DOI:
10.1039/C8RA09451A
(Paper)
RSC Adv., 2019,
9, 4488-4498
Biogas production and metal passivation analysis during anaerobic digestion of pig manure: effects of a magnetic Fe3O4/FA composite supplement
Received
16th November 2018
, Accepted 21st January 2019
First published on 5th February 2019
Abstract
Anaerobic digestion has been widely used to produce biogas renewable energy and stabilize fecal manure. In this work, magnetic fly ash composites (Fe3O4/FA) were synthesized and mixed with pig manure in different ratios to study their effects on biogas production and metal passivation during anaerobic digestion. The results showed that the use of 0.5% Fe3O4/FA presented the most positive impact on biogas production compared to anaerobic digestion without Fe3O4/FA, i.e., the total biogas and methane content increased by 13.81% and 35.13%, respectively. Variations in the concentration and speciation of heavy metals (i.e., Cu and Zn) with and without Fe3O4/FA during anaerobic digestion were also analyzed. The concentrations of Cu and Zn increased after anaerobic digestion, showing a significant “relative concentration effect”. Additionally, sequential fractionation suggested that Cu was mainly present in organic matter, whereas Zn was mainly distributed in the oxidation states of iron and manganese. The addition of Fe3O4/FA enhanced the passivation of Cu and Zn in the solid digested residues, i.e., the residual states of Cu and Zn increased by 10.73% to 45.78% and 33.49% to 42.14% compared to the control, respectively. Moreover, better performance was found for the treatment with 2.5% Fe3O4/FA. X-ray diffraction (XRD) and scanning electron microscopy-energy dispersive X-ray spectroscopy (SEM-EDX) analysis demonstrated that Fe3O4/FA deactivated heavy metals mainly via physical adsorption during anaerobic digestion, which can convert them into stable mineral precipitates and thus decrease the solubility and mobility of these metals.
Introduction
In recent years, rapid economic development and improvement of human living standards have stimulated imperative demands for meat and milk, promoting the rapid expansion of the livestock industry. The amount of fecal manure in China reached approximately 38 billion tons in 2016.1 In addition, fecal manure contains numerous heavy metals, such as copper (Cu) and zinc (Zn), due to the addition of heavy metals to feed.2–4 Pig manure can provide indispensable nutrient elements for plants, such as nitrogen and phosphorus; it can be used as an effective alternative to synthetic N and P fertilizers.5 However, it can also cause serious heavy metal pollution of soil when metal-containing fecal manure is continuously introduced to farmland.6,7 These heavy metals can accumulate and enter the soil–water-plant system; this may cause risks to human health via the food chain.
The heavy metal pollution caused by fecal manure has been investigated by researchers worldwide.8,9 Anaerobic digestion was found to be an effective way to control fecal pollution from large-scale livestock and poultry farms.10–12 Different types of digestion catalysts (e.g., fly ash (FA), phosphate, zeolite and biochar) have been added to the anaerobic digestion system to improve biogas production and decrease the bioavailability of heavy metals in fecal manure.13–15 Nanoparticles were also found to have great potential to accelerate the hydrolysis of anaerobic digestion, improve methane yield and stabilize the sludge.16–18 Previous studies reported that FA can decrease the contents of soluble and exchangeable heavy metals via adsorption or changing the pH.19,20 Lu et al. recently found that the combined use of straw, sepiolite, coal fly ash (2.5%) and phosphate rock (5.0%) can result in efficient passivation of Cu during aerobic composting treatment.21 Additionally, enhanced effects of fly ash on the biodegradability and methane production of secondary paper and pulp sludge were reported.22
It has been proved that Fe3O4 nanoparticles can effectively promote methanogenesis through facilitating direct interspecies electron transfer in syntrophic methane production.23 Suanon et al. recently studied the influence of nanoscale zero-valent iron (ZVI) and Fe3O4 on the behavior and fate of heavy metals during anaerobic digestion of sludge.24 Compared with anaerobic digestion without iron nanoparticles, the use of 0.5% iron nanoparticles led to increased biogas production and improved metal stabilization in the digestate. However, to date, information regarding the possible effects of magnetic Fe3O4/FA composites on biogas production and metal passivation during anaerobic digestion of pig manure has remained insufficient.
The current work was designed and performed to evaluate the potential effects of Fe3O4/FA composites on methane production and stabilization of heavy metals (e.g., Cu and Zn) during anaerobic digestion. Pig manure was spiked with Fe3O4/FA composites and treated via an anaerobic digestion process. The basic physiochemical properties during anaerobic digestion were determined, including pH, total solids (TS), volatile solids (VS), ammonium nitrogen (NH4+–N), elemental analysis (C and N), chemical oxygen demand (COD) and methane (CH4) production. The stabilization of heavy metals was assessed based on their distribution in the final product compared to the original pig manure. The modified Gompertz model was used to fit the experimental results and predict the methane yield of each manure sample in anaerobic digestion. Additionally, the mineral composition, morphology and element content of the heavy metal residual fractions before and after anaerobic digestion were characterized to understand the possible mechanism of heavy metal passivation.
Experimental section
Materials and methods
Naturally air-dried pig manure was supplied by a pig farm in Lujiang County (Hefei, Anhui, China). The manure was stored at 4 °C for further use. The inoculum was collected from the anaerobic digestion tank of a sewage treatment plant of a food company in Hefei City (Anhui, China) and domesticated at medium temperature (35 ± 1) °C for about 15 days. Portions of the manure and inoculum were dried in an oven at 105 °C to constant weight and then ground and screened with 200 meshes for physicochemical characterization (Table 1). FA was collected from the Hefei thermal power plant. Ferric sulfate (>96%), ferrous sulfate (>99%), and sodium hydroxide (>96%) were purchased from Sinopharm Chemical Reagents Co. Ltd. (Shanghai, China).
Table 1 Physicochemical characterization of the materialsa
Characteristic |
Pig manure |
Inoculum |
FA |
nd: non-detectable. |
TS (%) |
81.75 ± 1.03 |
8.31 ± 0.07 |
nd |
VS (%) |
70.98 ± 0.34 |
7.76 ± 0.07 |
nd |
pH |
8.06 ± 0.10 |
7.15 ± 1.32 |
8.5 ± 1.21 |
TC (%) |
34.09 ± 3.14 |
30.27 ± 1.49 |
nd |
TN (%) |
2.31 ± 0.21 |
5.06 ± 1.24 |
nd |
C/N |
14.76 ± 2.72 |
5.98 ± 2.76 |
nd |
Cu (mg kg−1) |
273.12 ± 8.64 |
72.10 ± 12.99 |
79.16 ± 3.20 |
Zn (mg kg−1) |
1990.86 ± 14.33 |
492.00 ± 13.89 |
150.32 ± 3.00 |
Preparation of Fe3O4/FA composites
The magnetic Fe3O4/FA composites were synthesized by liquid phase co-precipitation. Briefly, 9.25 g Fe2(SO4)3·nH2O (n = 6 to 9) and 18.29 g FeSO4·7H2O were firstly dissolved in 650 mL and 75 mL deionized water, respectively. The above two solutions were combined and vigorously stirred at 25 °C to 30 °C. FA (25 g, 60 mesh) was suspended in distilled water (250 mL) and then mixed with the above solution. The suspension was slowly stirred at room temperature for 30 min. Subsequently, 10 M NaOH solution was added dropwise to the FA/Fe2+/Fe3+ suspension until the solution pH reached 10.0 to 11.0. After mixing for 60 min, the suspension was aged at room temperature for 24 h, filtered, and then repeatedly washed with distilled water followed by ethanol. The Fe3O4/FA composites were vacuum-filtered and dried under vacuum at 60 °C for 10 h.
