DOI:
10.1039/C8RA09518C
(Paper)
RSC Adv., 2019,
9, 11263-11271
Dominance of Candidatus saccharibacteria in SBRs achieving partial denitrification: effects of sludge acclimating methods on microbial communities and nitrite accumulation
Received
19th November 2018
, Accepted 1st April 2019
First published on 10th April 2019
Abstract
Partial denitrification (NO3−-N → NO2−-N) was combined with anaerobic ammonium oxidation (ANAMMOX) to achieve nitrogen removal with a low C/N ratio and low energy consumption. Three different acclimation conditions, namely, R1 (sequencing batch reactor (SBR) under anoxic conditions), R2 (SBR under alternating anoxic/aerobic conditions), and R3 (SBR under low-intensity aeration), were investigated using glucose as an electron donor to achieve continuous accumulation of nitrite during a 120 d run. Subsequently, the denitrification performance and microbial community structure of the sludge were investigated. The results showed that the acclimatized sludge in reactors R2 and R3 achieved better partial denitrification performance than the sludge in R1 due to the presence of dissolved oxygen as a result of aeration. Notably, the R3 reactor had the optimal conditions for nitrite accumulation. The high-throughput sequencing analysis indicated that the dominant bacteria in R2 and R3 were Candidatus saccharibacteria with a relative abundance of 45.44% and 34.96%, respectively. This was the first time that Candidatus saccharibacteria was reported as the dominant bacteria in denitrifying sludge. The microbial diversity of the R1 reactor was much greater than that of R2 and R3, indicating that a larger proportion of denitrifying bacteria were present in the R2 and R3 reactors. In addition, the batch experiments showed that the higher the initial pH, the higher the nitrite accumulation rate was.
1. Introduction
The anaerobic ammonium oxidation (ANAMMOX) process has been recognized as the most effective and promising method for nitrogen removal. At present, the nitrite needed as an electron acceptor in the ANAMMOX process is mainly derived from partial nitrification (NH4+-N → NO2−-N) in the Single reactor for High activity Ammonia Removal Over Nitrite (SHARON)-ANAMMOX process and the Completely Autotrophic Nitrogen Removal over Nitrite (CANON) process. However, it is difficult to ensure the stable and long-term operation of the reactors because autotrophic bacteria, such as ammonia oxidizing bacteria (AOB), nitrite-oxidizing bacteria (NOB), and ANAMMOX bacteria are vulnerable to environmental factors. Therefore, it is necessary to find new pathways to obtain the nitrite required for biological nitrogen removal in the ANAMMOX process.
The accumulation of nitrite during the denitrification process has been reported frequently;1–4 nitrite is harmful to human health and inhibits microorganism in the nitrogen and phosphorus removal process. Therefore, the combination of ANAMMOX and heterotrophic denitrification with NO2−-N accumulation may be a promising and effective approach for the removal of nitrogen. Kalyuzhnyi et al.5 proposed a new denitrification process called DEnitrifying AMmonium OXidation (DEAMOX), in which the ANAMMOX and complete denitrification processes occur in a single reactor. However, it was difficult to prevent the reduction of nitrite to N2. Thus, Du et al.6 established a novel DEAMOX process by coupling ANAMMOX with partial denitrification. Partial denitrification was achieved using two different carbon sources, i.e., acetate and ethanol, which had nitrate to nitrite transformation ratios of 95.8% and 90.0%, respectively. The improvement of the accumulation rate and the extension of the accumulation time of nitrite by controlling environmental conditions represent key objectives to achieve partial denitrification. Almeida et al.7 assumed that the competition for electrons between nitrite reductase and nitrate reductase led to different reduction rates and ultimately caused the accumulation of nitrite. Blaszczyk et al.8 indicated that the delayed synthesis of nitrite reductase compared to nitrate reductase, i.e., the difference in the activity between nitrite reductase and nitrate reductase, led to the accumulation of nitrite. Several environmental factors affect the accumulation of nitrite, including the C/N ratio, the carbon source type, pH, and salinity. Sun et al.9 used denitrifying sludge to treat pre-treated landfill leachate in an anoxic/anaerobic upflow anaerobic sludge bed (UASB). The activated sludge was analyzed to investigate the effects of the C/N ratios and types of carbon sources on the nitrite accumulation during the denitrification. The stoichiometry of the denitrification indicated that nitrite accumulation occurred due to an insufficient carbon source required for the reduction of nitrite to nitrogen gas when the C/N ratio was below the theoretical threshold of 3.75. Additionally, nitrite accumulation was observed