DOI:
10.1039/C8QI01026A
(Research Article)
Inorg. Chem. Front., 2019,
6, 74-81
Self-supported Al-doped cobalt phosphide nanosheets grown on three-dimensional Ni foam for highly efficient water reduction and oxidation†
Received
25th September 2018
, Accepted 25th October 2018
First published on 26th October 2018
Abstract
Developing efficient non-noble-metal electrocatalysts for overall water-splitting is still a great challenge for future renewable energy conversion and storage systems. Herein, cross-linked Al-doped CoP nanosheets on three-dimensional (3D) nickel foam (Al-CoP/NF) are synthesized by a simple low-temperature phosphidation method using a CoAl layer double hydroxide precursor and exhibit high activity and good stability towards the hydrogen evolution reaction (HER) at all pH values, with low overpotentials of 51 mV (0.5 M H2SO4, pH = 0), 66 mV (1.0 M KOH, pH = 14) and 83 mV (1.0 M PBS, pH = 7) to achieve a current density of 10 mA cm−2. In addition, this self-supported electrode shows excellent activity towards the oxygen evolution reaction (OER) in alkaline media, affording an anodic current density of 10 mA cm−2 at an overpotential of 330 mV. As a result, Al-CoP/NF as a bifunctional electrocatalyst can afford a current density of 10 mA cm−2 at a cell voltage of 1.63 V over a long-term overall water splitting operation in 1.0 M KOH. The remarkable catalytic performance of the self-supported Al-CoP/NF is closely associated with its outstanding structural advantages such as a 3D conductive framework, fascinating porous characteristics and Al doping. This study might open up a new route to improve the activity and durability of self-supported electrocatalysts by heteroatom doping.
1. Introduction
Recently, the depletion of fossil fuels and the deterioration of the environment have triggered the urgent need to develop clean, cheap and recyclable alternative energy sources.1,2 Hydrogen is considered to be one of the most promising alternative fuels due to its cleanness and sustainability.3,4 Among the strategies considered, electrocatalytic water-splitting is an efficient route for high-purity hydrogen generation. The state-of-the-art electrocatalysts for the hydrogen evolution reaction (HER) are noble metal Pt-based catalysts; however, the high cost and low availability of Pt limit their large-scale application.5 As such, it is highly desired to design and develop alternative cost-effective electrocatalysts showing high activity and excellent durability to Pt-based catalysts for HER.
Featuring low cost and excellent electrocatalytic performance, transition metal phosphides (TMPs) have been intensively studied in recent years, for example, CoP,6–8 NiP,9–11 MoP,12,13 CuP,14 FeP15 and WP.16 Among them, CoP catalysts have attracted tremendous attention owing to their intriguing electrochemical conductivity and excellent HER electrocatalytic activity.17–19 Normally, expensive binders (Nafion or PTFE) are required in the powder catalyst preparation process for effectively immobilizing them on the electrode surface, but the catalytic activity will be greatly reduced because of the addition of polymer binders.20–22 Distinctively, self-supported catalysts can be directly used as a hydrogen-evolution electrode to generate hydrogen without any binding agents, with structural advantages over powder catalysts in electrocatalytic applications.23,24 The electron-transport kinetics of self-supported catalysts can be remarkably improved owing to the high efficiency of charge transfer from catalyst nanoparticles to the support substrate. Moreover, the flexible 3D integrated frame can provide enriched active boundary sites and avoid the collapse of the structure, thus greatly enhancing the activity and stability of the catalysts.25–32 A few self-supported electrocatalysts with outstanding electrocatalytic properties and stability have been synthesized for water splitting, for example, CoP/NF,6 Ni2P/NF9 and CoP@NPC/CP.33 Nevertheless, the HER catalytic activity and stability of such catalysts still need to be further improved to compete with Pt-based catalysts.
It is highlighted that non-metal- and/or metal-doped TMPs hold great promise as candidates for the HER due to the synergistic interaction between the doping elements and catalyst nanoparticles. Featuring adjustable compositions and structures, non-metal (C, N or S elements) doped materials have been widely used for the HER and the oxygen evolution reaction (OER), for example, CoMoP@C,34 Co2P@Co/N-C,35 Ni2P/NRGO,36 FeP@NPCs,37 and MoP/S.38 However, the doping amount of non-metal elements cannot be controlled well owing to their instability at high temperatures. By contrast, metal (Al, Mn or Fe elements) doped materials are more competitive due to their low cost and good stability, and their doping amount can also be accurately controlled. In addition, metal-doping can effectively modify the electronic structure of catalysts and greatly reduce the hydrogen adsorption free energy (ΔGH*) of the catalysts,39–45 causing an enhanced charge-transfer ability. Recent studies verified that such a configuration indeed led to robust water-splitting catalyst electrodes, such as Mn-CoP/Ti44 and Fe-Ni2P/CC.45
Considering the outstanding characteristics of the self-supported system and the excellent advantages of metal doping, we herein rationally design and synthesize self-supported Al-doped CoP nanosheets on three-dimensional (3D) Ni foam (Al-CoP/NF). The as-synthesized Al-CoP/NF electrode can be directly used as a cathode for the HER, and exhibits high activity under acidic, alkaline and neutral conditions, with low overpotentials of 51, 66 and 83 mV to achieve a current density of 10 mA cm−2, respectively, which outperforms most of the reported non-noble-metal HER catalysts. Also this electrode shows excellent activity towards OER in alkaline media, affording an anodic current density of 10 mA cm−2 at an overpotential of 330 mV. Furthermore, it can behave as an excellent bifunctional catalyst for the electrolysis of alkaline water and 1.63 V is required to achieve a current density of 10 mA cm−2 for its two-electrode water electrolyser in 1.0 M KOH. The high catalytic performance of Al-CoP/NF is closely linked to its outstanding structural advantages such as a 3D conductive framework, fascinating porous characteristics and Al doping. To the best of our knowledge, the superior self-supported Al-CoP/NF electrode with high activity and good stability for the HER and OER has rarely been reported. This study may provide new insights into the design and synthesis of promising cost-effective electrocatalysts for the HER and OER.
