DOI:
10.1039/C8RA08678H
(Paper)
RSC Adv., 2019,
9, 1967-1975
Effect of lignocellulose-derived weak acids on butanol production by Clostridium acetobutylicum under different pH adjustment conditions
Received
19th October 2018
, Accepted 13th December 2018
First published on 15th January 2019
Abstract
The effects of formic acid, acetic acid and levulinic acid on acetone–butanol–ethanol (ABE) fermentation under different pH adjustment conditions were investigated using Clostridium acetobutylicum as the fermentation strain. CaCO3 supplementation can alleviate the inhibitory effect of formic acid on ABE production. The ABE titers from the medium containing 0.5 g L−1 formic acid with pH adjusted by CaCO3 and KOH were 11.08 g L−1 and 1.04 g L−1, which reached 64.8% and 6.3% of the control group, respectively. Compared with CaCO3 pH adjustment, fermentation results with higher ABE titers and yields were obtained from the medium containing acetic acid or levulinic acid, when the pH was adjusted by KOH. When formic acid, acetic acid, and levulinic acid co-existed in the medium, better fermentation result was achieved by adjusting the pH by CaCO3. Moreover, 12.50 g L−1 ABE was obtained from the medium containing 2.0 g L−1 acetic acid, 0.4 g L−1 formic acid, and 1.0 g L−1 levulinic acid as compared to 3.98 g L−1 ABE obtained from the same medium when the pH was adjusted by KOH. CaCO3 supplementation is a more favorable pH adjustment method for ABE medium preparation from lignocellulosic hydrolysate.
1. Introduction
Recent concerns about the fossil fuel depletion and environmental deterioration have aroused significant interests in the field of renewable fuel production.1 Butanol, a promising renewable fuel, is recognized as a potential substitute of gasoline due to its favorable fuel properties such as high energy density, low volatility and hygroscopicity, less corrosiveness as well as the high miscibility with gasoline at any ratio.2 Butanol can be produced by acetone–butanol–ethanol (ABE) fermentation from lignocellulosic biomass by Clostridium strains (such as C. acetobutylicum).3–7 The pentoses and hexoses in the lignocellulosic hydrolysate can be utilized by C. acetobutylicum for butanol production.8,9 In addition, the cost of lignocellulosic biomass is about 3 to 5 times more economical than that of traditional starchy substrates such as corn.10,11 Therefore, lignocellulosic biomass is expected to be an ideal substrate for butanol production.12
Although lignocellulose is the most abundant feedstock for butanol production, it is not easy to obtain fermentable sugars from the native lignocellulosic biomass.13 Diluted acid pretreatment has emerged as a favorable method for industrial scale polysaccharide degradation.14 The polysaccharides can be degraded to fermentable sugars (such as glucose, xylose, arabinose, etc.) by the dilute acid pretreatment. Butanol fermentation from dilute acid hydrolysate of lignocellulose was expected to be an ideal mode for its large-scale production. However, inhibitors in the hydrolysate impede the butanol refining efficiency. The inhibitors mainly include furan derivatives, phenolics, and weak acids. The furan derivatives are furan and 5-hydroxymethylfurfural (5-HMF), which are derived from pentoses and hexoses, respectively. Phenolic compounds (such as coumaric acid, ferulic acid, and syringaldehyde) originate from Klasson lignin.15 Weak acids mainly include acetic acid, formic acid, and levulinic acid.16 Acetic acid is liberated from the acetyl groups of hemicellulose fraction.17 Formic acid is obtained from the degradation of furfural and 5-HMF, and levulinic acid is obtained from the degradation of 5-HMF.18 Thus, it is urgent to develop a simple and efficient process control method.
The inhibiting or promoting effects of acetic acid and/or formic acid on ABE fermentation have been reported.19–23 The undissociated weak acids can diffuse across the cell membrane, which will result in the drop of intracellular pH and the collapse of the transmembrane proton gradient.24,25 Most researchers have focused on the removal of weak acids from hydrolysate by chemical treatment or physical adsorption.26,27 There are few reports on the process control strategies for improving the ABE fermentation efficiency without weak acids removal. Our previous study has suggested that the inhibiting effect of weak acids (formic acid) on ABE fermentation can be alleviated by improving the buffering capacity of the medium.28 Based on the weak electrolyte dissociation characteristics of weak acids, the medium buffering capacity was increased to improve the butanol fermentation efficiency from lignocellulose hydrolysate without the removal of the inhibitors. This study aimed at the investigation of the effects of weak acid inhibitors produced by the degradation of lignocellulose on the ABE fermentation under different pH adjustment conditions.