when methanol, ethanol, sodium acetate, and sodium propionate were used as carbon sources. The concentrations of nitrite and the time when the nitrite accumulation peaked were different in different media. Le et al.10 investigated the impact of different carbon sources (acetate, glycerol, methanol, and ethanol) and the C/N ratio on partial denitrification selection for short- and long-term operations. All carbon sources supported partial denitrification in the short-term but in long-term operations, the contribution of partial denitrification to nitrate removal was greater than 90% in reactors using acetate or glycerol as carbon sources. In addition, the C/N ratio affected the nitrate reduction rate to control partial denitrification; therefore the C/N ratio was used to balance the effectiveness of denitrification and ANAMMOX. Qian et al.11 found that high pH values were beneficial for partial denitrification; three partial denitrification reactors were operated for 420 d with influent pH values of 5.0, 7.0, and 9.0. The experimental results showed that the nitrite accumulation rates (NARs) increased with increasing pH and were 21%, 38%, and 57%, respectively. Conversely, Cao et al.12 reported that the nitrate and nitrite reduction rates were inhibited by high pH values but the nitrate reduction was more vulnerable to a high pH. Chen et al.13 stated that salinity decreased the nitrogen removal efficiency of activated sludge and increased the extracellular polymeric substance (EPS) content. Li et al.14 described an efficient method that increased the influent salinity for enriching the community of partial denitrifiers. The nitrite accumulation efficiency was higher than 75%, the nitrate conversion efficiency was high, and Halomonas was the dominant genus. Ji et al.15 investigated the effect of salinity on the partial-denitrification performance and the microbial community structures of partial-denitrification. The results showed that the denitrifying activity of the non-domesticated sludge was completely suppressed by a high salinity of 1.5 wt%, whereas stable and high nitrite accumulation was achieved after 120 d of operation and the nitrate-to-nitrite transformation ratio (NTR) was about 90%. Dissolved oxygen (DO) is also a critical factor affecting nitrite accumulation and has attracted increased attention in recent years. Gayle et al.16 found that nitrite reductase was strongly inhibited by oxygen. Nitrite accumulation occurred in the presence of oxygen. Both nitrate reductase and nitrite reductase were inhibited in the presence of DO. However, the latter was more sensitive to O2, which resulted in the accumulation of nitrite. The same phenomenon was also reported by Coyne17 in a study of denitrifying enzyme synthesis and activity in Achromobacter cycloclastes. Nitrate reductase activity was observed at all O2 concentrations, whereas the N2O reductase protein was not active until the O2 concentrations decreased to 23 μM, i.e., nitrite reduction was the most O2-sensitive denitrifying step in the denitrification process.
To date, few studies have focused on the determination of the mechanisms of NO2−-N accumulation and the microbial community change under different cultivation conditions with or without aeration during the denitrification process. In the present study, batch experiments were carried out to compare the nitrite accumulation during the denitrification process for three different cultivation conditions with and without aeration (DO) to achieve partial denitrification using glucose as a carbon source. Illumina high-throughput sequencing analysis was conducted to investigate the changes in the microflora in the reactors with different cultivation conditions with and without the presence of DO. The effect of various initial pH values on the nitrite accumulation during the denitrification process was also investigated.
2. Materials and methods
2.1 SBR set up and operation
Three parallel cylindrical sequencing batch reactor (SBRs) were used with a working volume of 4.0 L at a constant temperature of about 25 °C. Synthetic wastewater (2.0 L) was pumped into each SBR reactor during every cycle with a water fill ratio of 1/2. The reactors were mixed using a mechanical stirrer at 90 rpm during the reaction stage. The reactors were operated at two cycles per d and each cycle was operated for 6 h during the 120 d of operation. The reaction cycles of the reactors are listed in Table 1. The reactor operation consisted of two stages. The first stage was the start-up stage (0–10 d), during which the C/N ratio was maintained at 6 to improve the denitrification performance of the sludge. Subsequently, the C/N ratio was changed to 3 to acclimate the sludge to achieve partial denitrification. The sludge concentration (mixed liquor suspended solids, MLSS) was maintaining at 1500 ± 20 mg L−1 by conducting regular sludge discharges during the 120 d operation period.