Anaerobic digestion and experimental design
Anaerobic digestion experiments were carried out in the laboratory. Four sets of batch experiments were set up: a blank control (CK) and treatments with Fe3O4/FA at different doses (i.e., 0.5%, 1.0% and 2.5% on the basis of TSadded). The dry matter of pig manure was set to 150 g, and a glass bottle with a working volume of 2000 mL was used as the reactor; the inoculation of domesticated sludge was 30% (v/vworking volume). The water addition was adjusted so that the TS load in the digestive system was 8%. All mixtures were homogenous and flushed with nitrogen gas for 5 min to ensure anaerobic conditions before starting the experiments, and the mixtures were placed in a constant temperature incubator (35.0 ± 1.0) °C. The biogas volume was recorded daily by reading the water displacement inside the calibrate glass cylinder in the reactor.
Each reactor was manually mixed three times daily (6:00 am, 14:00 pm and 22:00 pm) to avoid stratification during anaerobic digestion. The pH, NH4+–N, COD and volatile fatty acids (VFAs) of the liquid samples were measured every two or three days. On the completion of digestion (35th d), the samples were collected, followed by solid–liquid separation. The separated liquid was stored in the refrigerator at 4 °C. The solid residues were freeze-dried (ALPHA 1–2 LD plus, Christ, Germany), ground and sieved (100 mesh) for measurements of metal forms.
Analytical methods
A modified Gompertz model was used to estimate the biogas production in the anaerobic digestion system.25,26 The formula is as follows: |
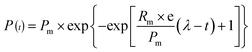 | (1) |
where P(t) is the cumulative gas production at time t (mL g−1 VS); Pm is the potential maximum gas yield (mL g−1 VS); Rm is the maximum gas production rate (mg per VS per d); λ is the lag phase (d); t is the duration of the assay (d) and e is exp(1) = 2.7183.
The bioavailability of heavy metals was expressed as α, representing the ratio of C(a) and C(t). Specifically, C(a) is the sum of the concentrations of exchangeable (C(1)), carbonates (C(2)), ferromanganese oxides (C(3)) and organic compounds (C(4)), and C(t) is the sum of C(a) and the residual concentration (C(5)) in mg kg−1 (dry sludge) (eqn (2)–(4)).27
|
C(a) = C(1) + C(2) + C(3) + C(4)
| (2) |
|
 | (4) |
To measure the total amount of heavy metals, 0.2 g (accurate to 0.0001 g) samples over 100 mesh sieves were digested in a mixture of HNO3, HF and HClO4 (5
:
5
:
3, v/v/v). The contents of Cu and Zn were determined by inductively coupled plasma mass spectrometry (ICP-MS) (7700x, Agilent, USA). The fraction analysis of heavy metals was performed according to the procedures described in,27,28 in which 1 g (accurate to 0.0001 g) sample over 100 mesh sieves was used with the five-step extraction method. The solution was diluted with 1% HNO3 to 50 mL, and the contents of Cu and Zn were measured by ICP-MS.
TS and VS were measured by the weight method. The total carbon and total nitrogen in the raw materials and inoculum were examined by an element analyzer (vario MACRO cube, Germany). The daily gas volume was determined by the saturated salt water method.29 Liquid samples of the anaerobic digestion were taken every two or three days, and the solution pH and COD were individually measured by a pH meter (3C Pro-F, STARTER, China) and the potassium dichromate oxidation method, respectively. The contents of the aqueous VFAs were determined by gas chromatography (7890A, Agilent, USA) coupled with an FID detector. The contents of NH4+–N in solution were measured by the traditional method.30 The percentage of methane gas was measured by a gas chromatograph coupled with a TCD detector (7890A, Agilent, USA). The morphologies and structures of the residual states of the heavy metals were analyzed via SEM (EVO18, ZEISS, Germany) with EDX spectroscopy (XM2, Genesis, USA). The XRD (D8-FOCUS, Bruker, Germany) patterns were acquired on a powder diffraction system with Cu Kα radiation at 40 kV, 40 mA. The samples were scanned from 5° to 80° with a scan speed of 2° min−1.
The seed germination index (GI) is considered to be the most sensitive parameter for the toxicity evaluation of anaerobic digesting mixtures.31 In the present study, 10 Chinese cabbage seeds were placed on gauze moistened with the extracted liquid from the digests. The extract was obtained by adding 50 mL water to 5 g digests, followed by agitating at 200 rpm for 1 h and filtration. The cultures were carried out at 25 °C for 48 h, and the germination rates and root lengths of the seedlings were measured. The GI value was calculated using eqn (5).32 The control group was treated with deionized water.
|
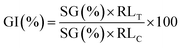 | (5) |
where SG, RL
T and RL
C represent the seed germination, root length of the treated seed and root length of the control, respectively.
Statistical analysis
All experimental values were expressed as the means ± standard deviation (SD). Statistical analysis was performed using the SPSS 19.0 statistical package (Chicago, USA). Statistical differences among different groups were analyzed using one-way analysis of variance (ANOVA) and Dunnett's tests with the significance level set as p < 0.05. The XRD pattern phase identification and analysis results were processed by the analysis software Jade 6.0.
QA/QC
The reagents used in the experiments were all of excellent grade, and the experimental water was ultrapure water. A national soil reference material (GSS-3) was added for quality control. The recoveries of Cu and Zn were 86.96% to 112.45% and 80.27% to 118.78%, respectively; each sample was parallelized by three groups, and three blanks were used for each batch of samples.