2. Experimental
2.1 Materials
Co(NO3)2·6H2O, Al(NO3)3·9H2O, NH4F, urea and NaH2PO2 were purchased from Tianjin Guangfu Chemical Co. Nickel foam (>99.5%, 1.0 mm thick) obtained from Taiyuan Yingze Lizhiyuan Battery. All chemicals were used as received without further purification.
2.2 Synthesis of Al-CoP/NF and CoP/NF
A CoAl layer double hydroxide precursor on Ni foam was prepared (CoAl-LDH/NF) as follows. In a typical synthesis, Co(NO3)2·6H2O (6 mmol), Al(NO3)3·9H2O (0.6 mmol), NH4F (8 mmol) and urea (20 mmol) were dissolved in deionized (DI) water (40 mL) under vigorous stirring for 1 h, affording a homogeneous pink solution. Meanwhile, a piece of commercial Ni foam (1 cm × 3.5 cm) was sonicated in 6 M HCl, ethanol and DI water, respectively, to clean the surface, and dried at 60 °C for 8 h. The above solution and cleaned Ni foam were transferred to an 80 mL Teflon-lined autoclave. The autoclave was sealed and maintained at 120 °C for 8 h in an electric oven. After the autoclave cooled slowly to room temperature, the CoAl-LDH/NF was taken out and washed several times with water, followed by vacuum drying at 60 °C. To convert the obtained CoAl-LDH/NF into Al-CoP/NF, NaH2PO2 and CoAl-LDH/NF were placed at two separate positions in a tube furnace with 0.8 g of NaH2PO2 at the upstream side, then annealed at 300 °C in N2 flow for 2 h, and allowed to cool naturally to ambient temperature under a N2 atmosphere. For a comparative study, CoP/NF was prepared under the same conditions in the absence of Al(NO3)3·9H2O. To investigate the influence of the doping amount of Al on the HER performance of Al-CoP/NF, two other Al-doped electrocatalysts with Co/Al molar ratios of 10
:
0.5 and 10
:
2, respectively, were synthesized under the same conditions.
2.3 Scratched Al-CoP nanosheets recoated on Ni foam (scratched Al-CoP/NF)
For comparison, the as-synthesized Al-CoP particles were scratched off Al-CoP/NF and dispersed in 1 mL of a water/ethanol (1
:
4) solution. Next, 40 μL of 5% Nafion reagent was added to the solution. The obtained solution was ultrasonicated for 30 min to obtain a homogeneous catalyst ink, which was further coated on the cleaned Ni foam and left to dry overnight. The final product was denoted as scratched Al-CoP/NF.
2.4 Physical characterization
Scanning electron microscopy (SEM) was performed on a Jeol JSF-7500 L microscope at 5 kV. Transmission electron microscopy (TEM) was performed on a Jeol JEM-2800 microscope at 200 kV. X-ray diffraction (XRD) patterns were acquired using a Bruker D8 Focus diffractometer with Cu-Kα radiation (λ = 0.15418 nm) operated at 40 kV and 40 mA. X-ray photoelectron spectroscopy (XPS) measurements were carried out on a Kratos Axis Ultra DLD (delay line detector) spectrometer equipped with a monochromatic Al-Kα X-ray source (1486.6 eV).
2.5 Electrochemical measurements
All the electrochemical measurements were performed in a standard three-electrode mode using the as-synthesized Al-CoP/NF as the working electrode, and a graphitic rod and the Ag/AgCl in 4 M KCl solution as the counter and reference electrodes, respectively. 0.5 M H2SO4 and 1.0 M KOH solutions were used as the electrolytes for the HER and OER, respectively. Before the electrochemical measurements, the electrolyte 0.5 M H2SO4 and the electrolyte 1.0 M KOH were degassed by using H2 and O2 for 30 min, respectively. Linear sweep voltammetry (LSV) curves with a sweep rate of 2 mV s−1 were recorded on an electrochemical workstation (Pine Research Instrumentation, USA). The polarization curves were acquired and corrected for iR compensation within the cell.46 The Tafel slope was acquired according to the Tafel equation:
η = b × log J + a |
where η is the overpotential, b is the Tafel slope and J is the current density.
The current density was normalized to the geometrical surface area and all the potentials reported in our work were the potential vs. RHE:
ERHE = EAg/AgCl + 0.059pH + 0.205 |
The durability of the electrode was tested by both cyclic voltammetry (CV) scanning and chronopotentiometric response. Electrochemical impedance spectroscopy (EIS) was performed using a Zahner IM6eX (Zahner, Germany) electrochemical analyzer at different overpotentials ranging from 0.01 to 100 kHz at an AC voltage of 5 mV.
