DOI:
10.1039/C8QM00596F
(Research Article)
Mater. Chem. Front., 2019,
3, 484-491
Fast-response ionogel humidity sensor for real-time monitoring of breathing rate†
Received
20th November 2018
, Accepted 3rd January 2019
First published on 9th January 2019
Abstract
Ionogel-based chemoresistive humidity sensors have been successfully fabricated through ionothermal assembly of ionic liquids into a silica network. Our results demonstrated that ionogel sensors with different types of anions (Br−, BF4−, and PF6−) exhibited good humidity sensitivity, low humidity hysteresis, and good reproducibility. In particular, the response and recovery times of the SiO2/[Bmim]Br-based sensor reached 3 s and 10 s, respectively, which were considerably shorter than those of other SiO2-based humidity sensors. The improved sensitivity characteristics of SiO2/[Bmim]Br can be attributed to its high conductivity and hydrophilicity, resulting in fast ion transfer using Br− and H3O+ as charge carriers under humid conditions. Furthermore, this ionogel sensor showed fast and substantial changes in impedance with changing humidity for real-time monitoring of human breath. These findings are expected to provide opportunities for the design and fabrication of high-performance sensors for online humidity monitoring.
1. Introduction
Monitoring humidity with a reliable sensor is now essential to ensure suitable moisture conditions in various fields, such as medicine, the automobile industry, manufacturing, and agriculture. To measure humidity in dynamic situations, highly responsive sensors are often required, mainly depending on the sensing materials.1 Therefore, humidity sensors based on polymers,2 semiconductors,3 and inorganic/organic hybrids4 have been developed with the aim of optimizing performance. Among these, inorganic/organic hybrids, particularly SiO2-based hybrids, have attracted much attention and been widely investigated owing to their good stability, convenient fabrication process, and wide operating temperature range.5 For example, Korposh and coworkers reported an optical fibre sensor using poly(allylamine hydrochloride)/silica (PAH/SiO2) as sensing materials.6 This hybrid sensor showed high humidity sensitivity, and was successfully used to monitor humidity in ventilator care equipment. However, owing to the low ionic conductivity of silica, this type of sensor often suffers from a slow response and recovery behaviour (Table S1, ESI†), which greatly limits their further practical application. Therefore, achieving SiO2-based humidity sensors with a dynamic response capability in rapid succession remains challenging.7
Ionic liquids (ILs) are completely composed of cations and anions, and can be considered as salts that are liquid at room temperature. ILs usually possess several unique properties, such as high intrinsic conductivity, high thermal stability, and a good solvating ability.8 Furthermore, the physicochemical properties of ILs are mainly determined by the cation–anion pairs. Therefore, ILs can be referred to as “designed media” with properties that can be tuned by adjusting the ion pairs, which makes them ideal sensing materials for various sensing platforms.9 However, for practical applications, the direct use of ILs as liquids is inconvenient. Therefore, it is necessary to solidify ILs in devices while maintaining their distinctive performances.10 To this end, ionogels are a class of hybrid materials that can encapsulate or immobilize ILs in a three-dimensional network, including organic, inorganic, and organic/inorganic hybrid materials.11 Furthermore, owing to the confinement effect of nanopores in the host matrix, ionogels with high ionic conductivity become more sensitive to environmental stimulation.12 Therefore, ionogel-based chemical sensors are a promising class of highly responsive sensors, especially for the fast detection of trace analytes.
In this study, we successfully fabricated ionogel-based chemoresistive humidity sensors through a facile route at room temperature, avoiding the conventional high temperature process (Scheme 1a and b). To confine ILs in solid matrices, ionogels were encapsulated in a silica gel network using a sol–gel method. Herein, 1-n-butyl-3-methylimidazolium bromide ([Bmim]Br), 1-n-butyl-3-methylimidazolium tetrafluoroborate ([Bmim]BF4), and 1-n-butyl-3-methlimidazolium hexafluorophosphate ([Bmim]PF6) were selected for their high ionic conductivities and different hydrophilicities. Their affinity for water molecules primarily depended on the anions, with the hydrophilicity known to follow the order Br− > BF4− > PF6−. The conductivity of the ionogel sensors varied sensitively over a wide relative humidity (RH) range, with fast response and recovery times. Specifically, hydrophilic [Bmim]Br in the hybrid material played an important role in facilitating ion diffusion behaviour and charge carrier transfer, resulting in rapid response (3 s) and recovery (10 s) properties with moisture. This fast responsive behaviour was significantly superior to that of other SiO2-based humidity sensors (Table S1, ESI†). Accordingly, the corresponding mechanism was investigated using complex impedance plots. Furthermore, this ionogel sensor was confirmed to show rapid substantial impedance changes with changing humidity for the real-time monitoring of human breath owing to its high sensitivity (Scheme 1c). Therefore, this ionogel sensor is expected to be implemented meritoriously to continuously monitor humidity.