Table 1 The operation modes of reactor R1, R2 and R3
Reactors |
Operation modes |
R1 |
4.5 h anoxic reaction stage, 1.5 h settling stage |
R2 |
3 cycles of 1 h anoxic reaction stage, 0.5 h aerobic reaction stage (DO = 2 mg L−1) using micropore aeration, finally 1.5 h settling time |
R3 |
3 h aerobic reaction stage with low dissolved oxygen (DO = 0.1–0.2 mg L−1), 1.5 h anoxic reaction stage, finally 1.5 h settling time |
The inoculated sludge was obtained from a sewage treatment plant in Beijing, China. The synthetic wastewater contained NO3−-N and a mineral solution, which consisted of 182.1 mg L−1 NaNO3 (30 mg L−1 NO3−-N), 0.5 mg L−1 KH2PO4, 82 mg L−1 MgSO4·7H2O, 220 mg/LCaCl2·2H2O, 300 mg L−1 NaHCO3, and 1.25 mL trace element solution. The trace element solution contained (g L−1) 1.5 FeCl3·6H2O, 0.03 CuSO4·5H2O, 0.12 MnCl2·4H2O, 0.06 Na2MoO4·2H2O, 0.12 ZnSO4·7H2O, 0.15 CoCl2·6H2O, 0.18 KI, 0.15 H3BO3, and 10 EDTA.
2.2 Batch experiments
Sealed conical flask reactors (1.0 L) were used in the batch experiments to investigate the nitrite accumulation mechanism of the acclimated sludge under three different cultivation conditions. The sludge obtained from the three reactors after 120 d of operation was flushed three times with deionized water to remove the residual substrate. The prepared sludge was loaded into the reactors and the concentration of the denitrifying sludge was 1500 ± 10 mg VSS per L. Subsequently, the synthetic wastewater was added to the reactors to maintain the initial NO3−-N concentration of 30 mg L−1 and a C/N ratio of 3.0. Nitrogen gas was then immediately pumped into the reactors to establish an anoxic environment. All reactors were placed on stirring plates in a constant-temperature incubator at 25 ± 0.5 °C. The initial pH was 7.3. All tests were repeated three times.
2.3 Effect of different initial pH value on NO2−-N accumulation
The optimal acclimation condition of the activated sludge to achieve partial denitrification was determined by the batch experiments described in Section 2.2. Subsequently, the effect of different initial pH value on the nitrite accumulation in the denitrification process was investigated at C/N = 3. The pH value in the sewage treatment plant from which the samples were obtained ranged from 6.5 to 8.5. This range was appropriately expanded in this experiment to investigate the accumulation of NO2−-N at initial pH values of 5.5, 6.5, 7.3, 8.5, and 9.5 and to monitor the change in the pH during the reaction process; 1 mol L−1 NaOH and 1 mol L−1 HCl solutions were used to adjust the pH. In the batch experiments, a 1 L beaker was used, the water bath temperature was maintained at 25 °C and a magnetic stirring apparatus was used with a rotational speed of 90 rpm.
2.4 Calculation of nitrate-to-nitrite transformation ratio (NTR)
The NTR was calculated as follows:18
NTR (%) = (NO2 −t −NO−2 initial)/(NO−3 initial − NO−3 t) × 100% |
where NO−2 t and NO−2 initial were the nitrite concentration of the sampling point and the initial. And NO−3 t and NO−3 initial were the nitrate concentration of the sampling point and the initial, respectively.
2.5 Analytical methods
NH4+-N, NO2−-N, NO3−-N, total nitrogen (TN), COD, mixed liquor suspended solids (MLSS) and MLVSS were analyzed according to the standard methods.19 Temperature, DO, and pH values were determined using a WTW/Multi 3420 multiparameter device.
2.6 Microbial community analysis
The samples were collected from the three reactors (R1, R2, R3) on day 109 after the SBRs were operating in a stable manner to characterize the microbial community structure of the different partial-denitrification systems using Illumina high-throughput sequencing analysis. DNA was extracted using the E.Z.N.A™ Mag-Bind Soil DNA Kit (OMEGA) according to the manufacturer's instructions. The extracted DNA samples were stored at – 4 °C. The V3–V4 region of the bacterial 16S rRNA genes was amplified by universal primers, 341F: CCTACGGGNGGCWGCAG, 805R: GACTACHVGGGTATCYAAYCC. The polymerase chain reaction (PCR) system (30 μL) was as follows: 15 μL 2× Taq master Mix, 1 μL Bar-PCR primer F (10 μM), 1 μL primer R (10 μM), 10–20 ng genomic DNA; ddH2O was added to obtain 30 μL of solution. The reaction conditions for the PCR amplification were: 3 min of denaturation at 94 °C, 5 cycles of 30 s at 94 °C, 20 s at 45 °C and 30 s at 65 °C, 20 cycles of 20 s at 94 °C, 20 s at 55 °C and 30 s at 72 °C, and 10 min at 72 °C. Subsequently, the Illumina-bridge PCR compatible primers obtained from the first round were introduced into the second round of PCR products. The mixed components of the PCR (30 μL) were as follows: 15 μL 2× Taq master Mix, 1 μL primer F (10 μM),1 μL primer R (10 μM), 20 ng PCR products; ddH2O was added to obtain 30 μL. The PCR reaction conditions were: 3 min of denaturation at 95 °C, 5 cycles of 20 s at 94 °C, 20 s at 55 °C and 30 s at 72 °C, and 5 min at 72 °C. The amplified DNA was purified and accurately quantified using the Qubit3.0 DNA detection kit. Finally, the Illumina-MiSeq sequencing instrument (Shanghai Sangon Biotech., China) was used for sequencing with a concentration of the PCR products of 20 pmol.