3. Results and discussion
3.1 Materials characterization
The self-supported cross-linked Al-doped CoP nanosheets on 3D Ni foam (Al-CoP/NF) were fabricated by a simple low-temperature phosphidation method using a CoAl layer double hydroxide precursor (CoAl LDH/NF), presenting an obvious colour change from silver white to purple to black after phosphorization (Fig. S1†). The corresponding XRD pattern for the precursor (Fig. S2†) shows diffraction peaks at 23.9°, 34.6°, 39.2°, 46.9° and 59.7° (2θ) indexed to the (006), (012), (015), (018) and (110) planes of CoAl-LDH, respectively.47 Upon phosphorization, the CoAl-LDH/NF precursors were successfully converted to Al-CoP/NF (JCPDS no. 29-0497), as proved by the XRD pattern of Al-CoP/NF (Fig. 1a). The SEM images of the CoAl-LDH/NF precursors (Fig. 1b) display the full coverage of the pristine Ni foam (Fig. S3†) with CoAl-LDH nanosheets. After phosphorization, the obtained Al-CoP/NF product still retains its macroporous feature and the cross-linked nanosheets growing vertically on NF (Fig. 1c). The corresponding energy dispersive X-ray spectroscopy (EDS) elemental mapping analysis for Al-CoP/NF demonstrates that Al, Co and P elements are uniformly distributed on a Ni foam skeleton (Fig. 1d). The TEM image of Al-CoP/NF further reveals the nanostructure of Al-CoP nanosheets (Fig. 1e), and the HRTEM image taken from Al-CoP/NF reveals the lattice fringes with an interplanar distance of 0.19 nm assigned to the (211) plane of CoP (Fig. 1f). All these observations further affirm the successful fabrication of the self-supported Al-CoP/NF.
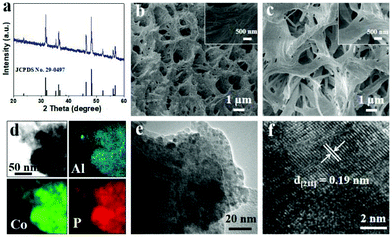 |
| Fig. 1 (a) XRD pattern of Al-CoP/NF. SEM images of (b) CoAl-LDH/NF and (c) Al-CoP/NF. (d) STEM image and EDS elemental mapping of Al-CoP/NF. (e) TEM and (f) HRTEM images of Al-CoP. | |
The full scanning spectrum of XPS, shown in Fig. 2a, reveals the existence of Co, P, Al, O and C elements in the Al-CoP/NF. The high-resolution XPS spectra of Al 2p (Fig. 2b), Co 2p (Fig. 2c) and P 2p (Fig. 2d) were collected for Al-CoP/NF. The peak at 74.7 eV is assigned to Al 2p of Al3+, identifying the successful doping of Al element into the Al-CoP/NF electrode. The Co 2p XPS spectrum in Fig. 2c shows peaks at 778.2 and 795.2 eV, which correspond to the 2p3/2 and 2p1/2 peaks of cobalt phosphide (CoP), respectively.6,33 The peaks at 781.0 and 797.2 eV are assigned to the 2p3/2 and 2p1/2 peaks of oxidized Co (Co3O4), respectively,33,48 which is in full agreement with the distinguished satellite peaks at 784.3 and 802.1 eV of the oxidized Co. The major reason for this is the superficial oxidation of CoP due to air contact. In the P 2p spectrum of the Al-CoP/NF sample, shown in Fig. 2d, the peaks at 129.2 and 130.1 eV correspond, respectively, to the P 2p3/2 and P 2p1/2 of the phosphide (CoP), and another peak at BE of 133.7 eV is assigned to oxidized phosphate species owing to exposure to the air.6 Noticeably, the peaks for Co and P in Al-CoP/NF show negative shifts compared with the peaks for pure CoP,49–51 indicating a strong influence of Al doping on the electronic structure of CoP.52
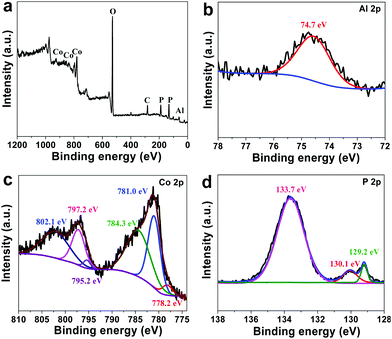 |
| Fig. 2 (a) XPS survey spectrum and (b) Al 2p, (c) Co 2p and (d) P 2p XPS spectra of Al-CoP. | |
3.2. All-pH-value HER performance of Al-CoP/NF
The Al-CoP/NF (Al-CoP loading: 6.51 mg cm−2) was directly used as a flexible working electrode for the HER in 0.5 M H2SO4 with a scan rate of 2 mV s−1 using a typical three-electrode system. In the meantime, the pristine Ni foam, CoP/NF (CoP loading: 6.43 mg cm−2) and a commercial Pt/C electrode deposited on NF were also tested for comparison. According to the linear sweep voltammetry (LSV) curves (Fig. 3a), the pristine Ni foam shows relatively poor HER performance, whereas the self-supported CoP/NF electrode shows high activity for the HER with an overpotential of 91 mV to achieve a current density of 10 mA cm−2. Interestingly, the self-supported Al-CoP/NF electrode exhibits superior HER activity comparable with Pt/C and only needs 51 mV to drive a current density of 10 mA cm−2, implying the positive role of Al-doping in enhancing the electrochemical activity. The performance of Al-CoP/NF compares favorably to most of the reported Co-based phosphide catalysts for the HER in acidic media (Table S1†). Fig. 3b presents the Tafel plots. Pt/C on NF shows a Tafel slope of 33 mV dec−1, consistent with ref. 53. CoP/NF and Al-CoP/NF exhibit Tafel slopes of 74 mV dec−1 and 68 mV dec−1, respectively. Such Tafel slopes indicate that the HER occurs on Al-CoP/NF following a Volmer–Heyrovsky mechanism,54 and at the same time, suggests that Al-CoP/NF has a far superior HER performance than CoP/NF.