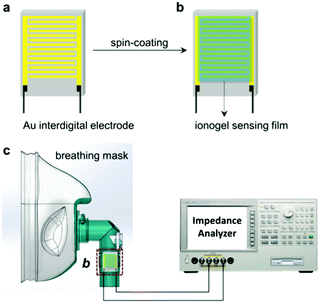 |
| Scheme 1 (a) Illustration of the Au interdigital electrode. (b) Fabrication of humidity sensor by spin-coating ionogel on the interdigital electrode. (c) Illustration of real-time monitoring of human breath using ionogel-based humidity sensor. | |
2. Experimental section
2.1 Materials
1-n-Butyl-3-methylimidazolium bromide ([Bmim]Br), 1-n-butyl-3-methylimidazolium tetrafluoroborate ([Bmim]BF4), and 1-n-butyl-3-methlimidazolium hexafluorophosphate ([Bmim]PF6) were obtained from Lanzhou Greenchem ILS, LICP. CAS. China. Other chemicals were purchased and used without further purification. Water was deionized before use.
2.2 Preparation and measurement of humidity sensors
The humidity sensors were fabricated by spin-coating the SiO2/IL hybrid ionogels onto pre-cleaned interdigitated electrodes. Alumina ceramic was used as the substrate owing to its high dielectric strength and excellent chemical stability. The interdigitated electrodes, composed of titanium (10 nm) and gold (80 nm) with a line width of 80 μm, were fabricated by sputtering. In a typical fabrication process, the ionogels were first prepared using a nonhydrolytic sol–gel route. A mixture of tetraethylorthosilicate (TEOS) and methyltrimethoxysilane (MTMS) was added to ILs dissolved in formic acid (FA) solution (molar ratio, FA
:
TEOS
:
MTMS
:
IL = 7.8
:
0.5
:
1
:
0.5). The sensors were then fabricated by dropping the above mixture solution (4.0 μL) onto the pre-cleaned interdigitated electrodes, followed by gelatinization at room temperature for 20 h.
A schematic diagram of the experimental setup used for humidity sensing measurements is shown in Scheme S1 (ESI†). In a typical test procedure, the humidity sensing properties were investigated by exposing the ionogel-based sensor to various relative humidity (RH) levels, which was achieved using several saturated aqueous solutions. In general, a saturated aqueous solution in a closed vessel at a stable temperature can provide stable and controllable RH levels in their equilibrium states. Several 250 mL closed vessels containing different saturated salt solutions, including LiCl, MgCl2, K2CO3, NaBr, KI, KCl, and K2SO4, were used as sources of 11%, 33%, 43%, 59%, 75%, 85%, and 98% RH, respectively. The impedance response of the ionogel-based sensor was measured using an impedance analyser (Agilent 4294, 40 Hz to 100 MHz), which was connected to a PC to collect and analyse data. The applied voltage amplitude was 500 mV, and the measuring temperature was about 25 °C. The sensor response as a function of RH was measured by exposing the sensor to different RH levels inside the closed vessels for the intake/outtake of water molecules. For real-time monitoring of the human breathing rate, we employed a healthy male as a volunteer, and variations in the RH value were monitored by holding the ionogel humidity sensor a distance of about 2–3 cm from the nose in a breathing mask while breathing normally (Scheme 1c).
2.3 Characterization
The ionogel products were characterized by X-ray diffraction (XRD), scanning electron microscopy (SEM), and Fourier transform infrared spectroscopy (FT-IR) measurements. XRD measurements were performed on a Rigaku D/max 2500 diffractometer with Cu Kα radiation (λ = 0.154056 nm) at V = 40 kV and I = 150 mA, with a scanning speed of 6° min−1. The morphology and size of the samples were characterized by SEM (Zeiss SUPRA 55) combined with energy dispersive X-ray spectroscopy (EDS, Oxford). FT-IR spectra of the samples were recorded at room temperature with a KBr pellet on a VECTOR-22 (Bruker) spectrometer ranging from 400 to 4000 cm−1.