2. Materials and methods
2.1. Strains and seed culture
C. acetobutylicum CH02, obtained from C. acetobutylicum ATCC 824 by long-term adaptation, was used for butanol production in this research. The inoculum of C. acetobutylicum CH02 was prepared as described in our previous study.29
2.2. Medium preparation and batch fermentation
The medium of the control group was composed of (per liter medium): glucose 25 g, xylose 25 g and wheat bran 11 g. To evaluate the influence of formic acid, acetic acid, and levulinic acid on ABE fermentation, each single acid at different concentrations (formic acid: 0.1, 0.2, 0.3, 0.4, and 0.5 g L−1; acetic acid: 0.2, 0.5, 1.0, 2.0, and 5.0 g L−1; levulinic acid: 0.2, 0.5, 1.0, 2.0, and 5.0 g L−1) and combined acids (formic acid 0.4 g L−1, acetic acid 2.0 g L−1 and levulinic acid 1.0 g L−1) were added to the media. The initial pH of the media after the addition of the acids was adjusted to 6.5 via the following two methods: (i) by adding 4 g L−1 CaCO3 and a small amount of CaO or (ii) by adding 6 M KOH. The initial pH of the media without the addition of the acids was adjusted to 6.5 with 20 mM sulfuric acid.
All batch fermentations were conducted in 250 mL serum bottles with the working volume of 200 mL in duplicates. The inoculation levels were controlled at 5% (v/v), and the bottles were incubated statically at 37 °C for 120 h.
2.3. Analytical methods
The solvents (acetone, butanol and ethanol) were analyzed using a gas chromatograph (GC, Agilent 7890A). The acids (formate, acetate, butyrate, and levulinate) and sugars (glucose and xylose) were measured by a high performance liquid chromatograph (HPLC, Waters 2695e) as in our previous report.28 The pH of the medium was maintained by a pH meter. The ABE yield was calculated as given in the following eqn (1): |
 | (1) |
where cacetone, cbutanol and cethanol means the concentration of acetone, butanol, and ethanol in the fermentation broth, respectively. ciglucose and cixylose means the initial concentration of glucose and xylose in the medium before fermentation, respectively. crglucose and crxylose means the residual concentration of glucose and xylose, respectively.
3. Results and discussion
3.1. Effect of acetic acid on ABE fermentation under different pH adjustment conditions
To investigate the effect of acetic acid on butanol fermentation under different pH adjustment conditions, butanol fermentation was conducted in groups A1–A5 (containing 0.2, 0.5, 1.0, 2.0, and 5.0 g L−1 acetic acid). In the control group B, butanol fermentation was conducted without the addition of acetic acid. Fig. 1 and 2 show the fermentation results with pH adjusted by CaCO3 and KOH, respectively.
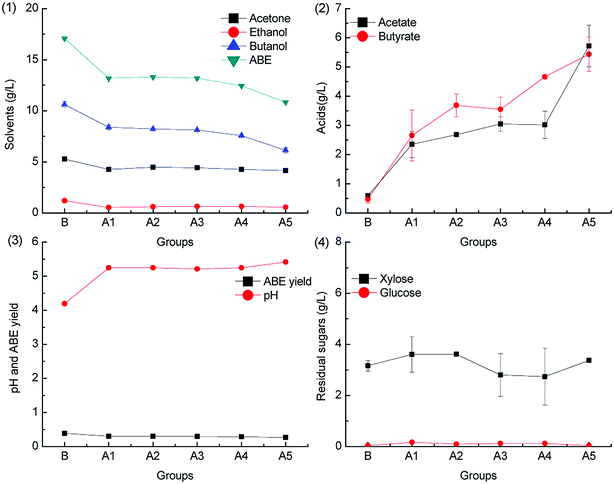 |
| Fig. 1 Effect of acetic acid on ABE fermentation with pH adjusted by CaCO3. | |
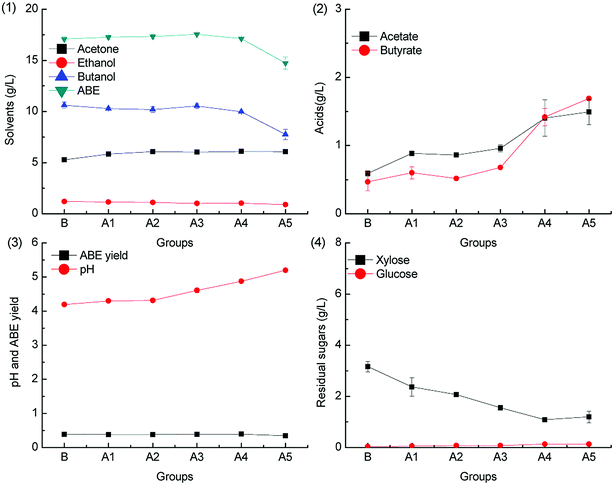 |
| Fig. 2 Effect of acetic acid on ABE fermentation with pH adjusted by KOH. | |
As can be seen from Fig. 1.1, with CaCO3 supplementation, the solvent (acetone, ethanol, and butanol) titers were lower than those in the control group. However, the acid accumulation is relatively high. ABE fermentation by C. acetobutylicum is typically characterized by acidogenesis and solventogenesis.30 The acetic acid concentrations after fermentation in group A1–A5 were higher than the native supplementation levels; this suggested that no net acetic acid was consumed. In the batch fermentation A5, 5.72 g L−1 acetic acid and 5.42 g L−1 butyric acid were accumulated after fermentation. On the one hand, the relatively high pH level, as shown in Fig. 1.3, caused by CaCO3 supplementation can induce acid accumulation in acidogenesis.31 On the other hand, the dissociated acetic acid and butyric acid cannot be re-assimilated in solventogenesis.32 Therefore, the acid concentration after fermentation in A1–A5 was high. Interestingly, the accumulation trends of acetic acid and butyric acid in different batch fermentations were similar (as shown in Fig. 1.2). This was because ATP production from acetic acid pathway was inhibited by the high acetic acid supplementation level in the medium, and therefore, most of the ATP was produced through the butyric acid pathway.28 The acetic acid and butyric acid accumulation consumed a large amount of the substrates, and the solvent yield was relatively lower than that in the control group, as shown in Fig. 3.3. The residual sugars after all batch fermentations were lower than 4 g L−1 (Fig. 1.4); this suggested that the acetic acid supplementation lower than 5 g L−1 did not cause significant inhibition of sugar consumption.