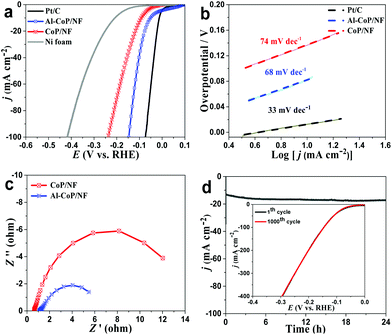 |
| Fig. 3 (a) Polarization curves of Pt/C, Al-CoP/NF, CoP/NF and Ni foam for HER with a scan rate of 2 mV s−1. (b) Tafel plots of CoP/NF, Al-CoP/NF, and Pt/C on NF. (c) The Nyquist plots of CoP/NF and Al-CoP/NF measured at an overpotential of 50 mV. (d) Chronoamperometry curve of Al-CoP/NF at a fixed overpotential of 20 mV for 24 h; inset in (d): polarization curves of Al-CoP/NF before and after 1000 CV cycles (scan rate: 100 mV s−1). All experiments were performed in H2-saturated 0.5 M H2SO4. | |
Fig. 3c shows the electrochemical impedance spectroscopy (EIS) of the self-supported Al-CoP/NF and CoP/NF. The semicircular diameter of the Al-CoP/NF is obviously smaller than that of CoP/NF, implying its higher electron transport rate and faster HER kinetics. According to Bader charge analysis, Al atoms can give 0.45 electron to the adjacent Co atoms, which effectively modulates the electronic interaction between Co and H atoms and makes the electrochemical reaction easier to initiate at low overpotentials.55 After 1000 cyclic voltammetric (CV) cycle scanning, the polarization curves of Al-CoP/NF show negligible decay (Fig. 3d, inset). In addition, the chronoamperometry test further confirms its excellent long-term electrochemical stability (Fig. 3d). The XRD pattern (Fig. S4†) taken after the stability test shows no change in the crystal phase of Al-CoP/NF, and the SEM image (Fig. S5†) taken after the 1000 CV cycle scanning shows that Al-CoP/NF has no significant difference in morphology before and after electrolysis. The outstanding stability of the Al-CoP/NF is attributed to the in situ growth of active components on the substrates, which can prevent the fluctuation of H2 bubbles produced during the HER and maintain the shape of the electrode to ensure that electrochemical tests are executed well. In fact, such in situ growth also influences the HER performance of Al-CoP/NF. According to Fig. S6,† the scratched Al-CoP/NF needs a higher overpotential of 261 mV to drive 10 mA cm−2, suggesting that the electrocatalytic activity of the scratched Al-CoP/NF is inferior to the in situ grown Al-CoP/NF. Noticeably, the different doping amounts of Al atoms also affect the HER performance of Al-CoP/NF. The doping levels in Al-CoP can be controlled by changing the molar ratio of Co to Al in solution for the precursor preparation. According to the LSV curves of the different Al-doped catalysts (Fig. S7a†), the three Al-doped catalysts (Co
:
Al = 10
:
1, 10
:
0.5, 10
:
2) require 51, 62 and 74 mV to achieve 10 mA cm−2, respectively. Consistently, the semicircular diameters of the Al-CoP/NF electrocatalysts (Co
:
Al = 10
:
2, 10
:
0.5, 10
:
1) decrease in turn (Fig. S7b†), suggesting the smallest electrochemical impedance and the fastest electron transport rate of Al-CoP/NF (Co
:
Al = 10
:
1).
Inspired by the excellent self-supported system and remarkable advantages of Al doping, the HER performance of Al-CoP/NF in H2-saturated 1.0 M KOH was further investigated. As seen from the LSV curves of Fig. 4a, Pt/C on NF exhibits excellent HER activity while the pristine Ni foam still shows poor HER performance. And CoP/NF displays high electrocatalytic HER activity, affording a low overpotential of 80 mV to achieve a current density of 10 mA cm−2. In sharp contrast, Al-CoP/NF is very efficient toward the HER with the need for an overpotential of only 66 mV to attain 10 mA cm−2. The HER activity of Al-CoP/NF also compares favorably to the behaviors of most reported Co-based TMP catalysts in alkaline media (Table S2†). Besides, the Tafel slope (Fig. 4b) of 94 mV dec−1 for Al-CoP/NF is lower than that for CoP/NF (112 mV dec−1), suggesting faster kinetics behavior on Al-CoP/NF. The stability of Al-CoP/NF was also examined in alkaline media. As observed in Fig. 4c, no obvious degradation in current density occurs after 1000 CV cycles at a scan rate of 100 mV s−1. Chronopotentiometry testing at a higher current density of 50 mA cm−2 was also performed, and the results (Fig. 4d) show that the activity of Al-CoP/NF can be maintained over a period of 12 h.
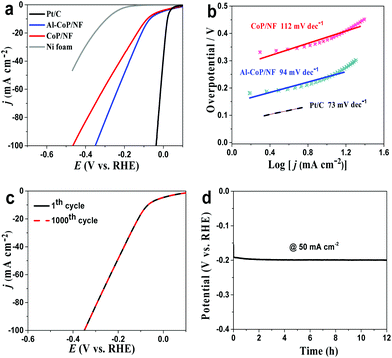 |
| Fig. 4 (a) Polarization curves of Pt/C, Al-CoP/NF, CoP/NF and Ni foam for HER with a scan rate of 2 mV s−1. (b) Tafel plots of CoP/NF, Al-CoP/NF, and Pt/C on NF. (c) Polarization curves of Al-CoP/NF before and after 1000 CV cycles (scan rate: 100 mV s−1). (d) Chronopotentiometry curve of Al-CoP/NF with a constant current density of 50 mA cm−2 for 12 h. All experiments were performed in H2-saturated 1.0 M KOH. | |
Compared with acidic and alkaline media, the HER proceeding in electrocatalysts under neutral conditions presents slower kinetics due to the low proton concentration and larger ohmic loss. As a result, most electrocatalysts exhibit poor HER performance in neutral solution. Noticeably, benefiting from the unique structural advantages and electronic interaction of Al-doping, the Al-CoP/NF electrode exhibits superior HER activity in 1.0 M PBS. As depicted in Fig. 5a, it only requires an overpotential of 83 mV to drive 10 mA cm−2, which far exceeds that of CoP/NF (186 mV) and those of reported electrocatalysts in neutral media (Table S3†). Besides, Al-CoP/NF exhibits a lower Tafel slope (89 mV dec−1) than CoP/NF (119 mV dec−1), as shown in Fig. 5b. Furthermore, the polarization curves are stable after 1000 CV cycles (Fig. 5c) and the chronopotentiometry curve (Fig. 5d) shows that the HER activity of Al-CoP/NF can be maintained over a period of 10 h in neutral media, illustrating the good long-time stability of the Al-CoP/NF electrode towards the HER.