Results and discussion
Characterization of FA and Fe3O4/FA
The micro-morphologies of FA and Fe3O4/FA were characterized by SEM images (Fig. 1A and B). As shown in Fig. 1A, FA consisted of ball-like particles with different sizes. Most of the particles were broken into pieces, and the surface became coarse after surface co-precipitation with Fe3O4 (Fig. 1B). EDX analysis suggested that the content of Fe in FA was 4.43%, which sharply increased to 19.89% in Fe3O4/FA composites. XRD analysis also confirmed the successful synthesis of the composites. It was observed that quartz (marked by Q, JCPDS No. 33-1161) was the main mineral composition of FA (Fig. 1C).33 After coating with Fe3O4 (marked by M), three sharp peaks at 2θ = 35.570° (311), 43.191° (400) and 62.928° (662) were strengthened in the diffraction pattern of Fe3O4/FA;34 these peaks match well with the standard spinel structure (JCPDS No. 89-3854). Compared with the initial FA, the diffraction line was much smoother and the small diffraction peaks disappeared after loading Fe3O4, indicating that the Fe3O4/FA composite has a higher degree of crystal order than FA.
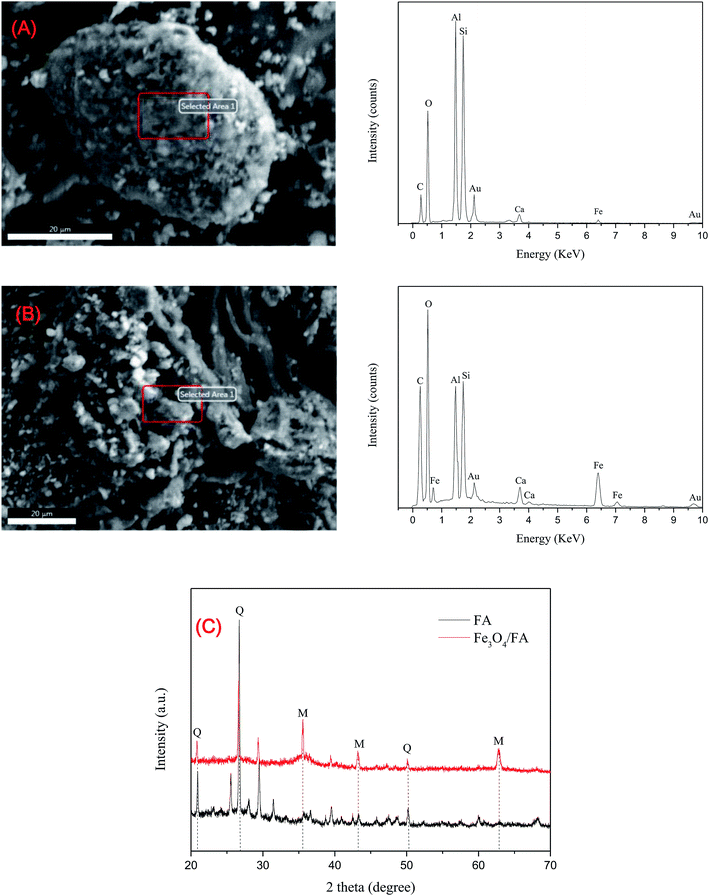 |
| Fig. 1 Characterization of FA and Fe3O4/FA. (A), SEM image and EDX spectrum of FA; (B), SEM image and EDX spectrum of Fe3O4/FA; (C), XRD patterns of FA and Fe3O4/FA. | |
Effects of Fe3O4/FA on biogas production during anaerobic digestion
The daily biogas yield, cumulative gas yield and cumulative volume of methane produced during anaerobic digestion of pig manure are shown in Fig. 2. The daily biogas production increased rapidly during 6 days of anaerobic digestion (Fig. 2A). Two peaks of the biogas yields were observed. The first peak for 0.5% Fe3O4/FA, 1.0% Fe3O4/FA and CK was found on the 6th day, and the values were 2460 mL, 1563 mL and 2132 mL, respectively. The second peak appeared on the 10th day, with individual values of 2418 mL, 2832 mL and 3183 mL, respectively. Two peaks of the daily biogas yield of 2.5% Fe3O4/FA were recorded on the 8th day (2725 mL) and 13th day (1410 mL). It may thus be inferred that a high concentration of Fe3O4/FA exhibits adverse effects on anaerobic digestion and leads to postponement of the gas production peak in anaerobic digestion, as previously mentioned by Yang et al.35 The substrate of digestion is consumed continuously following anaerobic digestion, contributing to the decreased biogas production after the second peak of gas production. The difference in the daily outputs among all the experimental groups decreased with time. The gas production was also decreased on completion of 35 days of digestion.
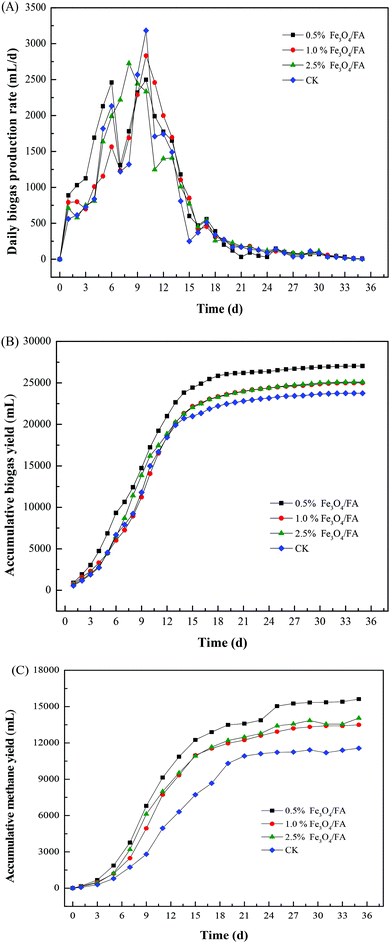 |
| Fig. 2 Daily biogas production (A), cumulative biogas yields (B) and cumulative volume of methane (C) produced within 35 days of anaerobic digestion with and without Fe3O4/FA. | |
The cumulative volume of biogas produced during anaerobic digestion is shown in Fig. 2B. The total volumes of biogas were 180.27, 166.70, 167.33 and 158.38 mL g−1 VS in the digesters with 0.5%, 1.0%, 2.5% Fe3O4/FA and CK, respectively. The results suggested that the production of biogas was increased after the addition of Fe3O4/FA compared to the control, implying obvious stimulation of the biogas by these composites. The increased gas production may be due to the presence of Fe3O4, which plays a critical role in enhancing the syntrophic/cooperative metabolism between electron-donating and electron-accepting microorganisms via direct interspecies electron transfer processes.36,37 Gao et al. reported that Ca2+ was necessary for the formation of methanogens and microbial aggregates during anaerobic digestion.38 The Ca2+ released from CaO in FA during anaerobic digestion may also function to increase biogas generation. Moreover, we found that the addition of low concentrations of Fe3O4/FA significantly stimulated anaerobic digestion, whereas the biogas yields decreased with increasing dose of Fe3O4/FA. Similar phenomena were reported by Suanon,24 in which the total gas production induced by 0.5% nanometer ZVI and 0.5% Fe3O4 was higher than that by 1% nanometer ZVI and 1% Fe3O4 during anaerobic digestion of sludge.