When the pH was adjusted by KOH, the fermentation results were obviously different. As illustrated in Fig. 2.1, the ABE titers increased slightly when the acetic acid supplementation was increased from 0.2 to 1.0 g L−1, and the ABE titers reached 17.54 g L−1 when 1.0 g L−1 acetic acid was added to the medium. However, the ABE titers decreased when acetic acid supplementation further increased to 5.0 g L−1. Compared with Fig. 1.2, the re-assimilation of acetic acid was obvious. As shown in Fig. 2.2, 17.88%, 31.20% and 69.97% supplemented acetic acid was consumed in the batch fermentations A3-A5. More undissociated acetic acid and butyric acid were re-assimilated by C. acetobutylicum when the medium pH was at a relatively low level, as shown in Fig. 2.3. Acetic acid, glucose, and xylose can serve as the co-substrates for solvent production. Solvent yield calculated from the sugar in Fig. 2.3 was higher than that in Fig. 1.3. As illustrated in Fig. 2.4, glucose was almost completely consumed after fermentation, and the residual xylose was lower than 4 g L−1. It can be seen from the comparison results that when the medium is adjusted by KOH, acetic acid can be re-assimilated at a relatively high level, which will result in a high solvent yield.
3.2. Effect of formic acid on ABE fermentation under different pH adjustment conditions
Formic acid is deleterious to ABE fermentation by C. acetobutylicum because it can trigger acid crash,20 but the inhibitory effect can be alleviated by CaCO3 supplementation.28 The inhibitory effect of formic acid on ABE fermentation with different concentration was evaluated herein, and the results are shown in Fig. 3 and 4.
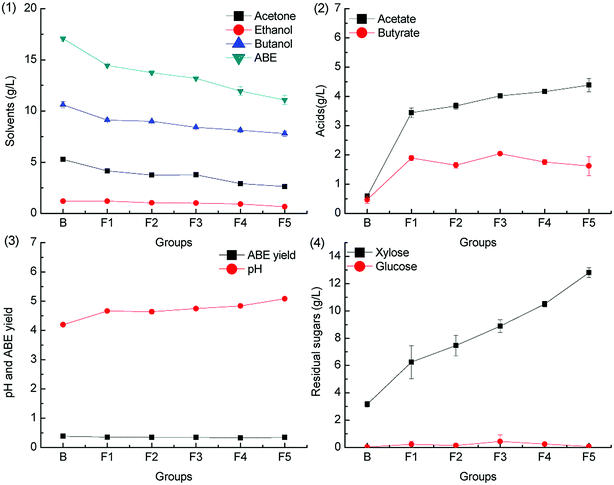 |
| Fig. 3 Effect of formic acid on ABE fermentation with pH adjusted by CaCO3. | |
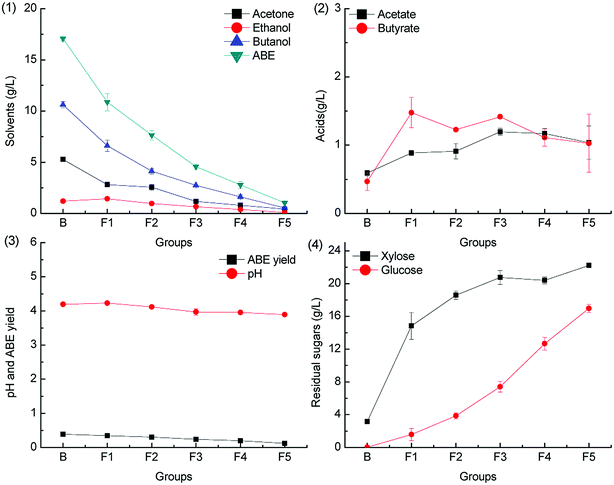 |
| Fig. 4 Effect of formic acid on ABE fermentation with pH adjusted by KOH. | |
To investigate the effect of formic acid on butanol fermentation under different pH adjustment conditions, batch fermentations were conducted in the groups F1–F5 (containing 0.1, 0.2, 0.3, 0.4, and 0.5 g L−1 formic acid). The ABE titers obtained from the medium F1–F5 were 84.5%, 80.5%, 77.2%, 70.0% and 64.8% of the control group B when the pH was adjusted by CaCO3. However, the ABE titers in the batch fermentation of F1–F5 reached only 63.6%, 44.7%, 26.7%, 16.4% and 6.1% of the control group when the pH was adjusted by KOH. The results are in agreement with our previous report that CaCO3 can alleviate the inhibitory effect of formic acid on butanol fermentation.28 The acids production with different pH adjustment methods is obviously different. As shown in Fig. 3.2, acetic