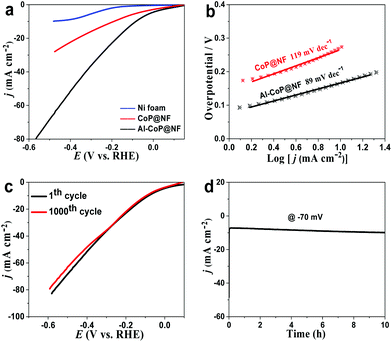 |
| Fig. 5 (a) Polarization curves of Ni foam, CoP/NF and Al-CoP/NF for HER with a scan rate of 2 mV s−1. (b) Tafel plots of CoP/NF and Al-CoP/NF. (c) Polarization curves of Al-CoP/NF before and after 1000 CV cycles (scan rate: 100 mV s−1). (d) Chronoamperometry curve of Al-CoP/NF at a fixed overpotential of −70 mV for 10 h. All experiments were performed in H2-saturated 1.0 M PBS. | |
3.3. Oxygen evolution activity of Al-CoP/NF
The OER performance of Al-CoP/NF was tested in 1.0 M KOH solution at a scan rate of 2 mV s−1. IrO2/NF, CoP/NF, and the pristine Ni foam were also tested for comparison. As shown in the LSV curves of Fig. 6a, the IrO2/NF only needs 1.64 V to achieve a current density of 100 mA cm−2, exhibiting its excellent electrochemical activity for OER. CoP/NF and the pristine Ni foam need voltages of 1.79 and 1.8 V to achieve 100 mA cm−2, respectively, suggesting that their electrochemical performance toward OER is not a patch on that of IrO2/NF. However, Al-CoP/NF needs 1.71 V to achieve 100 mA cm−2, outperforming the CoP/NF and other samples reported recently, and the corresponding results are presented in Table S4.†Fig. 6b presents the Tafel plots for OER. Al-CoP/NF and CoP/NF show Tafel slopes of 69 and 92 mV dec−1, respectively, implying the positive role of Al-doping in promoting the kinetics process of the Al-CoP/NF for OER. Fig. 6c and Fig. S8† show the cyclic voltammograms (CVs) collected in the region of 0.38 to 0.49 V. The double-layer capacitance (Cdl) of the Al-CoP/NF (36.8 mF cm−2) is higher than that of CoP/NF (21.7 mF cm−2), suggesting that the Al-CoP/NF nanosheets can expose more active sites than the CoP/NF. The polarization curves of Al-CoP/NF (Fig. 6d, inset) have no obvious difference between the initial and 1000 CV cycles, implying that a very weak decay of current density occurs. Besides, according to the current–time curve of Fig. 6d, the Al-CoP/NF electrode can stably give a current density of 21 mA cm−2 for at least 24 h. All these results (Fig. 6d) show the long-term electrochemical durability and stability of Al-CoP/NF for OER in 1.0 M KOH. It is noteworthy that Al content has a significant influence on the OER performance. As shown in Fig. S9a†, the three Al-doped catalysts (Co
:
Al=10
:
1, 10
:
0.5, 10
:
2) need 1.71, 1.76 and 1.77 V to achieve 100 mA cm−2, respectively. Moreover, a lower charge transfer resistance value (Fig. S9b†) was observed for Al-CoP/NF (Co
:
Al=10
:
1) (80.6 Ω) compared to Al-CoP/NF (Co
:
Al=10
:
0.5) (101.2 Ω) and Al-CoP/NF (Co
:
Al=10
:
2) (121.1 Ω), suggesting the fastest electron transport rate and the best OER capability of the optimized Al-CoP/NF (Co
:
Al=10
:
1).
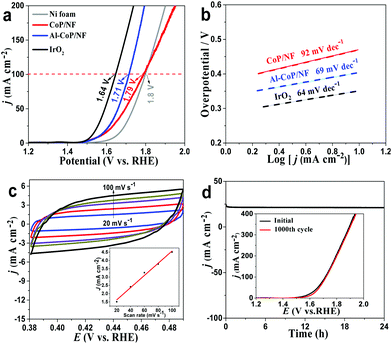 |
| Fig. 6 (a) Polarization curves of IrO2/NF, Al-CoP/NF, CoP/NF and Ni foam for OER with a scan rate of 2 mV s−1. (b) Tafel plots of CoP/NF, Al-CoP/NF, and IrO2 on NF. (c) Cyclic voltammograms of Al-CoP/NF measured at different scan rates ranging from 20 to 100 mV s−1. Inset in (c): a plot of the current density at 0.44 V (vs. RHE) against the scan rate. (d) Chronopotentiometric curve of Al-CoP/NF at 21 mA cm−2 for 24 h. Inset in (d): polarization curves for Al-CoP/NF before and after 1000 CV cycles with a scan rate of 100 mV s−1. All experiments were performed in O2-saturated 1.0 M KOH. | |
Generally, transition metal phosphides have rarely been used in OER, compared to transition metal oxides, hydroxides, and phosphates.56 Herein, the constitution of Al-CoP/NF after OER was elucidated by XPS analysis to determine the actual active centers toward OER. The main XPS peak position of Co 2p presents a negative shift after OER (Fig. S10a†), which might signify a transition of the original Co2+ states to higher oxidation states during OER. Besides, the positions of P 2p peaks changed obviously (Fig. S10b†). The characteristic P 2p peak of phosphide components (130 eV) completely disappears, and the P 2p peak at 133.7 eV can be assigned to phosphate species. Based on the above XPS analysis, the phosphates and cobalt oxides produced on the surface of the electrocatalysts during OER both promote the proton-coupled electron transfer (PCET).57 In addition, the special cross-linked macroporous structure of the self-supported Al-CoP/NF can ensure enriched active sites and accessible active centers during OER. Definitely, Al-doping efficiently modifies the electronic structure of Al-CoP/NF, thus causing an improved charge-transfer ability for OER. Benefiting from the synergistic effect of these factors, the as-obtained Al-CoP/NF exhibits outstanding electrocatalytic activity towards OER.