3. Results and discussion
3.1 Effect of operation mode on partial-denitrification performance
Sodium acetate, glucose, methyl alcohol, and citrate are commonly used as carbon sources for denitrification. However, Ge et al.20 stated that the denitrification efficiency using glucose as a carbon source was lower than that of other ordinary carbon sources due to the complex metabolic pathways of glucose. The reduction of nitrite was also relatively low over time, thus resulting in relatively high nitrate accumulation. For this reason, glucose was chosen as the carbon source in this experiment.
The denitrification pattern of the activated acclimated sludge in R1 are shown in Fig. 1. From 0–2.5 h, the reduction rates of NO3−-N and NO2−-N were 6.03 ± 0.89 mg (L h)−1 and 2.72 ± 0.31 mg (L h)−1, respectively, whereas the reduction rate of NO3−-N and NO2−-N decreased to 0.52 ± 0.11 mg (L h)−1 and 0.98 ± 0.04 mg (L h)−1 at 2.5–8 h. Due to a sufficient carbon source in the early stage of the reaction, the synthesis rate of nitrate reductase was far higher than that of nitrite reductase, which resulted in a much higher reduction rate of nitrate than of nitrite and a significant accumulation of NO2−-N. The large consumption of carbon resulted in an insufficient carbon source in the later stage. The activity of nitrate reductase was affected by the carbon concentrations to a greater degree than the activity of nitrite reductase. In the later reaction stage, the activity of nitrite reductase was higher than that of nitrate reductase, i.e., the reaction rate of nitrite was higher than that of nitrate, a fact that resulted in a lower accumulation of NO2−-N. This result was in agreement with many previous research findings. Even under low C/N conditions (C/N = 1–3), the nitrite still accumulated at first and then reduced in the denitrification process.
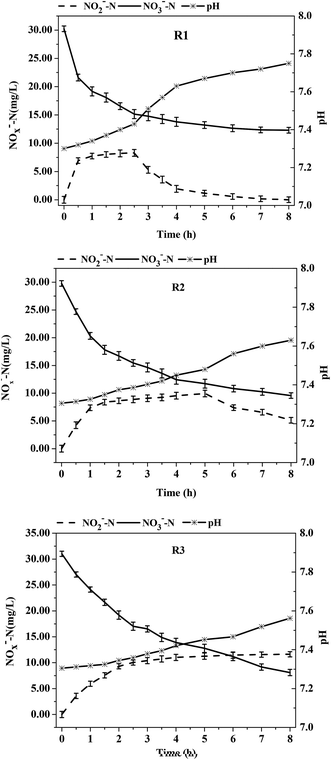 |
| Fig. 1 The variations of NO3−-N, NO2−-N and pH with time using different acclimating modes during denitrification. The data are the means for three independent experiments and presented as the means ± SE. | |
There were distinctive differences in the nitrite accumulation patterns of the acclimated sludge between reactors R2 or R3 (which contained DO due to aeration) and reactor R1 (without DO). As shown in Fig. 1, at C/N = 3, a certain amount of nitrite had accumulated in the three acclimated sludges during the denitrification process. The nitrite accumulation in reactor R1 reached the maximum value of 8.29 ± 1.01 mg L−1 at t = 2.5 h. Subsequently, the value declined and finally reached zero at t = 8 h. It took 5 h for the nitrite accumulation in the R2 sludge to reach the maximum value of 9.94 ± 0.24 mg L−1. However, the nitrite accumulation in the R3 sludge continuously increased and reached the highest value of 11.63 ± 0.77 mg L−1 at t = 8 h. The results indicated that the nitrite accumulation was highest in the R3 reactor and required the longest time. Differences in the reduction rates of NO3−-N and NO2−-N of the denitrifying sludge were also observed between the three acclimation conditions. At t = 0–0.5 h when the initial carbon source concentrations were the same and sufficient, the reduction rates of NO3−-N were R1 [17.24 ± 1.22 mg (L h)−1] > R2 [10.16 ± 0.91 mg (L h)−1] > R3 [7.98 ± 0.47 mg (L h)−1] and the reduction rates of NO2−-N were R1 [3.38 ± 0.79 mg (L h)−1] > R2 [1.72 ± 0.34 mg (L h)−1] > R3 [0.8 ± 0.26 mg (L h)−1]. However, the nitrite accumulation rates were R1 (80.41%) < R2 (83.07%) < R3 (89.97%). In summary, the nitrate/nitrite reduction abilities by the sludge in the R2 and R3 reactors were weaker than that of the denitrifying sludge in the anoxic reactor R1 due to the presence of DO in R2 and R3. However, the nitrite accumulation rates were higher in R2 and R3 than in R1. In addition, the accumulation time of nitrite was longer in R2 and R3, thus better partial-denitrification performance was observed. Research has shown that most denitrifying bacteria are facultative anaerobic bacteria, which are more active under the conditions of low DO or alternating anaerobic–aerobic conditions.21 Although DO to some extent inhibits the activity of denitrifying enzymes, it does not do impair the growth of denitrifying bacteria. In addition, the relative