3. Results and discussion
3.1 Structure characterization
To determine the phase structures, XRD measurement of pure SiO2 and ionogels with different ILs was conducted, as shown in Fig. S1 (ESI†). All patterns were characteristic of amorphous SiO2 networks. The peaks at around 22° were assigned to the coherent diffracting regions within the siliceous networks.13Fig. 1a and b show representative SEM images and the corresponding X-ray elemental maps, detected by EDS, of the as-prepared ionogel (SiO2/[Bmim]Br), respectively. The ionogels consisted of spherical aggregates with average diameters of 1–1.5 μm. Furthermore, the spherical ionogels were connected to form the network structure through ILs anchored to the SiO2 surface. This unique structure was beneficial for ion and mass transfer processes. The elements detected in the ionogel included silicon, carbon, nitrogen, and bromine. The corresponding mappings indicated fairly homogeneous elemental distributions (Fig. 1b), confirming that the IL was dispersed homogeneously within entire ionogel networks. Conversely, only irregularly shaped nanoparticles were obtained in the absence of ILs, indicating that ILs might act as the structure directing agent in ionogel formation (Fig. S2, ESI†). Specifically, the ILs tended to form self-assembly superstructures on the SiO2 surface through hydrogen bonding and additional π–π interactions. This self-organization of ionogels was demonstrated by the FT-IR results, as shown in Fig. 1c. The FT-IR spectrum of pure [Bmim]Br showed bands at 3145, 3102, 2985, 1569, 845, and 630 cm−1. Among these, the bands at 3145 and 3102 cm−1 stemmed from the C–H vibrations of cyclic [Bmim]+, while the bands at 2985, 845, and 630 cm−1 were assigned to aliphatic C–H vibrations from the imidazolium cation. Furthermore, the band at 1569 cm−1 was indexed to C–C and C–N bending vibrations in the aliphatic ring.14 Compared with the bands of pure [Bmim]Br, bands related to the imidazolium ring broadened in the ionogel, indicating π–π stacking of the imidazolium ring on the SiO2 surface.15
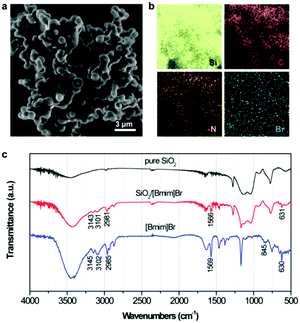 |
| Fig. 1 (a) SEM image and (b) corresponding elemental mappings of SiO2/[Bmim]Br ionogel. (c) FT-IR spectra of pure SiO2, SiO2/[Bmim]Br ionogel, and [Bmim]Br. | |
3.2 Humidity performances
Initially, the pristine SiO2 sensor was fabricated and measured at different RHs. Fig. S4a (ESI†) shows the dependence of impedance on RH from 40 Hz to 10 MHz. Clearly, the pristine SiO2 sensor showed a small response in the whole RH range. When introducing ILs into the hybrid materials, the impedance of all ionogels decreased with increasing RH. However, their response properties to humidity were different, and closely related to their hydrophilicity. SiO2/[Bmim]Br showed lower impedance than the other two ionogels at 50 kHz owing to its high hydrophilicity, and displayed the highest humidity sensitivity (Fig. 2). Generally, the measuring frequency plays an important role in the humidity properties, and the value of impedance for a humidity sensor can be expressed as follows:16
where R is a resistor element and C is a capacitor element in an equivalent circuit for a humidity sensor, and ω (ω = 2πf, f is the working frequency) is the angle frequency. In a low-frequency range (from 40 to 100 kHz), the frequency greatly influences the impedance value, especially at a low RH. At this stage, few water molecules are adsorbed and polarized on the ionogel film, resulting in large resistances and very small values of 1/R2. Therefore, the total impedance, Z, mainly relied on (ωC)2, which is closely related to the frequency. Conversely, the resistances of ionogels became small in the presence of large numbers of adsorbed water molecules at high RH. In this case, the value of 1/R2 rather than (ωC)2 dominates the total resistance of the ionogels, and the frequency plays a less important role in changes in impedance value. In the high-frequency range (>100 kHz), the impedance curves of all three ionogel sensors became flat and independent of RH. As it is difficult for the polarization of water molecules to follow the change in direction of the electrical field, the adsorbed water molecules could not be polarized under these conditions. At this stage, the impedance is mainly dominated by the geometric capacitance of the sensor. Taking into account that the capacitance and dielectric constant of the sensor are small, the sensor becomes independent of RH. From Fig. S4 (ESI†), the curve of impedance vs. RH for SiO2/[Bmim]Br at 100 kHz showed the best linearity. The corresponding curves for SiO2/[Bmim]BF4 and SiO2/[Bmim]PF6 showed the best linearity at 2.5 kHz and 100 Hz, respectively. To obtain a comprehensive comparison, we selected these optimal frequencies as working conditions for subsequent discussions.