As a key parameter for assessing anaerobic digestion, the biogas production (mainly methane gas production) was recorded every two days (Fig. 2C). The values of the accumulative methane yields were 120.16, 103.85, 108.08 and 88.92 mL g−1 VS for 0.5%, 1.0%, 2.5% Fe3O4/FA and CK, respectively. Comparatively, the methane yield observed in this work is higher than that of anaerobic digestion of pig manure for 20 days (62.50 mL g−1 VS added)39 and in anaerobic digestion of sludge for 12 days (47.18 to 84.27 mL g−1 added VS).24 However, the methane yield in the present study is lower than the results reported by Ferreira et al., who found that the methane yield of the raw pig manure after anaerobic digestion was about 159 mL g−1 added VS.40 This can be attributed to the longer storage time and the lower moisture content of pig manure than those used in this work, which may lead to slow growth of the fermented microorganisms. In addition, fresh pig manure contains large amounts of livestock and poultry urine, which possess large amounts of COD. Therefore, the methane produced during anaerobic fermentation may be decomposed from organic matter in urine and feces; thus, the methane yields in these studies are higher than those in our study. Compared with the control, the methane contents increased by 16.78% to 35.13% after addition of Fe3O4/FA, which further demonstrates its enhancing effects on the anaerobic digestion of pig manure.
Kinetic analysis
Modified Gompertz equations were used to fit the data of the biogas production and methanogenesis processes. The R2 values were >0.99 for all groups (Table 2), indicating good fitness. The addition of Fe3O4/FA could significantly increase the methane yield (p < 0.05) at its optimal ratio of 0.5%. The gas production rate decreased with increasing Fe3O4/FA dosage (Table 2). Therefore, an appropriate concentration of Fe3O4/FA can significantly promote methane production during anaerobic digestion. Moreover, the delay period of anaerobic digestion was shortened after addition of Fe3O4/FA. This is mainly due to stimulation of the electric syntrophy effect between propionate-oxidizing bacteria and methanogens via Fe3O4 particles, which greatly improves the degradation rate of organic acids and the methanogenesis of propionic acid.41–43
Table 2 Kinetics results obtained by the modified Gompertz model
Experimental groups |
Biogas |
Methane |
Hm/mL g−1 VS |
Rm/mL g per VS per d |
λ/d |
R2 |
Hm/mL g−1 VS |
Rm/mL g per VS per d |
λ/d |
R2 |
CK |
182.09 |
17.42 |
3.23 |
0.998 |
89.85 |
6.81 |
5.61 |
0.998 |
0.5% Fe3O4/FA |
208.45 |
18.41 |
2.33 |
0.998 |
117.62 |
9.22 |
3.57 |
0.999 |
1% Fe3O4/FA |
191.35 |
18.02 |
3.11 |
0.998 |
102.23 |
9.14 |
4.72 |
0.999 |
2.5% Fe3O4/FA |
191.60 |
18.33 |
3.04 |
0.999 |
105.07 |
8.66 |
4.00 |
0.998 |
Changes of physicochemical properties during anaerobic digestion
The variations of pH during anaerobic digestion of pig manure are shown in Fig. 3A. In the first 5 days, the pH value of each fermenting tank rapidly decreased from 8.0 to 6.7, and Fe3O4/FA addition slowed the extent of the pH decrease (Fig. 3A). The anaerobic corrosion of Fe3O4/FA could generate OH−, thereby increasing the buffering capacity.44,45
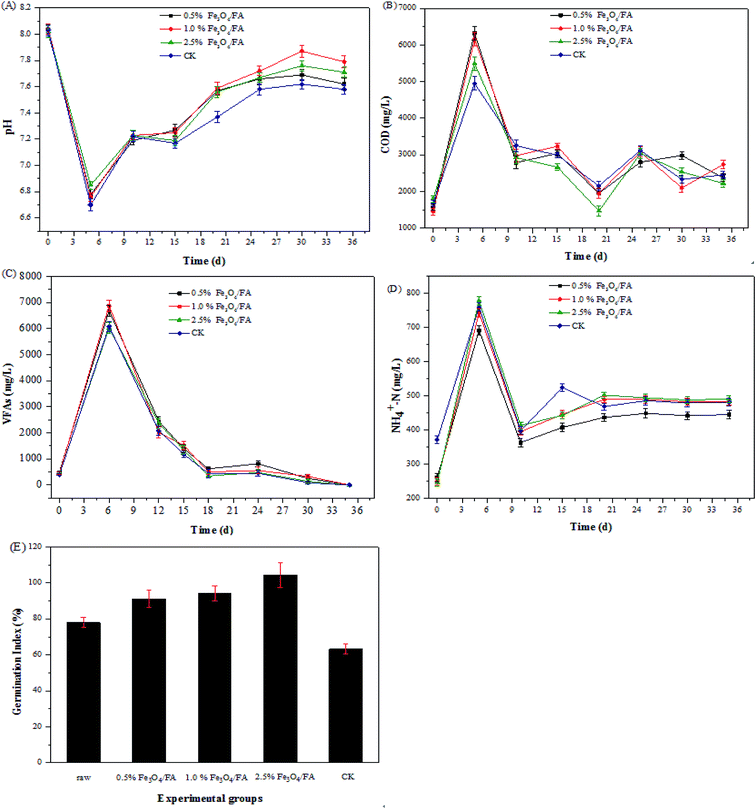 |
| Fig. 3 Effects of different concentrations of Fe3O4/FA on (A) pH, (B) COD, (C) VFAs, (D) NH4+–N and (E) GI value during anaerobic digestion. | |
The rapid decline of pH is due to the accumulation of COD and VFAs (Fig. 3B and C). The maximum COD values reached 6320 mg L−1 for 0.5% Fe3O4/FA, 6156 mg L−1 for 1.0% Fe3O4/FA, 5492 mg L−1 for 2.5% Fe3O4/FA, and 4952 mg L−1 for CK after 5 days of reaction. The additive activation enhanced the anaerobic digestion; the resulting COD values of the Fe3O4/FA-added groups were higher than that of the CK group. Gradually, the COD values in all experimental treatments showed a decreasing trend with further anaerobic digestion (after the 5th day).
The contents of VFAs reached the maximum values after 6 days of anaerobic digestion, i.e., 6685.16, 6875.88, 6035.65 and 6088.32 mg L−1 for the 0.5%, 1.0%, and 2.5% Fe3O4/FA groups and the CK group, respectively. The biogas digestion process was suppressed when the content of VFAs was greater than 6 g L−1.46 Therefore, there was no acid inhibition in this experiment. Ammonium nitrogen (NH4+–N) can inhibit microbial activity in pig manure anaerobic digestion.47 The contents of ammonium nitrogen ranged from 200 to 800 mg L−1 for the four experimental groups (Fig. 3D). The content of NH4+–N had no antagonistic effect on anaerobic bacteria in the range of 200 to 1000 mg L−1;48 therefore, there was no inhibition of NH4+–N during anaerobic digestion of pig manure in this work.