synthesis rate of nitrate/nitrite reductase by the sludge in the reactor was changed due to long-term acclimation at a low C/N ratio and the presence of DO. Nitrite reductase is more sensitive to DO than nitrate reductase.22,23 The synthesis rate of nitrite reductase was much lower than that of nitrate due to the acclimation in the presence of DO. Gayle et al.16 demonstrated that the activity of nitrite reductase was strongly inhibited by molecular oxygen and the accumulation of NO2−-N occurred in the presence of oxygen. In a study on the adaptive mechanisms of nitrate/nitrite reductase in Micrococcus denitrificans and the electron conversion pathway of NO3−-N and O2, Lam et al.24 found that nitrate reductase was not influenced by the presence of O2 but the activity of nitrite reductase was strongly inhibited.
After a period of reaction, the average reduction rates of NO3−-N were R1 = 1.24 ± 0.27 mg (L h)−1, R2 = 2.05 ± 0.09 mg (L h)−1, and R3 = 2.52 ± 0.56 mg (L h)−1 at t = 0.5–8 h when the major factor affecting the nitrate reduction was no longer the acclimatized bacterial community, but the carbon source concentration. Since the degradation rate of the carbon source was directly proportional to that of nitrogen in denitrification and the order of denitrification rate was R1 > R2 > R3 in the early stage of reaction, the order of carbon source concentration was R1 < R2 < R3 after 0.5 h due to the consumption by the sludge; as a result, the order of the average reduction rate of NO3−-N was R1 < R2 < R3 after 0.5 h. This indicated that a higher carbon source concentration resulted in a higher reduction rate of nitrate. Therefore, if the concentration of the carbon source remained unchanged or within a small range, it will further prolong the accumulation time of NO2−-N to obtain steady accumulation. In her research on the influence of addition times of a carbon source on denitrification, Wang et al.25 found that the nitrite accumulation in the denitrification process occurred under one time, four times and eight times of carbon source addition. However, a steady accumulation of nitrites was obtained for a separate addition. The separate additions of a carbon source weakened the inhibitory effect caused by the organic matter on the ANAMMOX bacteria and continuously offered nutrition for the heterotrophic denitrifying bacteria. These findings provide a theoretical basis for achieving partial denitrification coupled with ANAMMOX.
3.2 The microbial diversity of the three different acclimated sludges
3.2.1 Analysis of species diversity and community structure. To explore the accumulation mechanism of nitrite by the partial-denitrification sludge using different acclimatized modes and assess the changes in the microbial community, Illumina MiSeq high-throughput sequencing was used to analyze the microbial community structure during the stable phase of the R1, R2, and R3 reactors; 63
567, 51
051, and 64
600 pieces of high-quality sequence data were obtained from the sludge samples in R1, R2, and R3. The species abundance and diversity of the three samples are presented in Table 2; the operational taxonomic units (OTUs) of R1, R2, and R3 were 5550, 3637, and 5128, respectively. The coverage value of all samples exceeded 90%, demonstrating that the sample had a relatively high library coverage, which indicated the authenticity of the sequencing. The abundance-based coverage estimator (ACE) and the Chao index of sample R1 were higher than those of R2 and R3, indicating that R1 had higher species abundance than R2 and R3. The Shannon index is another index used to estimate the microbial diversity in a sample. The higher value testifies to the higher community diversity. The data in Table 2 showed that R1 possessed higher microbial diversity than R2 and R3. In other words, R2 and R3 had greater microbial specificity, indicating that the acclimation mode of reactors R2 and R3 thoroughly elutriated the microorganisms, and strengthened the dominant position of the functional bacteria. In addition, the Venn diagram showed that the OTU numbers of the three samples accounted for only 4.74% of the total OTU numbers, which demonstrated that the microbial communities of the partial-denitrification sludge varied significantly under different acclimation modes.