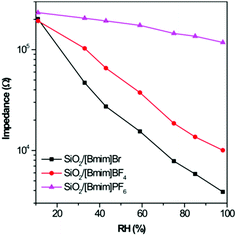 |
| Fig. 2 Impedance dependence of different ionogel-based sensors on RH at 50 kHz. | |
To act as ideal humidity sensors, the ionogel sensors should have high sensitivity, low hysteresis, fast responses and recovery properties, and repeatability, among other properties. Accordingly, we determined the related criteria of ionogel humidity sensors. The dynamic response tests of the ionogel based sensors from 11% RH to different RHs are shown in Fig. 3a–c. All ionogel based sensors exhibited good reversibility during the step changes in humidity. The hysteresis diagrams of the ionogel sensors are shown in Fig. 3d. Usually, the humidity hysteresis is evaluated from the difference between the logarithmic impedance values in the adsorption and desorption processes in the working range. The maximum hysteresis for the three types of ionogel sensors was less than 2% RH in the range of 11–97% RH, showing the good reliability of the ionogel humidity sensors. Furthermore, the SiO2/[Bmim]Br sensor was tested repeatedly every 3 days at fixed humidity levels for 30 days to examine the long-term stability (Fig. S5, ESI†). The sensor impedance fluctuated slightly over time, with the data showing good consistency with a low impedance variation (<5%) in each humidity region.
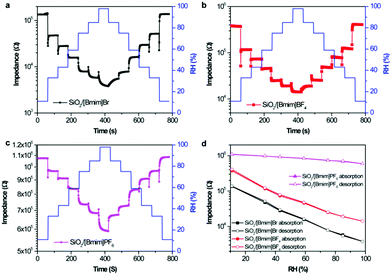 |
| Fig. 3 Real-time frequency response of ionogel-based sensors as the RH was increased from 11% to 98% RH and returned to the initial state: (a) SiO2/[Bmim]Br, (b) SiO2/[Bmim]BF4, and (c) SiO2/[Bmim]PF6. (d) Humidity hysteresis of different ionogel-based sensors. | |
The repeatability of the ionogel sensors was evaluated by exposing the sensors to fast RH changes between 11% and 98% RH for five cycles, as shown in Fig. 4. All ionogel humidity sensors possessed good repeatability, but different response and recovery characteristics. The response and recovery times are another two key factors in evaluating the sensitivity of a humidity sensor, and are defined as the times taken by a sensor to achieve 90% of the total impedance change in the adsorption and desorption processes, respectively. The response times for SiO2/[Bmim]Br and SiO2/[Bmim]BF4 were about 3 s and 2 s, respectively (Fig. 5). This fast response behaviour indicated that ILs anchored on the SiO2 surface facilitated the adsorption of water molecules. The recovery time for SiO2/[Bmim]Br was about 10 s, which was shorter than that of SiO2/[Bmim]BF4 (about 20 s). Furthermore, the SiO2/[Bmim]PF6-based sensor showed long response and recovery times (16 s and 18 s, respectively), because the hydrophobic property of [Bmim]PF6 resulted in less active sites for water molecules to anchor to the surface. The charge carrier in SiO2/[Bmim]PF6 was mainly solvated PF6− instead of H+ or H3O+. Its large volume and low ion conductivity led to slow response and recovery properties. These results showed that the response and recovery behaviours of the ionogel sensors can be designed by simply adjusting the anions in the ionogels. As the response and recovery times of the SiO2/[Bmim]Br sensor were very small throughout the RH range, this material has potential applications in real-time RH monitoring.
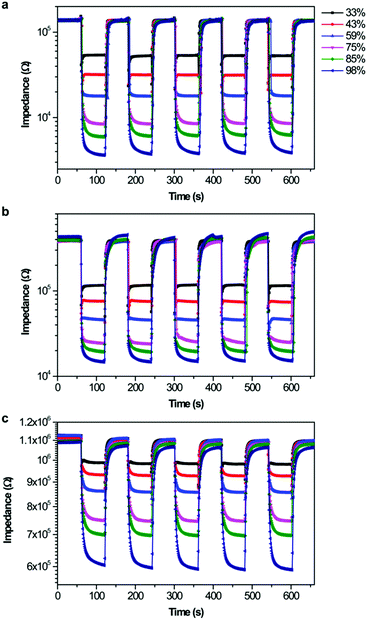 |
| Fig. 4 Repeatability of ionogel-based sensor from 11% to 98% RH: (a) SiO2/[Bmim]Br, (b) SiO2/[Bmim]BF4, and (c) SiO2/[Bmim]PF6. | |
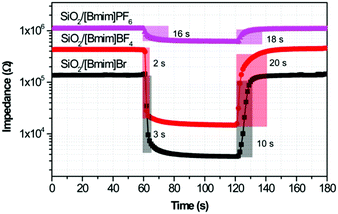 |
| Fig. 5 Response and recovery properties of various ionogel-based sensors from 11% to 98% RH. | |
3.3 Possible mechanism
