y. Tang, Y. Huang, Q. Yang, D. Ma, Y. Wang and M. Wang, A Schiff base fluorescence probe for highly selective turn-on recognition Zn2+, Tetrahedron Lett., 2017, 58, 365–370 CrossRef CAS.
- S. Yin, J. Zhang, H. Feng, Z. Zhao, L. Xu, H. Qiu and B. Tang, Zn2+-selective fluorescent turn-on chemosensor based on terpyridine-substituted siloles, Dyes Pigm., 2012, 95, 174–179 CrossRef CAS.
- D. Maity and T. Govindaraju, A differentially selective sensor with fluorescence turn-on response to Zn2+ and dual-mode ratiometric response to Al3+ in aqueous media, Chem. Commun., 2012, 48, 1039–1041 RSC.
- A. Helal, M. Harun, O. Rashid, C. Choi and H. Kim, New regioisomeric naphthol-substituted thiazole based ratiometric fluorescence sensor for Zn2+ with a remarkable red shift in emission spectra, Tetrahedron, 2012, 68, 647–653 CrossRef CAS.
- J. M. Jung, S. Y. Lee, E. Nam, M. H. Lim and C. Kim, A highly selective turn-on chemosensor for Zn2+ in aqueous media and living cells, Sens. Actuators, B, 2017, 244, 1045–1053 CrossRef CAS.
- D. Jeyanthi, M. Iniya, K. Krishnaveni and D. Chellappa, Charge transfer based “turn-on” chemosensor for Zn2+ ion recognition using new triaryl pyrazoline derivative, Spectrochim. Acta, Part A, 2016, 159, 231–237 CrossRef CAS PubMed.
- K. T. Kim, S. A. Yoon, J. Ahn, Y. Choi, M. H. Lee, J. H. Jung and J. Park, Chemical Synthesis of fluorescent naphthalimide-functionalized Fe3O4 nanoparticles and their application for the selective detection of Zn2+ present in contaminated soil, Sens. Actuators, B, 2017, 243, 1034–1041 CrossRef CAS.
- X. Chen, S. Nam, G.-H. Kim, N. Song, Y. Jeong, I. Shin, S. K. Kim, J. Kim, S. Park and J. Yoon, A near-infrared fluorescent sensor for detection of cyanide in aqueous solution and its application for bioimaging, Chem. Commun., 2010, 46, 8953–8955 RSC.
- N. Narayanaswamy, D. Maity and T. Govindaraju, Reversible fluorescence sensing of Zn2+ based on pyridine-constrained bis(triazole-linked hydroxyquinoline) sensor, Supramol. Chem., 2011, 23, 703–709 CrossRef CAS.
- A. Bhattacharyya, S. Ghosh, S. C. Makhal and N. Guchhait, Hydrazine bridged coumarin-pyrimidine conjugate as a highly selective and sensitive Zn2+ sensor: Spectroscopic unraveling of sensing mechanism with practical application, Spectrochim. Acta, Part A, 2017, 183, 306–311 CrossRef CAS PubMed.
- K. Wang, Z. Jin, H. Shang, C. Lv, Q. Zhang, S. Chen and Z. Hu, A highly selective fluorescent chemosensor for Zn2+ based on the rhodamine derivative incorporating coumarin group, J. Fluoresc., 2017, 27, 629–633 CrossRef CAS PubMed.
- R. Singh, A. Gogoi and G. Das, Benzothiazole based multi-analyte sensor for selective sensing of Zn2+ and Cd2+ and subsequent sensing of inorganic phosphates (Pi) in mixed aqueous medium, RSC Adv., 2016, 6, 112246–112252 RSC.
- F. Yu, X. Guo, X. Tian and L. Jia, A ratiomeric fluorescent sensor for Zn2+ based on N,N′-Di(quinolin-8-yl)oxalamide, J. Fluoresc., 2017, 27, 723–728 CrossRef CAS PubMed.