In addition, the phytotoxic effects of the final digest product were evaluated using the seed germination test. A GI value greater than 50% was used as an indicator of the phytotoxic-free compost,49 and a GI value greater than 80% was considered to indicate the mature compost.50 As presented in Fig. 3E, the seed GI was in the range of 91.18% to 104.41% for the three groups with addition of Fe3O4/FA, which is higher than the ranges of the raw (77.94%) and CK groups (63.24%). These results indicate that the addition of appropriate contents of Fe3O4/FA can improve the quality of digest.
Passivation of Cu and Zn during anaerobic digestion
Our results demonstrated that Cu and Zn were released into the liquid phase during anaerobic digestion of pig manure (Fig. 4). The aqueous concentrations of Cu and Zn in the CK group were 1.846 and 8.919 mg L−1, respectively, suggesting that 17.25% Cu and 11.93% Zn remained in the liquid phase. Comparatively, less than 10% of these two heavy metals in all of the experimental groups with Fe3O4/FA (0.5%, 1.0% and 2.5%) were released into the solution (Fig. 4). The release of metals in the solution can be attributed to the decomposition of organic matter and related compounds in pig manure.51–53 Because only very low concentrations of Cu and Zn were detected in the liquid phase, the metals appeared to be immobilized within the pig manure in the groups with 0.5%, 1.0% and 2.5% Fe3O4/FA (Fig. 4). This may be due to the good adsorption properties of the Fe3O4/FA added during digestion and the formation of a hydrated oxide covering layer on the surface of the particles, which enhanced the adsorption of heavy metal ions.54,55 The release rates of Cu are higher than that of Zn (Fig. 4). Heavy metals have inhibitory effects on anaerobic digestion,56 and previous studies suggested that biogas production is severely affected when using concentrations of CuO and ZnO nanoparticles greater than 15 mg L−1 and 120 mg L−1, respectively.56 Our results indicated that a higher concentration of Fe3O4/FA added during digestion can decrease the release of Cu and Zn and therefore result in lower potential inhibitory effects on microbial activity during anaerobic digestion.
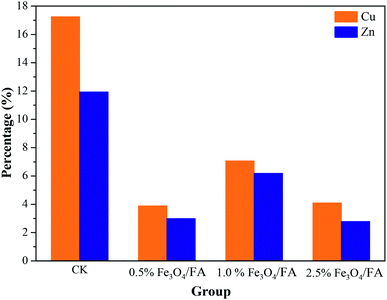 |
| Fig. 4 The contents of Cu and Zn in the liquid phase. | |
The concentrations of Cu and Zn in the solid mixture were 273.43 and 1916.30 mg kg−1 before anaerobic digestion, respectively. After 35 days of anaerobic digestion, the concentrations of Cu and Zn in the solid phase were within the ranges of 295.21 to 352.35 and 2067.65 to 2431.61 mg kg−1, respectively, i.e., about 1.08 to 1.29 and 1.08 to 1.27 times those in the initial solid mixtures. These results suggest a significant “relative concentration effect”,57 and the increased concentrations of heavy metals after anaerobic digestion may be due to the consumption of organic matter.58
According to Tessier et al.,28 the speciation of heavy metals can be divided into five types: (1) the exchangeable fraction, which is the most easily released into the environment, resulting in heavy metal pollution; (2) the carbonate fraction, which is sensitive to pH and readily released into the environment at low pH; (3) the Fe–Mn oxide-bound fraction; (4) the organic combination state, which is not stable when the redox conditions are changed; (5) the residual fraction, which is the most stable. The fraction distributions of Cu and Zn after anaerobic digestion are shown in Fig. 5; Cu was mainly present in organic matter, and its levels increased from 56.24% (in the initial pig manure) to 62.68% to 73.17% (after anaerobic digestion) (Fig. 5A), which may be due to the high affinity of Cu to humic acids during anaerobic digestion.59,60 Previous studies suggested that Cu can be immobilized by complexation and chelation with organic matter, which decreased the bioavailability of Cu to crops.61 Compared with raw pig manure, the contents of the exchangeable, carbonate, and Fe–Mn oxide fractions of Cu all decreased in the solid phase after anaerobic digestion. Meanwhile, compared to the CK group, the residual fraction of Cu significantly increased, i.e., from 22.28% for CK to 22.28% to 30.55% for the three groups with the addition of Fe3O4/FA (p < 0.05). Moreover, the residual state increased with increasing addition of Fe3O4/FA. Therefore, the present results indicate that addition of Fe3O4/FA can decrease the bioavailability of Cu during anaerobic digestion of pig manure.
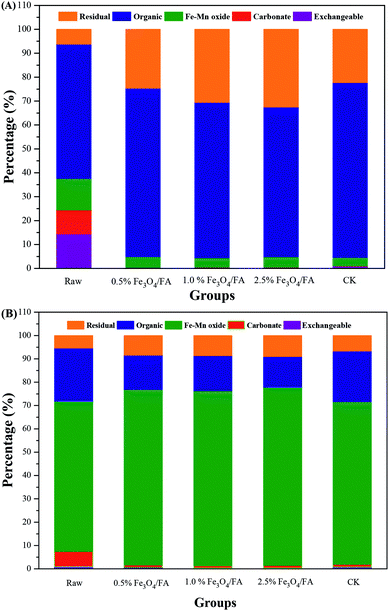 |
| Fig. 5 Chemical fractionation of Cu (A) and Zn (B). | |
Additionally, the effects of different concentrations of Fe3O4/FA on the morphology of Zn are presented in Fig. 5B. Zn were mainly distributed in the Fe–Mn oxide-bound fraction, i.e., 64.75%, 75.13%, 74.92%, 76.33% and 69.90% for raw pig mature with 0.5%, 1.0%, and 2.5% Fe3O4/FA and CK, respectively. Compared with the CK, the residual state of Zn in the biogas residue increased remarkably (p < 0.05), and the additions of 2.5% and 0.5% Fe3O4/FA increased it the most (42.14%) and the least (33.49%), respectively.
The value of α was calculated by eqn (4) and can reflect the bioavailability of Cu and Zn. As shown in Fig. 6, the bioavailability of Cu and Zn decreased compared to the initial pig manure, and the values decreased from 0.94 to 0.68 to 0.78 for Cu and from 0.95 to 0.91 to 0.93 for Zn. The α values of Cu and Zn in the Fe3O4/FA-treated groups individually decreased by 3.08% to 13.12% and 1.93% to 2.52% compared with CK. Moreover, the bioavailability of Cu was more obvious than that of Zn.