Table 2 The microbial community richness and diversity indices
Samples |
OTU |
ACE |
Chao |
Shannon |
Coverage |
R1 |
5550 |
62 399.82 |
29 872.44 |
5.71 |
0.94 |
R2 |
3637 |
38 643.62 |
19 047.06 |
4.54 |
0.95 |
R3 |
5128 |
59 391.09 |
27 037.6 |
4.82 |
0.94 |
24, 22, and 21 well-known bacterial phyla were detected. These three samples had similar dominant bacterial phyla, including Proteobacteria, Planctomycetes, Bacteroidetes, Acidobacteria, Candidatus saccharibacteria, and Chloroflexi, whose relative abundance accounted for over 90%. The Proteobacteria accounted for 44.57%, 14.91%, and 25.09% respectively in the reactors R1, R2, and R3; the proportion of the Proteobacteria in the R1 sample was in agreement with the reported abundance of this phylum in activated sludge.26 A statistical evaluation of the change in the dominant genera (Fig. 4) revealed the distinct bacterial structure in the three different acclimating modes, indicating that the cultivating mode significantly influenced the microorganism composition. The relative abundance of Candidatus saccharibacteria was significantly higher in R2 (45.44%) and R3 (34.96%) than in R1 (5.35%) (p < 0.001). However, the relative abundance of Planctomycetes was significantly lower in R2 (2.02%) and R3 (4.47%) than in R1 (13.71%) (p < 0.001) due to microbial competition. Additionally, the abundance values of Bacteroidetes in the three samples were 13.13%, 14.37%, and 19.14%, respectively, those of the Acidobacteria were 10.12%, 12.94%, and 7.18%, and those of the Chloroflexi were 4.00%, 2.97%, and 2.59%. The members of the denitrifying bacterial genus commonly found in sewage treatment, such as Pseudomonas, Paracoccus, and Comamonas, all belonged to the Proteobacteria phylum. Chloroflexi, containing green hormone, are facultative anaerobes that decompose saccharides and participate in nitrogen removal by denitrification. Bacteroidetes not only metabolize saccharides and protein but also participate in denitrification under anoxic conditions.27 The different acclimation modes gave rise to the significant change in the abundance of Candidatus saccharibacteria in the three samples. However, the abundance was only 5.35% in sample R1. Kindaichi et al.28 tested the abundance of Saccharibacteria in nine activated sludge samples using fluorescence in situ hybridization (FISH) and found that the proportion of these bacteria in different types of activated sludge ranged from 1.3% to 7.3%. However, in the acclimation mode of R2 (intermittent aeration, DO = 2 mg L−1) and R3 (DO = 0.1–0.2 mg L−1), this bacterial phylum had significantly higher abundance than in R1 with values of 45.44% and 34.96%, respectively. The reason was that the introduction of DO enhanced the growth of Candidatus saccharibacteria, which is more adaptable in aerobic environments.
3.2.2 Functional bacterial diversity in different partial-denitrification sludges. The results of the Illumina high-throughput sequencing analysis indicated the dominance of the candidate phylum Saccharibacteria under the three different operating modes (Fig. 2 and 3; Table 3). Saccharibacteria genera incertae sedis represented approximately 5.35% of the total reads in R1 (without aeration), whereas the relative abundance in R2 and R3 was 45.44% and 34.96%, respectively. The lack of DO was the reason for the lower abundance of the Saccharibacteria in the R1 reactor (5.35%) than in R2 and R3 (45.44%, 34.96%). The results also indicated that the genus Saccharibacteria preferred a low DO environment or aerobic environment. Unfortunately, to date, information on the ecological role of Saccharibacteria in natural or artificial ecosystems is still limited. In spite of the fact that the characteristics