3.4. Overall water splitting
Based on the results above, it can be reasonably anticipated that the self-supported Al-CoP/NF electrode can be used as an outstanding bifunctional electrocatalyst for overall water splitting to achieve the purpose of electrocatalyzing the HER and OER simultaneously. Fig. 7a shows the polarization curves indicating that the self-supported Al-CoP/NF∥Al-CoP/NF and Pt/C/NF∥IrO2/NF need 1.63 and 1.54 V to achieve a current density of 10 mA cm−2, respectively. Although the performance of the Al-CoP/NF is slightly inferior to that of the Pt/C/NF∥IrO2/NF electrodes, it is comparable with most of the recently reported electrocatalysts for overall water splitting (Table S5†). Obviously, a lot of hydrogen and oxygen bubbles can be observed in Fig. 7b (inset). As shown in Fig. 7b, the Al-CoP/NF electrode can maintain the catalytic activity for at least 24 h (the voltage stabilizes around 1.64 V eventually). Moreover, the polarization curves for Al-CoP/NF∥Al-CoP/NF electrodes before and after 1000 cycles (Fig. S11†) exhibit a negligible loss in current density. All these results (Fig. 7b and S11†) show the excellent stability and good durability of this electrode for overall water splitting.
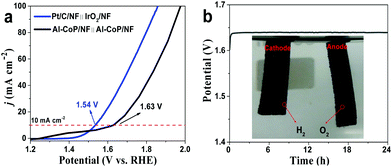 |
| Fig. 7 (a) Polarization curves of Al-CoP/NF∥Al-CoP/NF and Pt/C/NF∥IrO2/NF in a two-electrode alkaline water electrolysis with a scan rate of 5 mV s−1. (b) Chronopotentiometric curve of Al-CoP/NF∥Al-CoP/NF with a constant current density of 20 mA cm−2 for 24 h. Inset in (b): a representative photograph showing the generation of H2 (left) from the cathode and O2 (right) from the anode during overall water electrolysis. All experiments were carried out in 1.0 M KOH. | |
3.5. Discussion
The observation of a high catalytic performance of the Al-CoP/NF electrode for HER, OER and overall water splitting can be rationalized to the following features: first, the superior conductivity, good stability and strong flexibility of the self-supported substrate can effectively promote charge transfer and avoid the collapse of the structure of the catalyst and shedding of catalyst nanoparticles during the electrocatalytic reaction. Therefore, the flexible 3D integrated frame of the Ni foam with abundant active boundary sites enhances the stability and activity of the catalysts (Fig. 3d, 4d, 5d, 6d and 7b). Moreover, the direct in situ growth of cross-linked Al-CoP nanosheets on Ni foam prevents the utilization of high-cost polymeric binders and other extra conductive additives, thereby enhancing the electron-transfer rate between the catalyst nanoparticles and the electrolyte (Fig. S6†).
Second, the fascinating cross-linked porous characteristics of Al-CoP/NF can provide enriched accessible electrocatalytic active boundary sites, as proved by the electrochemical double layer capacitance (Cdl) measurements. The Cdl of the Al-CoP/NF (36.8 mF cm−2) (Fig. 6c) is much higher than those of CoP/NF (21.7 mF cm−2) (Fig. S8†) and pristine Ni foam, suggesting that such kinds of unique cross-linked porous structures and doping with metal elements can evidently enhance the electrochemically active surface area of Al-CoP/NF and provide superior HER and OER ability.
Lastly, the electrocatalytic reaction kinetics of Al-CoP/NF for HER and OER is significantly promoted due to the doping effect of Al atoms. According to the Bader charge analysis, Al atoms can offer 0.45 electron to the adjacent Co atoms, thereby the electronic interaction between Co and H atoms is weakened.55 Al-induced partial charge-transfer effectively modifies the electronic structure of CoP, leading to a more thermo-neutral hydrogen adsorption free energy (ΔGH*),55,58 which evidently improves the electrocatalytic activity of the as-fabricated Al-CoP/NF for HER and OER. According to the linear sweep voltammetry (LSV) curves (Fig. 3a, 4a, 5a and 6a), the HER and OER activities of Al-CoP/NF are better than that of CoP/NF, implying the positive role of Al-doping in enhancing the electrochemical activity. In addition, electrochemical impedance spectroscopy (EIS) tests show that the semicircular diameter of Al-CoP/NF is obviously smaller than that of CoP/NF (Fig. 3c), suggesting its higher electron transport rate and faster electrocatalytic reaction kinetics. In a word, the above-mentioned structural advantages and Al-doping strategy endow Al-CoP/NF with superior electrocatalytic performance.