acid concentration accumulated in the medium was higher than that of butyric acid in batch fermentation of groups F1–F5, whereas the acetic acid and butyric acid accumulation in the control group was almost the same. In contrast, the differences in acids accumulation in groups F1–F5 in Fig. 4.2 were not obvious. Acids production was accompanied by ATP generation, and ATP generation efficiency from acetic acid production was higher than that from butyric acid production.33 The uncoupling effect caused by the undissociated acids can be alleviated by the over-production of acetate. The ABE yield was between 0.3 g g−1 and 0.4 g g−1, as shown in Fig. 3.3, which was higher than that shown in Fig. 4.3 from the same medium.
3.3. Effect of levulinic acid on ABE fermentation under different pH adjustment conditions
Levulinic acid is produced from hexoses through the intermediate 5-HMF under acid pretreatment condition.34 Prior to butanol production from the hemicellulose hydrolysate, levulinic acid is often removed as the fermentation inhibitor.35,36 In this study, we have found that levulinic acid can be metabolized by C. acetobutylicum. To the best of our knowledge, there is no report on the catabolism pathway of levulinic acid in C. acetobutylicum. The metabolism pathway of levulinic acid in other microorganisms such as Cupriavidus necator has been reported, and it is found that levulinic acid is metabolized via conversion to acetyl-CoA and propionyl-CoA by an inducible levulinic acid acyl-CoA synthetase.37 The ABE fermentation results with different levulinic acid supplementation levels in groups L1–L5 (0.2 g L−1, 0.5 g L−1, 1.0 g L−1, 2.0 g L−1 and 5.0 g L−1) are illustrated in Fig. 5 and 6.
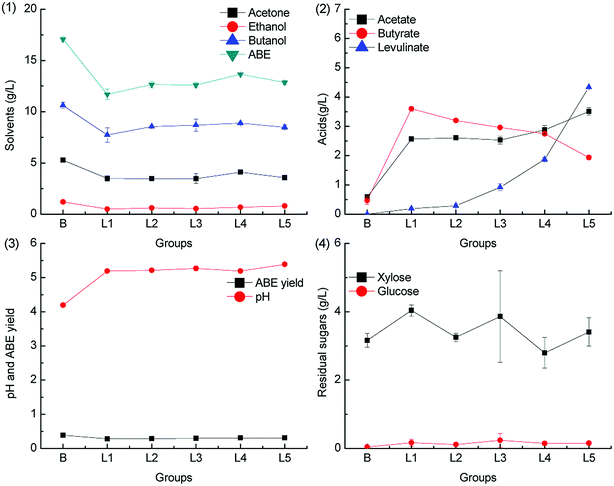 |
| Fig. 5 Effect of levulinic acid on ABE fermentation with pH adjusted by CaCO3. | |
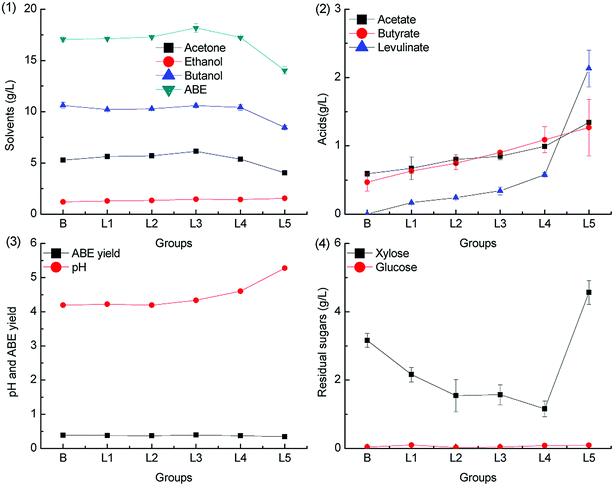 |
| Fig. 6 Effect of formic acid on ABE fermentation with pH adjusted by KOH. | |
As illustrated in Fig. 5, the ABE titers were lower than those in the control group when the pH was adjusted by CaCO3. On the other hand, more acetic acid and butyric acid were accumulated, and only a small quantity of levulinic acid was consumed at the end of the fermentation. When CaCO3 was supplemented in the medium, the pH was maintained at a relatively high level due to the pH buffering capacity of CaCO3, as shown in Fig. 5.3. Therefore, more acetic acid and butyric acid were produced and accumulated in the dissociated state, which could not be re-assimilated. This also resulted in low ABE yield. No obvious inhibitory effects on the sugar consumption were observed when 5.0 g L−1 levulinic acid was added to the fermentation medium.