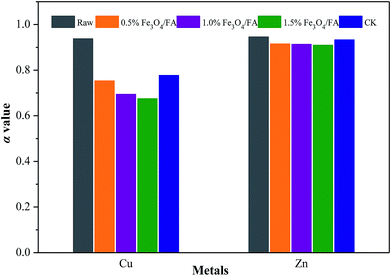 |
| Fig. 6 Variation of α values before and after anaerobic digestion. | |
The above results indicate that the use of Fe3O4/FA under anaerobic digestion conditions can effectively decrease the contents of highly reactive Cu and Zn (exchangeable fraction and carbonate fraction) and increase the residue content with the lowest activity. Moreover, the passivation effect is more obvious with higher concentrations of Fe3O4/FA. This may be due to the adsorption and alkaline effects of the fly ash surface62 and the direct interspecies electron transfer of Fe3O4/FA particles supported on the surface of fly ash to enhance the biodegradation of organic matter, thereby changing the distribution of Cu and Zn. The increased content of residual Cu and Zn enhanced the passivation effect and decreased their bioavailability.
Characterization of the residual fractions of Cu and Zn during anaerobic digestion
In order to reveal the possible mechanism of heavy metal passivation during anaerobic digestion, XRD was performed to determine the mineral phases of the heavy metal residue fractions before and after anaerobic digestion. As shown in Fig. 7, the heavy metal residue fractions of the initial pig manure (raw), CK and 2.5% Fe3O4/FA-treated groups were analyzed. The results suggest that the mineral phases in the residue fractions of the three groups were mainly silicate minerals. The XRD maps of the heavy metal residues of each group are very similar. Compared to the XRD map of the raw sample, the peaks at 2θ = 14.280° (d = 0.619 nm), 26.610° (d = 0.334 nm), 27.499° (d = 0.324 nm), 59.949° (d = 0.154 nm) and 68.121° (d = 0.137 nm) were obviously weakened in the residue fractions of the CK and 2.5% Fe3O4/FA groups. The above five peaks were attributed to CaCO3, (K,H3O)Al2Si3AlO10(OH)2, Ca2SiO4, CaMgSiO4 and SiO2 via phase identification, respectively. Hydrolysis of these minerals during anaerobic digestion may result in decreased self-activity. Compared with the XRD spectrum of the Raw sample, the peak intensities of 2θ = 8.830° (d = 1.000 nm) and 12.500° (d = 0.707 nm), representing (K,H3O)Al2Si3AlO10(OH)2 and K2Mg2Si2O30, respectively, in the residues of the Fe3O4/FA-treated groups were obviously decreased. It was speculated that the addition of Fe3O4/FA partly improves the hydrolysis of these clay minerals and thus transforms them into more stable minerals. In addition, a strong additional peak was located at 2θ = 28.009° (d = 0.318 nm, assigning as CaZnSiO4H2O). The silicate was wrapped with low-solubility minerals by physical adsorption with the addition of Fe3O4/FA composites in the anaerobic fermenting tank. However, we could not detect Cu minerals in the XRD analysis; this may be because of the small amount of Cu for the formation of new amorphous metallic minerals.
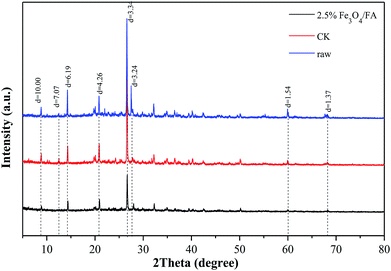 |
| Fig. 7 Powder XRD patterns of heavy metal residues before and after anaerobic digestion. | |
SEM-EDX was also used to observe the residual states of Cu and Zn before and after anaerobic digestion (Fig. 8). The results showed that the surface of the residue state was smoother after anaerobic digestion, and the treatment with 2.5% Fe3O4/FA was more obvious (Fig. 8C). This is probably due to the fact that the silicate minerals formed by heavy metals and other unknown crystals produced a surface coating on the residue during anaerobic digestion; they can also cover the heavy metal ions and prevent their leakage. In addition, EDX analysis showed that Cu and Zn were observed on the surface of the solid sample after anaerobic digestion. Compared with the initial pig manure, the contents of Cu and Zn were increased in the Fe3O4/FA-treated group, and the highest values of the two metals were found in the 2.5% Fe3O4/FA-treated group. Therefore, it can be inferred that the passivation mechanism of the Fe3O4/FA composites mainly via physical adsorption of Fe and Mn oxides in Fe3O4/FA. This physical adsorption can transform heavy metals into metal minerals with poor solubility and migration properties.
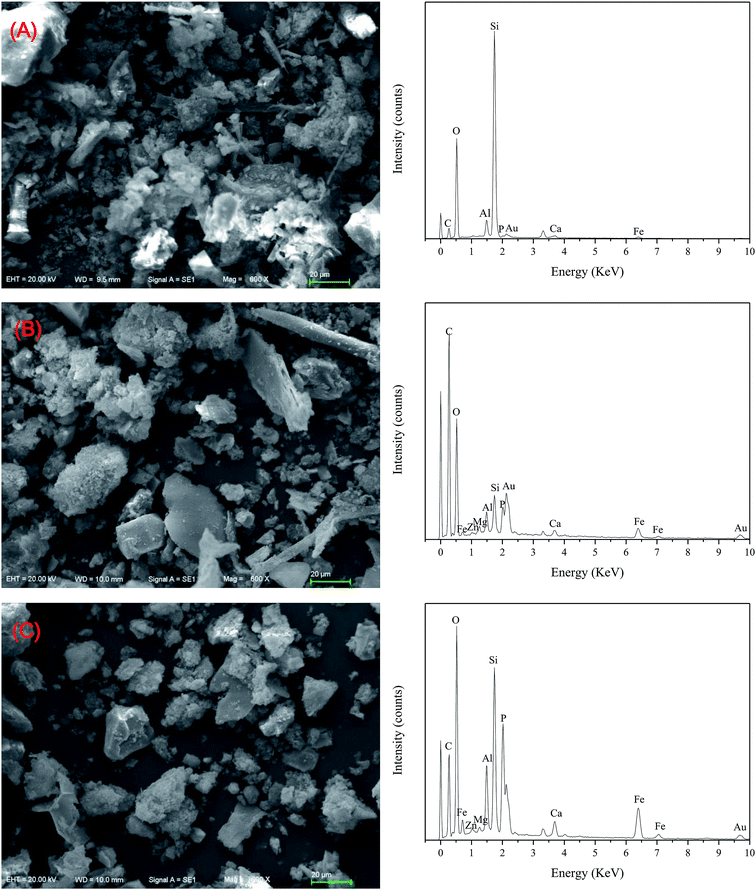 |
| Fig. 8 SEM-EDX images of the heavy metal residues before and after anaerobic digestion. (A) Initial pig manure (raw); (B) digester blank control (CK); (C) digester 2.5% Fe3O4/FA. | |
Conclusion and outlook
In this work, the gas production and distribution of heavy metals were analyzed during anaerobic digestion of pig manure treated with Fe3O4/FA composite. Our results suggested that the addition of Fe3O4/FA with different concentrations could promote methane production in the anaerobic digestion system with the optimal dose of 0.5% Fe3O4/FA. The introduction of Fe3O4/FA composites could effectively control the mobilization of heavy metals; also, the concentrations of Cu and Zn increased after anaerobic digestion, presenting a significant “relative concentration effect”. Morphological analysis suggested that the addition of Fe3O4/FA enhanced the passivation of Cu and Zn in the solid digested residues, i.e., the residual states of Cu and Zn were increased by 10.73% to 45.78% and 33.49% to 42.14%, respectively. Moreover, better performance was observed in the treatment with 2.5% Fe3O4/FA.