Based on the above results, the introduction of ILs into SiO2-based hybrid materials greatly enhanced the conductivity and affinity for water molecules. To investigate the humidity sensitive mechanism of ionogels, complex impedance plots of the sensors were performed from 40 Hz to 10 MHz at different RH levels, as shown in Fig. 6. The real part (Z′, horizontal axis) and imaginary part (Z′′, vertical axis) are magnified on the same plane to more conveniently compare several complex impedance plots. Interestingly, all complex impedance plots at different RH levels were composed of a semicircle at the high frequency range and a line at the low frequency range. This phenomenon indicated that the sensing mechanism of the ionogel sensor was represented not only by the intrinsic impedance of the ionogels, but also by ion diffusion (or electrolytic conductivity) at the interface between the ionogel film and electrode.17 Considering that only a few water molecules were adsorbed on the surface of the ionogel film under low-humidity conditions (11% RH), it is extremely difficult to produce free protons (H+) ionized from H2O or the continuous water layer. Therefore, ion diffusion mainly stems from the conduction of the ionogel anions (Br−, BF4−, and PF6−) owing to the high ion conductivity of the ILs. As the RH increased, the ionogel sensors exhibited different humidity features owing to their affinity for water molecules. For hydrophilic SiO2/[Bmim]Br and moderately hydrophilic SiO2/[Bmim]BF4, more water molecules were adsorbed onto the ionogel films, resulting in full-coverage water layers. In this situation, the adsorbed water molecules are easily ionized to form hydronium ions (H3O+) or protons as charge carriers under an electrostatic field. According to the ion transfer mechanism of Grotthuss,18 H2O + H3O+ → H3O+ + H2O, charge transport occurs among adjacent water molecules through proton release from H3O+. This fast ion transfer can greatly decrease the impedance of the SiO2/[Bmim]Br and SiO2/[Bmim]BF4 films. However, forming a continuous water layer on the hydrophobic SiO2/[Bmim]PF6 film is difficult, even at high RH. Therefore, the changes in resistance as RH increased were relatively small compared with the other two ionogel films.
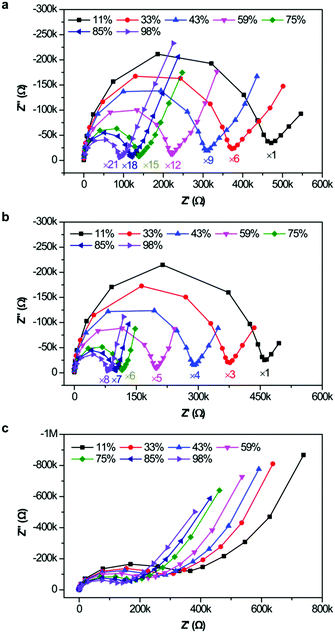 |
| Fig. 6 Complex impedance plots obtained from 40 Hz to 10 MHz at various RH levels: (a) SiO2/[Bmim]Br, (b) SiO2/[Bmim]BF4, and (c) SiO2/[Bmim]PF6. | |
Furthermore, analysis of the complex impedance plots was performed by fitting the experimental results according to an equivalent circuit (Fig. S6, ESI†) with Z-View software. The adopted equivalent circuit consisted of parallel and series combinations of resistances (R1 and Rs), constant phase elements (ZCPE), and Warburg impedance (Zw). The simulated results obtained through the equivalent circuit are also shown in Fig. 7, with all simulated curves fitting well with the experimental results (Fig. S7, ESI†). The serial resistance, Rs, is related to the surrounding region of the electrode, which is weakly dependent on RH (Fig. 7a). The equivalent circuit element of resistor R1 in parallel with the CPE simulates the sensing mechanism of the semicircle, where resistance R1 represents the bulk resistance of the sensing film and CPE characterizes the influence of polarization in the sensing film. The value of R1 for SiO2/[Bmim]Br decreased linearly with increasing RH (Fig. 7b), which was attributed to the improved electrical conductance of the SiO2/[Bmim]Br sensing film. It was reasonable for the swelling effect to become stronger when the humidity increased, leading to a decrease in IL viscosity. Furthermore, the relationship between conductivity (η) and viscosity (σ) of the ILs can be represented by the following Walden equation: lg
σ = lg
k + α
lg
η−1, where k is a temperature-dependent constant, and α is the fitting parameter. Therefore, the conductivity of ILs was inversely proportional to viscosity, and the intrinsic conductivity (R1) can be expected to increase with increasing humidity owing to a strong swelling effect. Furthermore, the effect of the short line at low frequencies in complex impedance spectra could be represented by Zw in the equivalent circuit, which characterized the effect of Br− ions and H+ ions. The exponential decrease in R1 and Zw resulted in the dramatic decrease in the impedance of the ionogel sensor with water molecule adsorption, while the ZCPE remained the same, in good accordance with the experimental results. Furthermore, the small change in the Zw value in Fig. 7d further confirmed the absence of fast proton transfer in SiO2/[Bmim]PF6. As a result, it is inferred that the equivalent circuit model can effectively explain the humidity sensing mechanism of the ionogel sensors (Scheme 2).