of Saccharibacteria in sewage treatment are rarely reported, an increasing number of studies have shown that Candidatus saccharibacteria can become the dominant bacterial phylum in many different sewage treatment technologies, such as an SBR used for treating chlorophenol wastewater29 and a microbial fuel cell using the freezing microtome method.30 Additionally, Gu et al.31 found that a high C/N ratio was beneficial for the enrichment of Saccharibacteria in a sequencing biofilm batch reactor treating livestock and poultry wastewater. Ma et al.32 suggested that the phylum Candidatus saccharibacteria preferred to degrade bio-refractory contaminants and utilized complex organic compounds. To our knowledge, this is the first time of Saccharibacteria genera incertae sedis being enriched in partial-denitrification sludge in the presence of DO. Kindaichi et al.28 pointed out that Saccharibacteria can utilize organic matter and saccharides and participate in nitrate reduction under anoxic conditions, thus proving its nitrogen removal ability by denitrification. At the genus level, the dominance of Saccharibacteria genera incertae sedis in the domesticated sludge with aeration demonstrates its role as a keystone species in achieving the transformation of nitrate to nitrite in a partial-denitrification reactor. Notably, when analyzing the community characteristics of partial denitrification coupled with the ANAMMOX process using acetate and alcohol as carbon sources, respectively, Du et al.6 found that Thauera, a denitrifying bacterial genus, accounted for 61.63% and 45.17%, respectively and was the dominant bacterial genus in the partial-denitrification process. However, in our research, the relative abundance of the Thauera genus in R2 and R3 was only 0.5% and 1.28% respectively. The Saccharibacteria had replaced the Thauera genus to become the dominant bacterial genus (Fig. 3), which might have been caused by the different initial seeding sludge samples and carbon sources used in the study. Moreover, some scholars noticed in their study of the Saccharibacteria genus in activated sludge that these bacteria did not consume acetate and ethyl alcohol.28 As shown in Table 3, some other genera reported as denitrifying bacteria in a previous study were detected in the activated sludge samples in R1, R2, and R3. The major denitrifying bacterial genera in this study included Aridibacter, Pseudomonas, Thauera, Gemmobacter, Citrobacter, and Saccharibacteria; Aridibacter was isolated from African soil samples. It was found that in addition to utilizing amino acids, polysaccharides, and organic acids, Aridibacter also participated in denitrification using nitrates as electron acceptors under anoxic conditions. Pseudomonas, which are a common denitrifying bacterial genus for sewage treatment, comprised 1.26% and 0.78% in R1 and R2, respectively. Gemmobacter has also been shown to have denitrification functions. Lv et al.33 separated 116 denitrifying bacterial genera from sediments, biofilms, and water samples from a river in Beijing, including Pseudomonas, Gemmobacter, and Rheinheimera. Li et al.34 found that the Citrobacter freundii strain PXL1 removed arsenic in underground water using nitrates as electron acceptors.
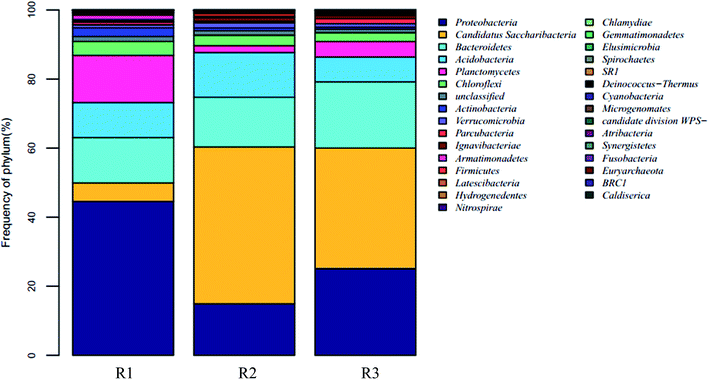 |
| Fig. 2 The comparison of microbial community structure in different partial denitrification sample at phylum level. | |
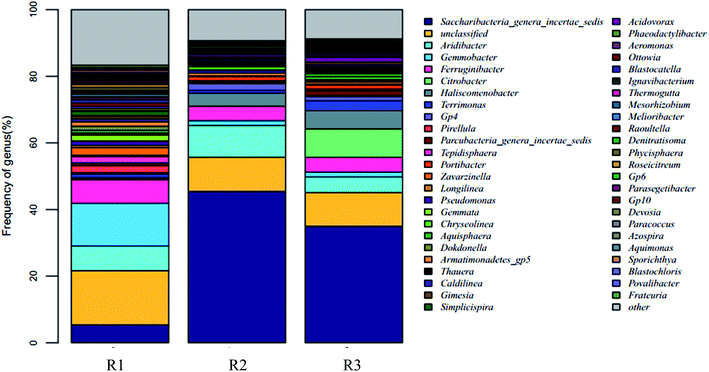 |
| Fig. 3 The comparison of microbial community structure in different partial denitrification sample at genus level. | |
Table 3 The relative abundance of denitrifying bacteria at the genus levelsa
Strains |
The relativeabundance (%) |
R1 |
R2 |
R3 |