4. Conclusions
In summary, cross-linked Al-CoP nanosheets have been developed on Ni foam (Al-CoP/NF) as efficient HER and OER electrocatalysts. The substitution of Al into CoP has a heavy influence on its HER performance and the resulting electrocatalyst requires overpotentials of 51, 66 and 83 mV to drive a current density of 10 mA cm−2 in acidic, alkaline and neutral media. Moreover, the Al-CoP/NF electrode shows excellent OER performance in alkaline solutions, with a low overpotential of 330 mV, driving a current density of 10 mA cm−2. Furthermore, as an excellent bifunctional catalyst, the Al-CoP/NF electrode needs 1.63 V to achieve a current of 10 mA cm−2 for overall water splitting, outperforming most of the reported transition metal Co-based catalysts. The remarkable electrochemical performance of Al-CoP/NF could be mainly ascribed to structural advantages such as a 3D conductive framework, fascinating porous characteristics, and Al doping. This work not only provides us with an advanced bifunctional electrode for overall water splitting but also paves an alternative pathway for designing and developing efficient multifunctional Al-doped transition metal phosphide (TMP) electrocatalysts for energy storage and sensing applications.
Conflicts of interest
There are no conflicts of interest to declare.
Acknowledgements
This work was supported by the National Natural Science Foundation of China (21421001, 21573115, and 21766026), the Natural Science Foundation of Tianjin (17JCYBJC17100), the 111 project (B12015), the Fundamental Research Funds for the Central Universities (63185015), and the Foundation of State Key Laboratory of High-efficiency Utilization of Coal and Green Chemical Engineering (2017-K13).
Notes and references
- M. S. Dresselhaus and I. L. Thomas, Nature, 2001, 414, 332–337 CrossRef CAS PubMed.
- J. Chow, R. J. Kopp and P. R. Portney, Science, 2003, 302, 1528–1531 CrossRef.
- J. A. Turner, Science, 2004, 305, 972–974 CrossRef CAS.
- H. Shi, H. Liang, F. Ming and Z. Wang, Angew. Chem., Int. Ed., 2017, 129, 588–592 CrossRef.
- D. Merki and X. Hu, Energy Environ. Sci., 2011, 4, 3878–3888 RSC.
- Y. P. Zhu, Y. P. Liu, T. Z. Ren and Z. Y. Yuan, Adv. Funct. Mater., 2015, 25, 7337–7347 CrossRef CAS.
- Y. Ji, L. Yang, X. Ren, G. Cui, X. Xiong and X. Sun, ACS Sustainable Chem. Eng., 2018, 6, 11186–11189 CrossRef CAS.
- L. Yang, H. Qi, C. Zhang and X. Sun, Nanotechnology, 2016, 27, 23LT01 CrossRef.
- J. Ren, Z. Hu, C. Chen, Y. Liu and Z. Yuan, J. Energy Chem., 2017, 26, 1196–1202 CrossRef.
- P. Jiang, Q. Liu and X. Sun, Nanoscale, 2014, 6, 13440–13445 RSC.
- Q. Liu, S. Gu and C. M. Li, J. Power Sources, 2015, 299, 342–346 CrossRef CAS.
- C. Deng, F. Ding, X. Li, Y. Guo, W. Ni, H. Yan, K. Sun and Y. M. Yan, J. Mater. Chem. A, 2016, 4, 59–66 RSC.
- Z. Pu, S. Wei, Z. Chen and S. Mu, Appl. Catal., B, 2016, 196, 193–198 CrossRef CAS.
- C. C. Hou, Q. Q. Chen, C. J. Wang, F. Liang, Z. Lin, W. F. Fu and Y. Chen, ACS Appl. Mater. Interfaces, 2016, 8, 23037–23048 CrossRef CAS.
- Y. Liang, Q. Liu, A. M. Asiri, X. Sun and Y. Luo, ACS Catal., 2014, 4, 4065–4069 CrossRef CAS.
- M. Pi, T. Wu, D. Zhang, S. Chen and S. Wang, Nanoscale, 2016, 8, 19779–19786 RSC.
- Q. Liu, J. Tian, W. Cui, P. Jiang, N. Cheng, A. M. Asiri and X. Sun, Angew. Chem., Int. Ed., 2014, 53, 6710–6714 CrossRef CAS.
- H. Zhao and Z. Y. Yuan, Catal. Sci. Technol., 2017, 7, 330–347 RSC.
- E. J. Popczun, C. G. Read, C. W. Roske, N. S. Lewis and R. E. Schaak, Angew. Chem., Int. Ed., 2014, 53, 5427–5430 CrossRef CAS.
- J. T. Ren, Y. J. Song and Z. Y. Yuan, J. Energy Chem., 2018 DOI:10.1016/j.jechem.2018.07.006.
- Q. Liu, A. M. Asiri and X. Sun, Electrochem. Commun., 2014, 49, 21–24 CrossRef CAS.
- Q. Luo, M. Peng, X. Sun and A. M. Asiri, Catal. Sci. Technol., 2016, 6, 1157–1161 RSC.
- X. W. Lv, Z. P. Hu, H. Zhao, Y. P. Liu and Z. Y. Yuan, Prog. Chem., 2018, 30, 947–957 Search PubMed.
- H. Zhao, Y. P. Zhu and Z. Y. Yuan, Eur. J. Inorg. Chem., 2016, 2016, 1916–1923 CrossRef CAS.
- S. Chen, J. Duan, M. Jaroniec and S. Z. Qiao, Adv. Mater., 2014, 26, 2925–2930 CrossRef CAS.
- X. Wang, Y. V. Kolen'ko, X. Q. Bao, K. Kovnir and L. Liu, Angew. Chem., Int. Ed., 2015, 127, 8306–8310 CrossRef.
- J. Duan, S. Chen, M. Jaroniec and S. Z. Qiao, ACS Nano, 2015, 9, 931–940 CrossRef CAS.
- N. Bai, Q. Li, D. Mao, D. Li and H. Dong, ACS Appl. Mater. Interfaces, 2016, 8, 29400–29407 CrossRef CAS.
- A. Han, H. Chen, H. Zhang, Z. Sun and P. Du, J. Mater. Chem. A, 2016, 4, 10195–10202 RSC.