When the fermentation pH was adjusted by KOH, the ABE titers increased slightly as compared to the case of the control group, with the levulinic acid supplementation level ranging from 0.2 g L−1 to 2.0 g L−1; the results are shown in Fig. 6. The maximum concentration of 18.17 g L−1 ABE was produced when 1.0 g L−1 levulinic acid was added to the medium, which was 6.5% higher as compared to that achieved from the control group. When the levulinic acid supplementation level was higher than 2.0 g L−1, the ABE titers decreased significantly, and the phenomenon could be explained from the acid production. The acetic acid and butyric acid accumulation increased with the levulinic acid supplementation level; this was due to the pH buffering capacity of potassium levulinate. As illustrated in Fig. 6.3, the final pH level increased with the levulinic acid supplementation level. The accumulated acetic acid and butyric acid were in the dissociated state and could not be re-assimilated for ABE production; therefore, the ABE titers and yield decreased as compared to that in the control group.
3.4. Effect of the co-presence of acetic acid, formic acid, and levulinic acid on ABE fermentation under different pH adjustment conditions
To investigate the co-effect of acetic acid, formic acid, and levulinic acid on ABE fermentation under different pH adjustment conditions, the acids concentrations were selected according to the wheat straw hydrolysate composition of our previous study with some changes.38 Batch fermentations with the co-presence of 2.0 g L−1 acetic acid, 0.4 g L−1 formic acid, and 1.0 g L−1 levulinic acid were performed.
Herein, 0.09 g L−1 acetic acid, 0.03 g L−1 formic acid, and 0.08 g L−1 levulinic acid were detected in the control medium because of the auto-hydrolysis of cellulose and hemicellulose component in wheat bran during the autoclave process. The fermentation results with the co-presence of three kinds of acids are illustrated in Fig. 7.
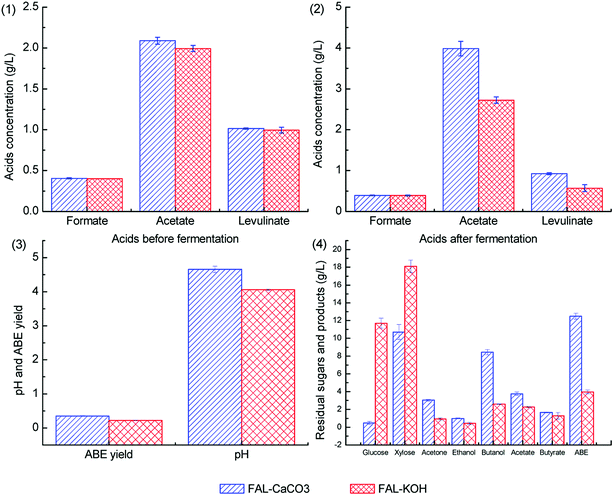 |
| Fig. 7 Effect of co-presence acetic acid, formic acid and levulinic acid on ABE fermentation under different pH adjustment conditions. FAL-KOH, co-presence of formic acid, acetic acid and levulinic acid with pH adjusted by KOH. FAL-CaCO3, co-presence of formic acid, acetic acid and levulinic acid with pH adjusted by CaCO3. | |
As shown in Fig. 7.1 and 7.2, formic acid cannot be metabolized under different pH adjustment conditions due to the lack of formate dehydrogenase (FDH) in C. acetobutylicum19. The levulinic acid consumption ratios in batch fermentations with pH adjusted by CaCO3 and KOH were 8.9% and 42.7%, respectively. More levulinic acid was consumed when the fermentation pH was adjusted by KOH, this was because more levulinic acid was present in the dissociated state when CaCO3 was supplemented to improve the pH buffering capacity. For the ABE production shown in Fig. 7.3, ABE titers in batch fermentations with pH adjusted by CaCO3 and KOH were 12.50 g L−1 and 3.98 g L−1, which reached 73.2% and 23.3% of the control group, respectively. As shown in Fig. 7.4, the ABE yield in batch fermentations with pH adjusted by CaCO3 and KOH was 0.35 g g−1 and 0.22 g g−1, respectively. Among the three kinds of weak acids, formic acid exhibits fatal inhibitory effect on the ABE production. Compared with the batch fermentation results of group F4 in Fig. 4.1, the inhibitory effect of formic acid can be alleviated by acetic acid and levulinic acid supplementation. On the one hand, acetate and levulinate can increase the medium's pH buffering capacity. On the other hand, acetic acid can play a role in preventing degeneration and enhancing the CoA transferase activity.21,39 Acetic acid and butyric acid accumulation was also different because of the different pH buffering capacity of KOH and CaCO3, and more acetic acid and butyric acid were accumulated when CaCO3 was supplemented. Cho et al. reported that the inhibition of formic