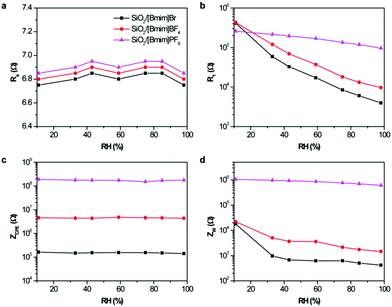 |
| Fig. 7 Dependence of (a) Rs, (b) R1, (c) ZCPE, and (d) ZW values on RH for various ionogels. | |
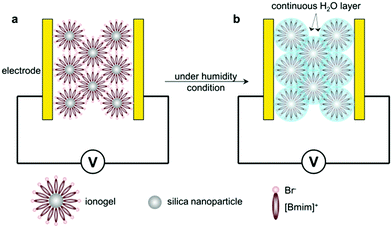 |
| Scheme 2 Illustration of the humidity sensing mechanism of ionogel-based sensors. | |
3.4 Real-time monitoring of human breath
As the SiO2/[Bmim]Br humidity sensor exhibited a fast response and high sensitivity, it is expected to be used in real-time monitoring humidity. Therefore, we explored its usefulness in monitoring human breath using different RH levels during exhalation and inhalation. Using a healthy adult male as volunteer, changes in RH were detected by placing the ionogel humidity sensor in a breathing mask during normal breathing (Scheme 1c). We investigated the response and recovery behaviours at different breathing frequencies for the same duration (Fig. 8a). The RH showed a sharp increase during exhalation and dropped to an ambient value during inhalation, which was consistent with the breathing cycles with different breathing rates. Considering that a normal breathing frequency for a healthy adult is 16–20 breaths per min, we further tested the ionogel sensor at three specific breathing rates, namely slow (10 breaths per min), normal (16 breaths per min), and fast (29 breaths per min). From Fig. 8b, the ionogel sensor efficiently the breathing cycle and was stable at the three breathing frequencies. To confirm that the sensor signal was produced by the response to H2O, two control experiments were conducted. First, as exhaled breath is primarily composed of N2, O2, and CO2, the ionogel humidity sensor was tested in these pure gas atmospheres at 59% RH while keeping the other test conditions constant. The corresponding test results are shown in Fig. S8a (ESI†), clearly showing that N2 had no significant effect on the test results, while O2 and CO2 had a slight effect. However, the effect of interfering gases was far lower than that of humidity. A second control experiment was performed to verify that humidity was the crucial factor when testing exhaled gases. Exhaled gas was dried by passing through a silica gel desiccant and produced no obvious signal when using the ionogel sensor to monitor breathing (Fig. S8b, ESI†). Therefore, the response signal was mainly caused by humidity. Furthermore, the humidity of the test chamber (inhaled gas) and exhaled gas were measured as 58.6% and 91.9% RH, respectively, using a commercial humidity sensor Aosong AM2305 (Aosong (Guangzhou) Electronics Co., Ltd), which was consistent with our test results. These breath-to-breath humidity measurements demonstrated the fast response and sensitivity of the ionogel sensor.
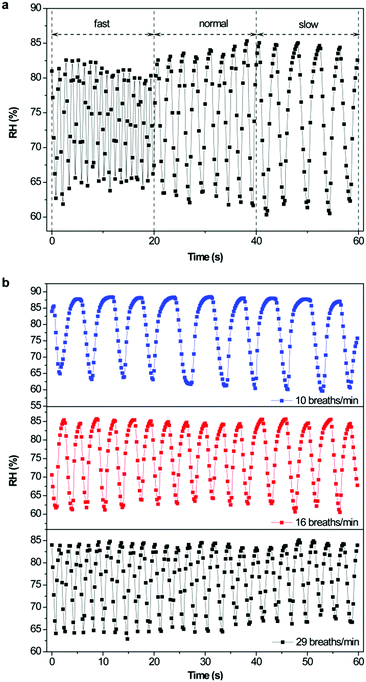 |
| Fig. 8 Response of the SiO2/[Bmim]Br humidity sensor at different breath rates: (a) total, (b) specific breath rate. | |
4. Conclusions
In summary, we have successfully developed a highly sensitive resistive humidity sensor based on an ionogel system. The sensing characteristics for humidity were optimized through anion selection. The ionogel sensors with different types of anion (Br−, BF4−, and PF6−) exhibited good humidity sensitivity, low humidity hysteresis, and good reproducibility. Specifically, by combining high ion conductivity and hydrophilicity, the SiO2/[Bmim]Br-based sensor manifested fast response and recovery behaviours owing to the improved conductivity of Br− and H3O+ under humid conditions. Furthermore, this ionogel sensor with fast response and high sensitivity was effectively employed for the dynamic monitoring of human breath. This study is expected to promote the development of high-performance sensors for real-time monitoring of humidity based on IL hybrid materials.