XRD and SEM-EDX analysis demonstrated that the mechanism of Fe3O4/FA for passivating heavy metals mainly involves physical adsorption during the anaerobic digestion. It can convert heavy metals into stable mineral precipitates, decreasing the solubility and mobility of the heavy metals and thus effectively passivating them. These findings may be beneficial to provide a theoretical basis for decreasing the heavy metal activity of livestock manure and decreasing the risk of heavy metal release. More studies are needed to fully address the underlying mechanisms of heavy metal passivation.
Conflicts of interest
There are no conflicts to declare.
Acknowledgements
This work was supported by the National Water Pollution Control and Treatment Science and Technology Major Project in China (2017ZX07603002), National Natural Science Foundation of China (No. 21607001 and 41172121), the Anhui Provincial Natural Science Foundation (No. 1608085QB45) and the Anhui Province Key Laboratory of Wetland Ecosystem Protection and Restoration (Anhui University).
Notes and references
- Y. J. Shen, L. X. Zhao, H. B. Meng, Y. Q. Hou, H. B. Zhou, F. Wang, H. S. Cheng and H. B. Liu, Waste Manage. Res., 2016, 34, 578–583 CrossRef CAS PubMed.
- C. E. Marcato, E. Pinelli, M. F. Cecchi, P. Winterton and M. Guiresse, Ecotoxicol. Environ. Saf., 2009, 72, 1538–1544 CrossRef CAS PubMed.
- F. S. Zhang, Y. X. Li, M. Yang and W. Li, Int. J. Environ. Res. Public Health, 2012, 9, 2658–2668 CrossRef CAS PubMed.
- F. Wang, L. X. Zhao, Y. J. Shen, H. B. Meng, X. Xiang, H. S. Cheng and Y. Luo, Trans. Chin. Soc. Agric. Eng., 2013, 29, 202–208 Search PubMed.
- G. Q. Peng, G. M. Tian and J. Z. Liu, Desalination, 2011, 271, 100–104 CrossRef CAS.
- J. C. Shi, X. L. Yu, M. K. Zhang, S. G. Lu, W. H. Wu, J. J. Wu and J. M. Xu, J. Environ. Qual., 2011, 40, 1695–1704 CrossRef CAS PubMed.
- Y. Huang, H. Dong, B. Shang, H. Xin and Z. Zhu, Appl. Energy, 2011, 88, 947–952 CrossRef CAS.
- Y. Xu, W. Yu, Q. Ma and H. Zhou, Plant, Soil Environ., 2013, 59, 492–499 CrossRef.
- B. Dong, X. G. Liu, L. Dai and X. Dai, Bioresour. Technol., 2013, 131, 152–158 CrossRef CAS PubMed.
- W. Liu, R. Huo, J. X. Xu, S. X. Liang, J. J. Li, T. K. Zhao and S. T. Wang, Bioresour. Technol., 2017, 235, 43–49 CrossRef CAS PubMed.
- T. T. T. Cu, P. H. Cuong, L. T. Hang, N. V. Chao, L. X. Anh, N. X. Trach and S. G. Sommer, J. Cleaner Prod., 2012, 27, 64–71 CrossRef.
- X. Y. Jiang, S. G. Sommer and K. V. Christensen, Energy Policy, 2011, 39, 6073–6081 CrossRef.
- K. Gondek, M. Mierzwa-Hersztek and M. Kopeć, J. Environ. Manage., 2018, 222, 132–134 CrossRef PubMed.
- D. Lu, L. X. Wang, B. X. Yan, Y. Ou, J. N. Guan, Y. Bian and Y. B. Zhang, Waste Management, 2014, 34, 1529–1536 CrossRef CAS PubMed.
- J. Singh, R. Prasad and A. S. Kalamdhad, Res. J. Chem. Environ., 2013, 17, 26–34 CAS.
- Y. W. Liu, Y. B. Zhang, X. Quan, Y. Li, Z. Q. Zhao, X. S. Meng and S. Chen, Chem. Eng. J., 2012, 192, 179–185 CrossRef CAS.
- Y. H. Feng, Y. B. Zhang, X. Quan and S. Chen, Water Res., 2014, 5, 242–250 CrossRef PubMed.
- H. J. Li, J. L. Chang, P. F. Liu, L. Fu, D. W. Ding and Y. H. Lu, Environ. Microbiol., 2015, 17, 1533–1547 CrossRef PubMed.
- J. Q. Xu, R. L. Yu, X. Y. Dong, G. R. Hu, X. S. Shang, Q. Wang and H. W. Li, J. Hazard. Mater., 2012,(217–218), 58–66 CrossRef PubMed.
- J. Sheng, W. J. Lu and H. T. Wang, J. Environ. Sci., 2007, 28, 1367–1371 CAS.
- X. M. Lu, P. Z. Lu, J. J. Chen, H. Zhang and J. Fu, Environ. Sci. Pollut. Res., 2015, 22, 14727–14737 CrossRef CAS PubMed.
- C. Huiliñir, S. Montalvo and L. Guerrero, Water Sci. Technol., 2015, 72, 230–237 CrossRef PubMed.
- H. J. Li, J. L. Chang, P. F. Liu, L. Fu, D. W. Ding and Y. H. Lu, Environ. Microbiol., 2015, 17, 1533–1547 CrossRef PubMed.
- F. Suanon, Q. Sun, D. Mama, J. W. Li, B. Dimon and C. P. Yu, Water Res., 2016, 88, 897–903 CrossRef CAS PubMed.
- M. H. Zwietering, I. Jongenburger, F. M. Rombouts and 'R. K. Van, Appl. Environ. Microbiol., 1990, 56, 1875–1881 CAS.