acid on C. acetobutylicum can be alleviated by acetic acid supplementation, and the results in this study are in agreement with these conclusions.19 Weak acids such as formic acid, acetic acid, and levulinic acid often co-exist in the biomass hydrolysate.40 Previous studies have often been focused on the removal of fermentation inhibitors (weak acids included). Poor fermentation results with low ABE titers and yields were achieved when the medium pH was adjusted by KOH or other alkali without pH buffering capacity. Qureshi et al. reported that only 1.48 g L−1 of ABE was obtained from the switchgrass hydrolysate when the pH was adjusted by NaOH.41
4. Conclusions
Among the three kinds of weak acids, formic acid exhibits fatal inhibitory effect on the ABE production. The inhibitory effect of formic acid can be alleviated by CaCO3 supplementation. However, pH adjustment with KOH was more suitable when a single acid such as acetic acid or levulinic acid existed in the medium. Satisfactory fermentation results were achieved with pH adjusted by CaCO3 in the medium containing the three kind of weak acids. Formic acid, acetic acid, and levulinic acid often co-exist in the lignocellulosic hydrolysate. pH controlling strategy by CaCO3 is compatible with industrial applications because of its economic and technical feasibility. On the one hand, CaCO3 is more affordable than KOH or other common alkaline materials. On the other hand, pH adjustment by CaCO3 is technically feasible because their supplementation level controls is easy, and even an excessive dose of CaCO3 supplementation will not result in a pH increase. Therefore, CaCO3 supplementation is the more favorable pH adjustment method for ABE medium preparation from lignocellulosic hydrolysate.
Conflicts of interest
There are no conflicts of interest to declare.
Acknowledgements
This work was supported by the National Key Research & Development Program of China (2016YFE0205600), National Key Project of China (2015ZX07103-007), Science and Technology Innovation Foundation of Chongqing (cstc2018jcyjAX0638 and cstc2018jscx-msybX0337), and Open Funding of Chongqing Technology and Business University (KFJJ2017072). We thank Dr M. Asraful Alam at GIEC-CAS for helpful discussions and language editing.
References
- A. I. Paniagua-García, M. Hijosa-Valsero, R. Díez-Antolínez, M. E. Sánchez and M. Coca, Enzymatic hydrolysis and detoxification of lignocellulosic biomass are not always necessary for ABE fermentation: the case of Panicum virgatum, Biomass Bioenergy, 2018, 116, 131–139 CrossRef.
- X. Lin, L. Xiong, G. Qi, S. Shi, C. Huang, X. Chen and X. Chen, Using Butanol Fermentation Wastewater for Biobutanol Production after Removal of Inhibitory Compounds by Micro/Mesoporous Hyper-Cross-Linked Polymeric Adsorbent, ACS Sustainable Chem. Eng., 2015, 3, 702–709 CrossRef CAS.
- Y. Wang, W. Guo, C. L. Cheng, S. H. Ho, J. S. Chang and N. Ren, Enhancing bio-butanol production from biomass of Chlorella vulgaris JSC-6 with sequential alkali pretreatment and acid hydrolysis, Bioresour. Technol., 2016, 200, 557–564 CrossRef CAS PubMed.
- L. V. Reddy, A. S. Veda and Y. J. Wee, Utilization of Sugarcane Field Residue (SFR) as Renewable Feedstock for Biobutanol Production, Sugar Tech, 2018, 20, 168–174 CrossRef CAS.
- A. Procentese, F. Raganati, G. Olivieri, M. E. Russo, M. de la Feld and A. Marzocchella, Renewable feedstocks for biobutanol production by fermentation, New Biotechnol., 2017, 39, 135–140 CrossRef CAS PubMed.
- L. D. Gottumukkala, K. Haigh and J. Görgens, Trends and advances in conversion of lignocellulosic biomass to biobutanol: microbes, bioprocesses and industrial viability, Renewable Sustainable Energy Rev., 2017, 76, 963–973 CrossRef CAS.
- A. Procentese, F. Raganati, G. Olivieri, M. E. Russo and A. Marzocchella, Pre-treatment and enzymatic hydrolysis of lettuce residues as feedstock for bio-butanol production, Biomass Bioenergy, 2017, 96, 172–179 CrossRef CAS.