- S. Goswami, A. Manna, S. Paul, A. K. Maity, P. Saha, C. K. Quah and H. Fun, FRET based ‘red-switch’ for Al3+ over ESIPT based ‘green-switch’ for Zn2+: dual channel detection with live-cell imaging on a dyad platform, RSC Adv., 2014, 4, 34572–34576 RSC.
- Z. Xu, G. Kim, S. Jung, M. Jung, C. Lee, I. Shin and J. Yoon, An NBD-based colorimetric and fluorescent chemosensor for Zn2+ and its use for detection of intracellular zinc ions, Tetrahedron, 2009, 65, 2307–2312 CrossRef CAS.
- L. Li, S. Yun, Z. Yuan-Hui, M. Lan, Z. Xi, C. Redshaw and W. Gang, A single chemosensor for multiple analytes: Fluorogenic and ratiometric absorbance detection of Zn2+, Mg2+ and F−, and its cell imaging, Sens. Actuators, B, 2016, 226, 279–288 CrossRef CAS.
- Z. Xu, K. Baek, H. N. Kim, J. Cui, X. Qian, D. R. Spring, I. Shin and J. Yoon, Zn2+-Triggered Amide Tautomerization Produces a Highly Zn2+-Selective, Cell-Permeable, and Ratiometric Fluorescent Sensor, J. Am. Chem. Soc., 2010, 132, 601–610 CrossRef CAS PubMed.
- A. Helal, S. H. Kim and H. Kim, Thiazole sulfonamide based ratiometric fluorescent chemosensor with a large spectral shift for zinc sensing, Tetrahedron, 2010, 66, 9925–9932 CrossRef CAS.
- L. He, X. Yang, Y. Liu and W. Lin, Colorimetric and ratiometric fluorescent probe for hydrogen sulfide using a coumarin-pyronine FRET dyad with a large emission shift, Anal. Methods, 2016, 8, 8022–8027 RSC.
- Y. Wang, X. Lv and W. Guo, A reaction-based and highly selective fl uorescent probe for hydrogen sulfide, Dyes Pigm., 2017, 139, 482–486 CrossRef CAS.
- B. Deng, M. Ren, J. Wang, K. Zhou and W. Lin, A mitochondrial-targeted two-photon fluorescent probe for imaging hydrogen sulfide in the living cells and mouse liver tissues, Sens. Actuators, B, 2017, 248, 50–56 CrossRef CAS.
- R. Zhang, X. Yu, Y. Yin, Z. Ye, G. Wang and J. Yuan, Development of a heterobimetallic Ru(II)-Cu(II) complex for highly selective and sensitive luminescence sensing of sulfide anions, Anal. Chim. Acta, 2011, 691, 83–88 CrossRef CAS PubMed.
- S. M. Kim, M. Kang, I. Choi, J. J. Lee and C. Kim, A highly selective colorimetric chemosensor for cyanide and sulfide in aqueous solution : experimental and theoretical studies, New J. Chem., 2016, 40, 7768–7778 RSC.
- X. Feng, T. Zhang, J. Liu, J. Miao and B. Zhao, A new ratiometric fluorescent probe for rapid, sensitive and selective detection of endogenous hydrogen sulfide in mitochondria, Chem. Commun., 2016, 52, 3131–3134 RSC.
- P. Wang, J. Wu, P. Su, C. Xu, Y. Ge, D. Liu, W. Liu and Y. Tang, Fluorescence ‘on-off-on’ peptide-based chemosensor for the selective detection of Cu2+ and S2− and its application in living cell bioimaging, Dalton Trans., 2016, 45, 16246–16254 RSC.
- S. Chen, H. Li and P. Hou, A novel imidazo[1,5-α]pyridine-based fluorescent probe with a large stokes shift for imaging hydrogen sulfide, Sens. Actuators, B, 2017, 1–7 CrossRef.
- M. G. Choi, S. Cha, H. Lee, H. L. Jeon and S. K. Chang, Sulfide-selective chemosignaling by a Cu2+ complex of dipicolylamine appended fluorescein, Chem. Commun., 2009, 7390–7392 RSC.