- Y. Mu, X. J. Zheng, H. Q. Yu and R. F. Zhu, Int. J. Hydrogen Energy, 2006, 31, 780–785 CrossRef CAS.
- Y. S. Liu, L. L. Ma, Y. Q. Li and L. T. Zheng, Chemosphere, 2007, 67, 1025–1032 CrossRef CAS PubMed.
- A. Tessier, P. G. Campbell and M. Bisson M, Anal. Chem., 1979, 51, 844–851 CrossRef CAS.
- Y. G. Liang, S. S. Yin, Y. B. Si, Z. Zheng, S. J. Yuan, E. Nie and X. Z. Luo, Chem. Eng. J., 2014, 237, 209–216 CrossRef CAS.
- APHA A WEF, Standard methods for the examination of water and wastewater, American Public Health Association, Washington, DC, 19th edn, 1995 Search PubMed.
- H. B. Zhou, H. B. Meng, L. X. Zhao, Y. J. Shen, Y. Q. Hou, H. S. Cheng and L. Q. Song, Bioresour. Technol., 2018, 258, 279–286 CrossRef CAS PubMed.
- S. Meunchang, S. Panichsakpatana and R. W. Weaver, Bioresour. Technol., 2005, 96, 437–442 CrossRef CAS PubMed.
- Q. Zhou, C. J. Yan and W. J. Luo, Mater. Des., 2016, 92, 701–709 CrossRef CAS.
- L. D. Garfield, D. Dixon, P. Nowotny, F. E. Lotrich, B. G. Pollock, S. D. Kristjansson, P. M. Doré and E. J. Lenze, Biomass Bioenergy, 2014, 71, 299–310 CrossRef.
- Y. Yang, J. L. Guo and Z. Q. Hu, Water Res., 2013, 47, 6790–6800 CrossRef CAS PubMed.
- F. Aulenta, S. Fazi, M. Majone and S. Rossetti, Process Biochem., 2014, 49, 2235–2240 CrossRef CAS.
- V. C. Cruz, S. Rossetti, S. Fazi, P. Paiano, M. Majone and F. Aulenta, Environ. Sci. Technol., 2014, 48, 7536–7543 CrossRef PubMed.
- P. C. Gao, X. B. Tang, Y. N. Tong and Y. X. Chen, Waste Management, 2008, 28, 1630–1636 CrossRef CAS PubMed.
- C. Shi, X. D. Liao, Y. B. Wu and J. B. Liang, Anim. Feed Sci. Technol., 2011, 166, 457–463 CrossRef.
- L. Ferreira, T. Souza, F. Fdz-Polanco and S. Pérez-Elvira, Bioresour. Technol., 2014, 152, 393–398 CrossRef CAS PubMed.
- C. Viggi, S. Rosserri, S. Fazi, P. Paiano, M. Majione and F. Aulenta, Environ. Sci. Technol., 2014, 48, 7536–7543 CrossRef PubMed.
- E. Baek, J. Kim and C. Lee, Bioresour. Technol., 2016, 222, 344–354 CrossRef PubMed.
- C. Yamada, S. Kato, Y. Ueno, M. Ishii and Y. Igarashi, J. Biosci. Bioeng., 2015, 119, 678–682 CrossRef CAS PubMed.
- G. Y. Zhen, X. Y. Lu, Y. Y. Li, Y. Liu and Y. C. Zhao, Chem. Eng. J., 2015, 263, 461–470 CrossRef CAS.
- X. Kong, Y. H. Wei, S. Xu, J. G. Liu, H. Li, Y. L. Liu and S. Y. Yu, Bioresour. Technol., 2016, 211, 65–71 CrossRef CAS PubMed.
- I. Siegert and C. Banks, Process Biochem., 2005, 40, 3412–3418 CrossRef CAS.
- Y. G. Orha and B. Demirel, Process Biochem., 2013, 48, 901–911 CrossRef.
- R. Rajagopal, D. I. Massé and G. Singh, Bioresour. Technol., 2013, 143, 632–641 CrossRef CAS PubMed.
- F. Zucconi, M. Forte, A. Monac and M. Beritodi, BioCycle, 1982, 22, 27–29 Search PubMed.
- Y. S. Wei, Y. B. Fan, M. J. Wang and J. S. Wang, Resour., Conserv. Recycl., 2000, 30, 277–300 CrossRef.
- L. Appels, J. Baeyens, J. Degreve and R. Dewil, Prog. Energy Combust. Sci., 2008, 34, 755–781 CrossRef CAS.
- F. Li, Z. Li, P. Mao, Y. W. Li, Y. X. Li, M. B. McBride, J. T. Wu and P. Zhuang, Environ. Sci. Pollut. Res., 2018, 9, 1–10 Search PubMed.
- N. M. Zhu, Q. L. Li, X. J. Guo, H. Zhang and Y. Deng, Ecotoxicol. Environ. Saf., 2014, 102, 8–24 CrossRef PubMed.
- P. Xu, G. M. Zeng, D. L. Huang, C. L. Feng, S. Hu, M. H. Zhao, C. Lai, Z. Wei, C. Huang, G. X. Xie and Z. F. Liu, Sci. Total Environ., 2012, 424, 1–10 CrossRef CAS PubMed.
- S. C. N. Tang and I. M. C. Lo, Water Res., 2013, 47, 2613–2632 CrossRef CAS PubMed.
- M. Luna-Delrisco, K. Qrupõld and H. C. Dubourguier, J. Hazard. Mater., 2011, 189, 603–608 CrossRef CAS PubMed.
- L. Dąbrowska and A. Rosińska, Chemosphere, 2012, 88, 168–173 CrossRef PubMed.
- H. M. Jin and Z. Z. Chang, Appl. Biochem. Biotechnol., 2011, 164, 268–282 CrossRef CAS PubMed.
- Y. G. Liang, X. J. Li, J. Zhang, L. G. Zhang and B. J. Cheng, Environ. Sci. Pollut. Res., 2017, 24, 12328–12337 CrossRef CAS PubMed.
- X. Xiong, Y. X. Li, J. Han, C. Y. Lin, C. Suo and Z. Q. Zhang, J. Agro-Environ. Sci., 2008, 27, 2137–2142 CAS.
- E. Donner, C. G. Ryan, D. L. Howard, B. Zarcinas, K. G. Scheckel, S. P. McGrat, M. D. Jonge, D. Paterson, R. Naidu and E. Lombi, Environ. Pollut., 2012, 166, 57–64 CrossRef CAS PubMed.
- Z. T. Yao, X. S. Ji, P. K. Sarker, J. H. Tang, L. Q. Ge, M. S. Xia and Y. Q. Xi, Earth-Sci. Rev., 2015, 141, 105–121 CrossRef.
|
This journal is © The Royal Society of Chemistry 2019 |
Click here to see how this site uses Cookies. View our privacy policy here.