- F. Raganati, G. Olivieri, P. Götz, A. Marzocchella and P. Salatino, Butanol production from hexoses and pentoses by fermentation of Clostridium acetobutylicum, Anaerobe, 2015, 34, 146–155 CrossRef CAS PubMed.
- F. Raganatia, A. Procentesea, G. Olivieria, P. Salatinoa and A. Marzocchellaa, Biobutanol production from hexose and pentose sugars, Chem. Eng. Trans., 2014, 38, 193–198 Search PubMed.
- N. Qureshi, M. J. Bowman, B. C. Saha, R. Hector, M. A. Berhow and M. A. Cotta, Effect of cellulosic sugar degradation products (furfural and hydroxymethyl furfural) on acetone–butanol–ethanol (ABE) fermentation using Clostridium beijerinckii P260, Food Bioprod. Process., 2012, 90, 533–540 CrossRef CAS.
- V. H. Díaz and G. O. Tost, Butanol production from lignocellulose by simultaneous fermentation, saccharification, and pervaporation or vacuum evaporation, Bioresour. Technol., 2016, 218, 174–182 CrossRef PubMed.
- Y. N. Zheng, L. Z. Li, M. Xian, Y. J. Ma, J. M. Yang, X. Xu and D. Z. He, Problems with the microbial production of butanol, J. Ind. Microbiol. Biotechnol., 2009, 36, 1127–1138 CrossRef CAS PubMed.
- Y. S. Jang, A. Malaviya, C. Cho, J. Lee and S. Y. Lee, Butanol production from renewable biomass by clostridia, Bioresour. Technol., 2012, 123, 653–663 CrossRef CAS PubMed.
- P. Alvira, E. Tomáspejó, M. Ballesteros and M. J. Negro, Pretreatment technologies for an efficient bioethanol production process based on enzymatic hydrolysis: A review, Bioresour. Technol., 2010, 101, 4851–4861 CrossRef CAS PubMed.
- E. Ximenes, Y. Kim, N. Mosier, B. Dien and M. Ladisch, Inhibition of cellulases by phenols, Enzyme Microb. Technol., 2010, 46, 170–176 CrossRef CAS.
- L. J. Jönsson, B. Alriksson and N. O. Nilvebrant, Bioconversion of lignocellulose: inhibitors and detoxification, Biotechnol. Biofuels, 2013, 6, 16 CrossRef PubMed.
- C. Cara, E. Ruiz, J. M. Oliva, F. Sáez and E. Castro, Conversion of olive tree biomass into fermentable sugars by dilute acid pretreatment and enzymatic saccharification, Bioresour. Technol., 2008, 99, 1869–1876 CrossRef CAS PubMed.
- S. Larsson, E. Palmqvist, B. Hahn-Hägerdal, C. Tengborg, K. Stenberg, G. Zacchi and N. O. Nilvebrant, The generation of fermentation inhibitors during dilute acid hydrolysis of softwood, Enzyme Microb. Technol., 1999, 24, 151–159 CrossRef CAS.
- D. H. Cho, S. J. Shin and H. K. Yong, Effects of acetic and formic acid on ABE production by Clostridium acetobutylicum and Clostridium beijerinckii, Biotechnol. Bioprocess Eng., 2012, 17, 270–275 CrossRef CAS.
- S. Wang, Y. Zhang, H. Dong, S. Mao, Y. Zhu, R. Wang, G. Luan and Y. Li, Formic Acid Triggers the “Acid Crash” of Acetone-Butanol-Ethanol Fermentation by Clostridium acetobutylicum, Appl. Environ. Microbiol., 2011, 77, 1674–1680 CrossRef CAS PubMed.
- C. K. Chen and H. P. Blaschek, Acetate enhances solvent production and prevents degeneration in Clostridium beijerinckii BA101, Appl. Microbiol. Biotechnol., 1999, 52, 170–173 CrossRef CAS PubMed.
- C. K. Chen and H. P. Blaschek, Effect of acetate on molecular and physiological aspects of Clostridium beijerinckii NCIMB 8052 solvent production and strain degeneration, Appl. Environ. Microbiol., 1999, 65, 499–505 CAS.
- A. Procentese, F. Raganati, G. Olivieri, M. E. Russo, P. Salatino and A. Marzocchella, Continuous xylose fermentation by Clostridium acetobutylicum–kinetics and energetics issues under acidogenesis conditions, Bioresour. Technol., 2014, 164, 155–161 CrossRef CAS PubMed.
- J. B. Russell, Another explanation for the toxicity of fermentation acids at low pH: anion accumulation versus uncoupling, J. Appl. Bacteriol., 2010, 73, 363–370 Search PubMed.
- D. D. Axe and J. E. Bailey, Transport of lactate and acetate through the energized cytoplasmic membrane of Escherichia coli, Biotechnol. Bioeng., 2010, 47, 8–19 CrossRef PubMed.