- K. Zheng, W. Lin, L. Tan and D. Cheng, A two-photon fluorescent probe with a large turn-on signal for imaging hydrogen sulfide in living tissues, Anal. Chim. Acta, 2015, 853, 548–554 CrossRef CAS PubMed.
- P. Zhang, J. Li, B. Li, J. Xu, F. Zeng, J. Lv and S. Wu, A logic gate-based fluorescent sensor for detecting H2S and NO in aqueous media and inside live cells, Chem. Commun., 2015, 51, 4414–4416 RSC.
- Y. Jiang, Q. Wu and X. Chang, A ratiometric fluorescent probe for hydrogen sulfide imaging in living cells, Talanta, 2014, 121, 122–126 CrossRef CAS PubMed.
- B. Gu, W. Su, L. Huang, C. Wu, X. Duan, Y. Li, H. Xu, Z. Huang, H. Li and S. Yao, Real-time tracking and selective visualization of exogenous and endogenous hydrogen sulfide by a near-infrared fluorescent probe, Sens. Actuators, B, 2018, 255, 2347–2355 CrossRef CAS.
- J. Zhang, R. Wang, Z. Zhu, L. Yi and Z. Xi, A FRET-based ratiometric fluorescent probe for visualizing H2S in lysosomes, Tetrahedron, 2015, 71, 8572–8576 CrossRef CAS.
- Ş. N. Karuk Elmas, F. Ozen, K. Koran, I. Yilmaz, A. O. Gorgulu and S. Erdemir, Coumarin Based Highly Selective “off-on-off” Type Novel Fluorescent Sensor for Cu2+ and S2− in Aqueous Solution, J. Fluoresc., 2017, 27, 463–471 CrossRef PubMed.
- J. Li, C. Yin and F. Huo, Chromogenic and fluorogenic chemosensors for hydrogen sulfide: Review of detection mechanisms since the year 2009, RSC Adv., 2015, 5, 2191–2206 RSC.
- Q. Meng, Y. Shi, C. Wang, H. Jia, X. Gao, R. Zhang, Y. Wang and Z. Zhang, NBD-based fluorescent chemosensor for the selective quantification of copper and sulfide in an aqueous solution and living cells, Org. Biomol. Chem., 2015, 13, 2918–2926 RSC.
- Q. Meng, R. Zhang, H. Jia, X. Gao, C. Wang, Y. Shi, A. V. Everest-Dass and Z. Zhang, A reversible fluorescence chemosensor for sequentially quantitative monitoring copper and sulfide in living cells, Talanta, 2015, 143, 294–301 CrossRef CAS PubMed.
- K. B. Kim, H. Kim, E. J. Song, S. Kim, I. Noh and C. Kim, A cap-type Schiff base acting as a fluorescence sensor for zinc(II) and a colorimetric sensor for iron(II), copper(II), and zinc(II) in aqueous media, Dalton Trans., 2013, 42, 16569–16577 RSC.
- H. Jung, N. Singh and D. Ok, Highly Fe3+ selective ratiometric fluorescent probe based on imine-linked benzimidazole, Tetrahedron Lett., 2008, 49, 2960–2964 CrossRef CAS.
- S. Das, K. Aich, S. Goswami, C. K. Quah and H. Fun, FRET-based fluorescence ratiometric and colorimetric sensor to discriminate Fe3+ from Fe2+, New J. Chem., 2016, 40, 6414–6420 RSC.
- N. Narayanaswamy and T. Govindaraju, Aldazine-based colorimetric sensors for Cu2+ and Fe3+, Sens. Actuators, B, 2012, 161, 304–310 CrossRef CAS.
- H. Jia, X. Gao, Y. Shi, N. Sayyadi, Z. Zhang, Q. Zhao, Q. Meng and R. Zhang, Fluorescence detection of Fe3+ ions in aqueous solution and living cells based on a high selectivity and sensitivity chemosensor, Spectrochim. Acta, Part A, 2015, 149, 674–681 CrossRef CAS PubMed.