- J. T. Ren, G. G. Yuan, C. C. Weng and Z. Y. Yuan, ACS Sustainable Chem. Eng., 2017, 6, 707–718 CrossRef.
- N. Kornienko, J. Resasco, N. Becknell, C. M. Jiang, Y. S. Liu, K. Nie, X. Sun, J. Guo, S. R. Leone and P. Yang, J. Am. Chem. Soc., 2015, 137, 7448–7455 CrossRef CAS PubMed.
- M. S. Faber, R. Dziedzic, M. A. Lukowski, N. S. Kaiser, Q. Ding and S. Jin, J. Am. Chem. Soc., 2014, 136, 10053–10061 CrossRef CAS PubMed.
- J. T. Ren, G. G. Yuan, C. C. Weng and Z. Y. Yuan, Electrochim. Acta, 2018, 261, 454–463 CrossRef CAS.
- Y. Y. Ma, C. X. Wu, X. J. Feng, H. Q. Tan, L. K. Yan, Y. Liu, Z. H. Kang, E. B. Wang and Y. G. Li, Energy Environ. Sci., 2017, 10, 788–798 RSC.
- C. Zhu, S. Fu, B. Z. Xu, J. Song, Q. Shi, M. H. Engelhard, X. Li, S. P. Beckman, J. Sun and D. Du, Small, 2017, 13, 1700796 CrossRef.
- Y. Pan, N. Yang, Y. Chen, Y. Lin, Y. Li, Y. Liu and C. Liu, J. Power Sources, 2015, 297, 45–52 CrossRef CAS.
- R. Zhang, C. Zhang and W. Chen, J. Mater. Chem. A, 2016, 4, 18723–18729 RSC.
- J. Kibsgaard and T. F. Jaramillo, Angew. Chem., Int. Ed., 2014, 53, 14433–14437 CrossRef CAS.
- M. E. Björketun, A. S. Bondarenko, B. L. Abrams, I. Chorkendorff and J. Rossmeisl, Phys. Chem. Chem. Phys., 2010, 12, 10536–10541 RSC.
- J. K. Nørskov, T. Bligaard, A. Logadottir, J. Kitchin, J. G. Chen, S. Pandelov and U. Stimming, J. Electrochem. Soc., 2005, 152, J23–J26 CrossRef.
- J. Greeley, J. K. Nørskov, L. A. Kibler, A. M. El-Aziz and D. M. Kolb, ChemPhysChem, 2006, 7, 1032–1035 CrossRef CAS.
- D. Kang, T. W. Kim, S. R. Kubota, A. C. Cardiel, H. G. Cha and K.-S. Choi, Chem. Rev., 2015, 115, 12839–12887 CrossRef CAS.
- R. Parsons, Trans. Faraday Soc., 1958, 54, 1053–1063 RSC.
- T. Liu, X. Ma, D. Liu, S. Hao, G. Du, Y. Ma, A. M. Asiri, X. Sun and L. Chen, ACS Catal., 2016, 7, 98–102 CrossRef.
- J. Wang, X. Ma, F. Qu, A. M. Asiri and X. Sun, Inorg. Chem., 2017, 56, 1041–1044 CrossRef CAS PubMed.
- T. Y. Ma, S. Dai, M. Jaroniec and S. Z. Qiao, J. Am. Chem. Soc., 2014, 136, 13925–13931 CrossRef CAS PubMed.
- J. B. Han, J. Lu, M. Wei, Z. L. Wang and X. Duan, Chem. Commun., 2008, 5188–5190 RSC.
- H. Jin, J. Wang, D. Su, Z. Wei, Z. Pang and Y. Wang, J. Am. Chem. Soc., 2015, 137, 2688–2694 CrossRef CAS.
- J. Huang, Y. Li, Y. Xia, J. Zhu, Q. Yi, H. Wang, J. Xiong, Y. Sun and G. Zou, Nano Res., 2017, 10, 1010–1020 CrossRef CAS.
- P. Jiang, Q. Liu, C. Ge, W. Cui, Z. Pu, A. M. Asiri and X. Sun, J. Mater. Chem. A, 2014, 2, 14634–14640 RSC.
- M. Li, X. Liu, Y. Xiong, X. Bo, Y. Zhang, C. Han and L. Guo, J. Mater. Chem. A, 2015, 3, 4255–4265 RSC.
- H. Du, L. Xia, S. Zhu, F. Qu and F. Qu, Chem. Commun., 2018, 54, 2894–2897 RSC.
- E. J. Popczun, J. R. McKone, C. G. Read, A. J. Biacchi, A. M. Wiltrout, N. S. Lewis and R. E. Schaak, J. Am. Chem. Soc., 2013, 135, 9267–9270 CrossRef CAS.
- B. Conway and B. Tilak, Electrochim. Acta, 2002, 47, 3571–3594 CrossRef CAS.
- R. Zhang, C. Tang, R. Kong, G. Du, A. M. Asiri, L. Chen and X. Sun, Nanoscale, 2017, 9, 4793–4800 RSC.
- J. Ryu, N. Jung, J. H. Jang, H. J. Kim and S. J. Yoo, ACS Catal., 2015, 5, 4066–4074 CrossRef CAS.
- S. Cobo, J. Heidkamp, P. A. Jacques, J. Fize, V. Fourmond, L. Guetaz, B. Jousselme, V. Ivanova, H. Dau and S. Palacin, Nat. Mater., 2012, 11, 802 CrossRef CAS.
- H. Du, L. Xia, S. Zhu, F. Qu and F. Qu, Chem. Commun., 2018, 54, 2894–2897 RSC.
Footnote |
† Electronic supplementary information (ESI) available. See DOI: 10.1039/c8qi01026a |
|
This journal is © the Partner Organisations 2019 |
Click here to see how this site uses Cookies. View our privacy policy here.