Conflicts of interest
There are no conflicts to declare.
Acknowledgements
This study was supported by the National Natural Science Foundation of China (grant no. 21601148 and 51574117) and the Natural Science Foundation of Fujian Province (grant no. 2017J05090).
References
-
(a) J. Ascorbe, J. M. Corres, F. J. Arregui and I. R. Matias, Sensors, 2017, 17, 23 Search PubMed;
(b) H. Farahani, R. Wagiran and M. N. Hamidon, Sensors, 2014, 14, 7881 CrossRef PubMed.
-
(a) H. T. Yu, Y. T. Guo, C. Yao, D. F. Perepichka and H. Meng, J. Mater. Chem. C, 2016, 4, 11055 RSC;
(b) T. Fei, H. R. Zhao, K. Jiang and T. Zhang, Sens. Actuators, B, 2015, 208, 277 CrossRef CAS;
(c) Y. Kitamura, R. Ichikawa and H. Nakano, Mater. Chem. Front., 2018, 2, 90 RSC.
-
(a) D. Z. Zhang, H. Y. Chang, P. Li, R. H. Liu and Q. Z. Xue, Sens. Actuators, B, 2016, 225, 233 CrossRef CAS;
(b) A. De Luca, S. Santra, R. Ghosh, S. Z. Ali, J. W. Gardner, P. K. Guha and F. Udrea, Nanoscale, 2016, 8, 4565 RSC;
(c) D. Burman, R. Ghosh, S. Santra and P. K. Guha, RSC Adv., 2016, 6, S7424 RSC;
(d) Z. Yang, Z. Zhang, K. Liu, Q. Yuan and B. Dong, J. Mater. Chem. C, 2015, 3, 6701 RSC;
(e) W. P. Xuan, X. L. He, J. K. Chen, W. B. Wang, X. Z. Wang, Y. Xu, Z. Xu, Y. Q. Fu and J. K. Luo, Nanoscale, 2015, 7, 7430 RSC;
(f) Y. Tan, K. Yu, T. Yang, Q. Zhang, W. Cong, H. Yin, Z. Zhang, Y. Chen and Z. Zhu, J. Mater. Chem. C, 2014, 2, 5422 RSC.
-
(a) J. Zhang, J. Zhong, Y. F. Fang, J. Wang, G. S. Huang, X. G. Cui and Y. F. Mei, Nanoscale, 2014, 6, 13646 RSC;
(b) D. Z. Zhang, J. Tong and B. K. Xia, Sens. Actuators, B, 2014, 197, 66 CrossRef CAS;
(c) X. L. He, D. J. Li, J. Zhou, W. B. Wang, W. P. Xuan, S. R. Dong, H. Jin and J. K. Luo, J. Mater. Chem. C, 2013, 1, 6210 RSC.
-
(a) M. A. Mahjoub, G. Monier, C. Robert-Goumet, F. Réveret, M. Echabaane, D. Chaudanson, M. Petit, L. Bideux and B. Gruzza, J. Phys. Chem. C, 2016, 120, 11652 CrossRef CAS;
(b) R. Xuan, Q. Wu, Y. Yin and J. Ge, J. Mater. Chem., 2011, 21, 3672 RSC;
(c) P.-G. Su and S.-C. Huang, Sens. Actuators, B, 2006, 113, 142 CrossRef CAS.
- F. U. Hernandez, S. P. Morgan, B. R. Hayes-Gill, D. Harvey, W. Kinnear, A. Norris, D. Evans, J. G. Hardman and S. Korposh, IEEE Trans. Biomed. Eng., 2016, 63, 1985 Search PubMed.
- U. Mogera, A. A. Sagade, S. J. George and G. U. Kulkarni, Sci. Rep., 2014, 4, 4103 CrossRef PubMed.