- US EPA, Secondary drinking water standards: Guidance for Nuisance Chemicals.
- V. K. Bhardwaj, P. Saluja, G. Hundal, M. S. Hundal, N. Singh and D. O. Jang, Benzthiazole-based multifunctional chemosensor: Fluorescent recognition of Fe3+ and chromogenic recognition of HSO4−, Tetrahedron, 2013, 69, 1606–1610 CrossRef CAS.
- Z. Chi, X. Ran, L. Shi, J. Lou, Y. Kuang and L. Guo, Molecular characteristics of a fluorescent chemosensor for the recognition of ferric ion based on photoresponsive azobenzene derivative, Spectrochim. Acta, Part A, 2017, 171, 25–30 CrossRef CAS PubMed.
- Y. S. Kim, J. J. Lee, S. Y. Lee, T. G. Jo and C. Kim, A highly sensitive benzimidazole-based chemosensor for the colorimetric detection of Fe(II) and Fe(III) and the fluorometric detection of Zn(II) in aqueous media, RSC Adv., 2016, 6, 61505–61515 RSC.
- S. Goswami, S. Das, K. Aich, D. Sarkar, T. K. Mondal, C. K. Quah and H. K. Fun, CHEF induced highly selective and sensitive turn-on fluorogenic and colorimetric sensor for Fe3+, Dalton Trans., 2013, 42, 15113–15119 RSC.
- M. Zhao, X. Zhou, J. Tang, Z. Deng, X. Xu, Z. Chen, X. Li, L. Yang and L. Ma, Pyrene excimer-based fluorescent sensor for detection and removal of Fe3+ and Pb2+ from aqueous solutions, Spectrochim. Acta, Part A, 2017, 173, 235–240 CrossRef CAS PubMed.
- Y. Liu, R. Shen, J. Ru, X. Yao, Y. Yang, H. Liu, X. Tang, D. Bai, G. Zhang and W. Liu, A reversible rhodamine 6G-based fluorescence turn-on probe for Fe3+ in water and its application in living cell imaging, RSC Adv., 2016, 6, 111754–111759 RSC.
- J. Jun Lee, G. Jin Park, Y. Sung Kim, S. Young Lee, H. Ji Lee, I. Noh and C. Kim, A water-soluble carboxylic-functionalized chemosensor for detecting Al3+ in aqueous media and living cells: Experimental and theoretical studies, Biosens. Bioelectron., 2015, 69, 226–229 CrossRef CAS PubMed.
- M. Hosseini, Z. Vaezi, M. R. Ganjali, F. Faridbod, S. D. Abkenar, K. Alizadeh and M. Salavati-Niasari, Fluorescence ‘turn-on’ chemosensor for the selective detection of zinc ion based on Schiff-base derivative, Spectrochim. Acta, Part A, 2010, 75, 978–982 CrossRef PubMed.
- M. Hosseini, A. Ghafarloo, M. R. Ganjali, F. Faridbod, P. Norouzi and M. S. Niasari, A turn-on fluorescent sensor for Zn2+ based on new Schiff's base derivative in aqueous media, Sens. Actuators, B, 2014, 198, 411–415 CrossRef CAS.
- Y. W. Choi, G. R. You, M. M. Lee, J. Kim, K. D. Jung and C. Kim, Highly selective recognition of mercury ions through the ‘naked-eye’, Inorg. Chem. Commun., 2014, 46, 43–46 CrossRef CAS.
- Y. J. Na, Y. W. Choi, J. Y. Yun, K. M. Park, P. S. Chang and C. Kim, Dual-channel detection of Cu2+ and F− with a simple Schiff-based colorimetric and fluorescent sensor, Spectrochim. Acta, Part A, 2015, 136, 1649–1657 CrossRef CAS PubMed.