- Q. Huang, H. Zhang, L. Xiong, C. Huang, H. Guo, X. Chen, M. Luo, L. Tian, X. Lin and X. Chen, Controllable Synthesis of Styrene-divinylbenzene Adsorption Resins and the Effect of Textural Properties on Removal Performance of Fermentation Inhibitors from Rice Straw Hydrolysate, Ind. Eng. Chem. Res., 2018, 57, 5119–5127 CrossRef CAS.
- S. I. Mussatto and I. C. Roberto, Alternatives for detoxification of diluted-acid lignocellulosic hydrolyzates for use in fermentative processes: a review, Bioresour. Technol., 2004, 93, 1–10 CrossRef CAS PubMed.
- G. Qi, L. Xiong, X. Lin, C. Huang, H. Li, X. Chen and X. Chen, CaCO3 supplementation alleviates the inhibition of formic acid on acetone/butanol/ethanol fermentation by Clostridium acetobutylicum, Biotechnol. Lett., 2017, 39, 1–8 CrossRef PubMed.
- G. X. Qi, L. Xiong, C. Huang, X. F. Chen, X. Q. Lin and X. D. Chen, Solvents Production from a Mixture of Glucose and Xylose by Mixed Fermentation of Clostridium acetobutylicum and Saccharomyces cerevisiae, Appl. Biochem. Biotechnol., 2015, 177, 996–1002 CrossRef CAS PubMed.
- C. Xue, X. Q. Zhao, C. G. Liu, L. J. Chen and F. W. Bai, Prospective and development of butanol as an advanced biofuel, Biotechnol. Adv., 2013, 31, 1575–1584 CrossRef CAS PubMed.
- I. S. Maddox, E. Steiner, S. Hirsch, S. Wessner, N. A. Gutierrez, J. R. Gapes and K. C. Schuster, The cause of “acid-crash” and “acidogenic fermentations” during the batch acetone-butanol-ethanol (ABE-) fermentation process, J. Mol. Microbiol. Biotechnol., 2000, 2, 95 CAS.
- Y. Wang, X. Li and H. P. Blaschek, Effects of supplementary butyrate on butanol production and the metabolic switch in Clostridium beijerinckiiNCIMB 8052: genome-wide transcriptional analysis with RNA-Seq, Biotechnol. Biofuels, 2013, 6, 138 CrossRef CAS PubMed.
- R. P. Desai and N. L. P. Et, Stoichiometric modeling of Clostridium acetobutylicum fermentations with non-linear constraints, J. Biotechnol., 1999, 71, 191 CrossRef CAS.
- C. Chang, P. Cen and X. Ma, Levulinic acid production from wheat straw, Bioresour. Technol., 2007, 98, 1448–1453 CrossRef CAS PubMed.
- W. L. Zhang, Z. Y. Liu, Z. Liu and F. L. Li, Butanol production from corncob residue using Clostridium beijerinckii NCIMB 8052, Lett. Appl. Microbiol., 2012, 55, 240–246 CrossRef CAS PubMed.
- C. Lu, J. Dong and S. T. Yang, Butanol production from wood pulping hydrolysate in an integrated fermentation-gas stripping process, Bioresour. Technol., 2013, 143, 467–475 CrossRef CAS PubMed.
- M. Jaremko and J. Yu, The initial metabolic conversion of levulinic acid in Cupriavidus necator, J. Biotechnol., 2011, 155, 293 CrossRef CAS PubMed.
- G. Qi, L. Xiong, B. Wang, X. Lin, H. Zhang, H. Li, C. Huang, X. Chen, C. Wang and X. Chen, Improvement and Characterization in Enzymatic Hydrolysis of Regenerated Wheat Straw Dissolved by LiCl/DMAc Solvent System, Appl. Biochem. Biotechnol., 2017, 181, 177–191 CrossRef CAS PubMed.
- M. H. Hüsemann and E. T. Papoutsakis, Effects of propionate and acetate additions on solvent production in batch cultures of Clostridium acetobutylicum, Appl. Environ. Microbiol., 1990, 56, 1497 Search PubMed.
- B. Du, L. N. Sharma, C. Becker, S. F. Chen, R. A. Mowery, G. P. van Walsum and C. K. Chambliss, Effect of varying feedstock–pretreatment chemistry combinations on the formation and accumulation of potentially inhibitory degradation products in biomass hydrolysates, Biotechnol. Bioeng., 2010, 107, 430–440 CrossRef CAS PubMed.
- N. Qureshi, B. C. Saha, R. E. Hector, B. Dien, S. Hughes, S. Liu, L. Iten, M. J. Bowman, G. Sarath and M. A. Cotta, Production of butanol (a biofuel) from agricultural residues: Part II–Use of corn stover and switchgrass hydrolysates, Biomass Bioenergy, 2010, 34, 566–571 CrossRef CAS.
|
This journal is © The Royal Society of Chemistry 2019 |
Click here to see how this site uses Cookies. View our privacy policy here.