-
(a) Y. Wang, S. Gong, S. J. Wang, G. P. Simon and W. L. Cheng, Mater. Horizons, 2016, 3, 208 RSC;
(b) K. Behera, S. Pandey, A. Kadyan and S. Pandey, Sensors, 2015, 15, 30487 CrossRef CAS PubMed;
(c) C. F. Wang, Y. J. Chen, K. L. Zhuo and J. J. Wang, Chem. Commun., 2013, 49, 3336 RSC;
(d) A. Rehman and X. Q. Zeng, Acc. Chem. Res., 2012, 45, 1667 CrossRef CAS;
(e) G. K. Dedzo, S. Letaief and C. Detellier, J. Mater. Chem., 2012, 22, 20593 RSC;
(f) L. Yu, Y. Huang, X. X. Jin, A. J. Mason and X. Q. Zeng, Sens. Actuators, B, 2009, 140, 363 CrossRef CAS;
(g) L. Yu, D. Garcia, R. B. Rex and X. Q. Zeng, Chem. Commun., 2005, 2277 RSC.
-
(a) J. Lee and D. S. Silvester, Analyst, 2016, 141, 3705 RSC;
(b) J. Lee, G. D. Plessis, D. W. M. Arrigan and D. S. Silvester, Anal. Methods, 2015, 7, 7327 RSC;
(c) D. S. Sliveter, Analyst, 2011, 136, 4871 RSC.
-
(a) W. J. Qian, J. Texter and F. Yan, Chem. Soc. Rev., 2017, 46, 1124 RSC;
(b) L. L. Wang, X. C. Duan, W. Y. Xie, Q. H. Li and T. H. Wang, Chem. Commun., 2016, 52, 8417 RSC;
(c) R. Guterman, M. Ambrogi and J. Y. Yuan, Macromol. Rapid Commun., 2016, 37, 1106 CrossRef CAS PubMed;
(d) X. R. Zhu, H. J. Zhang and J. M. Wu, Sens. Actuators, B, 2014, 202, 105 CrossRef CAS;
(e) J. Huang, C. A. Tao, Q. An, C. X. Lin, X. S. Li, D. Xu, Y. G. Wu, X. G. Li, D. Z. Shen and G. T. Li, Chem. Commun., 2010, 46, 4103 RSC.
-
(a) I. F. Diaz-Ortega, J. Ballesta-Claver, M. C. Martin, S. Benitez-Aranda and L. F. Capitan-Vallvey, RSC Adv., 2014, 4, 57235 RSC;
(b) J. Le Bideau, L. Viau and A. Vioux, Chem. Soc. Rev., 2011, 40, 907 RSC;
(c) M. A. Neouze, J. Le Bideau, P. Gaveau, S. Bellayer and A. Vioux, Chem. Mater., 2006, 18, 3931 CrossRef CAS.
- P. Sun and D. W. Armstrong, Anal. Chim. Acta, 2010, 661, 1 CrossRef CAS PubMed.
-
(a) R. C. Evans and P. C. Marr, Chem. Commun., 2012, 48, 3742 RSC;
(b) L. S. Fu, R. A. S. Ferreira, N. J. O. Silva, L. D. Carlos, V. D. Bermudez and J. Rocha, Chem. Mater., 2004, 16, 1507 CrossRef CAS.
-
(a) F. F. Yang, D. Wu, Z. Y. Luo, B. Tan and Z. L. Xie, Sens. Actuators, B, 2017, 249, 486 CrossRef CAS;
(b) X. C. Duan, H. Huang, S. H. Xiao, J. W. Deng, G. Zhou, Q. H. Li and T. H. Wang, J. Mater. Chem. A, 2016, 4, 8402 RSC;
(c) C. M. Wu, S. Y. Lin, K. Y. Kao and H. L. Chen, J. Phys. Chem. C, 2014, 118, 17764 CrossRef CAS;
(d) X. C. Duan, T. Kim, D. Li, J. M. Ma and W. J. Zheng, Chem. – Eur. J., 2013, 19, 5924 CrossRef CAS PubMed.
- Z. Ma, J. H. Yu and S. Dai, Adv. Mater., 2010, 22, 261 CrossRef CAS PubMed.
- W. C. Geng, Q. Yuan, X. M. Jiang, J. C. Tu, L. B. Duan, J. W. Gu and Q. Y. Zhang, Sens. Actuators, B, 2012, 174, 513 CrossRef CAS.
- K. Jiang, H. Zhao, T. Fei, H. Dou and T. Zhang, Sens. Actuators, B, 2016, 222, 440 CrossRef CAS.
- Y. Chen, Y. P. Zhang, D. M. Li, F. L. Gao, C. H. Feng, S. P. Wen and S. P. Ruan, Sens. Actuators, B, 2015, 212, 242 CrossRef CAS.
Footnote |
† Electronic supplementary information (ESI) available: SEM images and FT-IR spectra of ionogels, the impedance vs. RH of ionogel sensors measured at various frequencies, long-term stability, etc. See DOI: 10.1039/c8qm00596f |
|
This journal is © the Partner Organisations 2019 |
Click here to see how this site uses Cookies. View our privacy policy here.