- Y. Wang, H. Q. Wu, J. H. Sun, X. Y. Liu, J. Luo and M. Q. Chen, A novel chemosensor based on rhodamine derivative for colorimetric and fluorometric detection of Cu2+ in aqueous solution, J. Fluoresc., 2012, 22, 799–805 CrossRef CAS PubMed.
- S. Sarkar, T. Mondal, S. Roy, R. Saha, A. K. Ghosh and S. S. Panja, A multi-responsive thiosemicarbazone-based probe for detection and discrimination of group 12 metal ions and its application in logic gates, New J. Chem., 2018, 42, 15157–15169 RSC.
- Y. K. La, J. A. Hong, Y. J. Jeong and J. Lee, A 1,8-naphthalimide-based chemosensor for dual-mode sensing: Colorimetric and fluorometric detection of multiple analytes, RSC Adv., 2016, 6, 84098–84105 RSC.
- W. H. Ding, D. Wang, X. J. Zheng, W. J. Ding, J. Q. Zheng, W. H. Mu, W. Cao and L. P. Jin, A turn-on fluorescence chemosensor for Al3+, F− and CN− ions, and its application in cell imaging, Sens. Actuators, B, 2015, 209, 359–367 CrossRef CAS.
- K. Liu, X. Zhao, Q. Liu, J. Huo, Z. Li and X. Wang, A novel multifunctional BODIPY-derived probe for the sequential recognition of Hg2+ and I−, and the fluorometric detection of Cr3+, Sens. Actuators, B, 2017, 239, 883–889 CrossRef CAS.
- K. Krishnaveni, M. Iniya, D. Jeyanthi, A. Siva and D. Chellappa, A new multifunctional benzimidazole tagged coumarin as ratiometric fluorophore for the detection of Cd2+/F− ions and imaging in live cells, Spectrochim. Acta, Part A, 2018, 205, 557–567 CrossRef CAS PubMed.
- M. Iniya, D. Jeyanthi, K. Krishnaveni and D. Chellappa, A bifunctional chromogenic and fluorogenic probe for F− and Al3+ based on azo-benzimidazole conjugate, J. Lumin., 2015, 157, 383–389 CrossRef CAS.
- H. A. Benesi and J. H. Hildebrand, A Spectrophotometric Investigation of
the Interaction of Iodine with Aromatic Hydrocarbons, J. Am. Chem. Soc., 1949, 71, 2703–2707 CrossRef CAS.
- Y. W. Choi, G. R. You, J. J. Lee and C. Kim, Turn-on fluorescent chemosensor for selective detection of Zn2+ in an aqueous solution: Experimental and theoretical studies, Inorg. Chem. Commun., 2016, 63, 35–38 CrossRef CAS.
- Y. Liu, Q. Fei, H. Shan, M. Cui, Q. Liu, G. Feng and Y. Huan, A novel fluorescent ‘off-on-off’ probe for relay recognition of Zn2+ and Cu2+ derived from N,N-bis(2-pyridylmethyl)amine, Analyst, 2014, 139, 1868–1875 RSC.
- J. Y. Yun, T. G. Jo, J. Han, H. J. Jang, M. H. Lim and C. Kim, A highly sensitive and selective fluorescent chemosensor for the sequential recognition of Zn2+ and S2− in living cells and aqueous media, Sens. Actuators, B, 2018, 255, 3108–3116 CrossRef CAS.
-
Guidelines for drinking-water quality. Vol. 2, Health criteria and other supporting information: addendum, World Health Organization, Geneva, 2nd edn, 1998 Search PubMed.
- G. Grynkiewicz, M. Poenie and R. Y. Tsien, A new generation of Ca2+ indicators with greatly improved fluorescence properties, J. Biol. Chem., 1985, 260, 3440–3450 CAS.
Footnote |
† Electronic supplementary information (ESI) available. See DOI: 10.1039/c8pp00408k |
|
This journal is © The Royal Society of Chemistry and Owner Societies 2019 |
Click here to see how this site uses Cookies. View our privacy policy here.