Only the bacteria with the relative abundance of >0.1% are listed. |
Saccharibacteria (genera incertae sedis) |
5.35 |
45.44 |
34.96 |
Aridibacter |
7.39 |
9.49 |
4.67 |
Pseudomonas |
1.26 |
0.78 |
— |
Thauera |
— |
0.5 |
1.28 |
Gemmobacter |
12.87 |
1.44 |
1.46 |
Citrobacter |
— |
— |
8.5 |
3.3 The accumulation pattern of nitrite for different initial pH values
The influence of different initial pH values on the denitrification process of the acclimatized sludge in reactor R3 at C/N = 3 was investigated. The initial pH had a significant influence on the accumulation of NO2−-N in the denitrification process in R3. The accumulation mechanism of NO2−-N consisted of an initial increase followed by a decrease under acid conditions, whereas the accumulation of NO2−-N increased continuously under alkaline conditions. As shown in Fig. 5, at an initial pH of 5.5, the NO2−-N concentration reached a maximum of 10.00 ± 2.12 mg L−1 at t = 3 h and then gradually decreased. The NO2−-N concentration fell to 3.13 ± 0.81 mg L−1 after 10 h of reaction; at an initial pH of 6.5, the NO2−-N concentration reached a maximum of 12.65 ± 2.67 mg L−1 at t = 4 h and then decreased to 4.40 ± 1.05 mg L−1 after 10 h of reaction time. This result demonstrated that a lower initial pH value resulted in an earlier peak value of nitrite concentrations, a lower maximum concentration value, and lower overall NO2−-N concentration at the end of the reaction. Under alkalescent/alkaline conditions, when the initial pH was 7.3, 8.5, and 9.5, the NO2−-N concentrations reached 11.65 ± 2.01 mg L−1, 14.01 ± 1.11 mg L−1, and 18.72 ± 0.96 mg L−1, respectively, after 10 h reaction time and the accumulation rate of NO2−-N was 50.8%, 52.5%, and 55.4%; these values were much higher than the results reported in the published study. Cao et al.35 reported that a pilot-scale SBR was used to obtain a stable accumulation of NO2−-N from denitrification using ANAMMOX. Batch tests were conducted to study the effect of the C/N ratio on the production of nitrite and the accumulation of NO2−-N was around 25% using methyl alcohol as a carbon source at C/N ratios of 2.4–3.2 and initial pH values of 7.5–8.5. The results of their study clearly showed that a higher nitrite accumulation rate (≥50%) was easily obtained at C/N = 3 under alkalescent/alkaline conditions. Notably, a higher initial pH resulted in a higher accumulation rate of NO2−-N and was conducive to partial denitrification. In a study on the effect of the initial pH on nitrite accumulation of denitrification at C/N = 3 under low-temperature conditions, Li et al.36 found that the nitrite accumulation was relatively low at initial pH values of 6–7 but much higher when the initial pH values were 7–9; these results are consistent with the research results of our experiment.
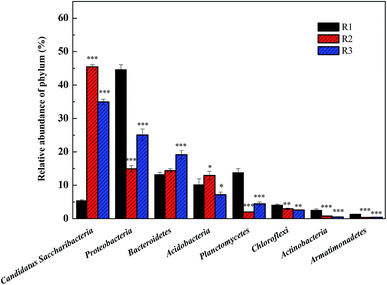 |
| Fig. 4 The relative abundance change of dominant bacterial phylum under three different acclimating modes. The date were presented as mean ± standard deviation. Significant differences compared to group R1 are indicated as follows: *P < 0.05, **P < 0.01 and ***P < 0.001. | |
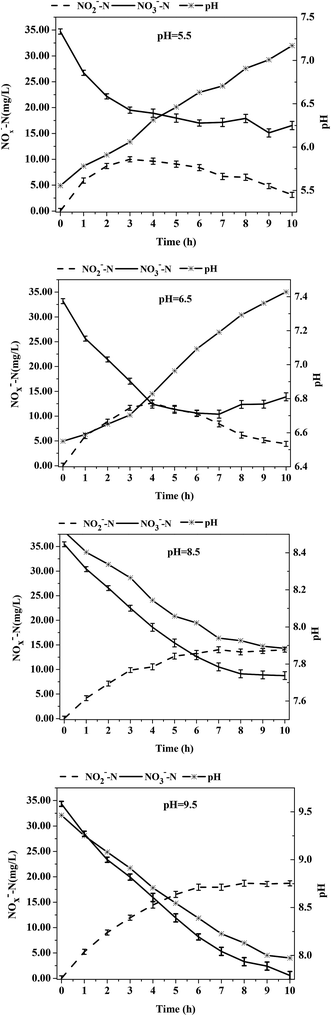 |
| Fig. 5 The variations of NO3−-N, NO2−-N and pH with time under different initial pH condition during denitrification. The data are the means for three independent experiments and presented as the means ± SE. | |
As shown in Table 4, the reduction rate of nitrates and the accumulation rate of nitrites was least affected by the pH value at t = 0–3 h for five initial pH values tested in the experiment. However, differences were observed after t = 3 h. Under acid conditions with an initial pH value of 5.5, the NO3−-N concentration remained unchanged after t = 3 h in the denitrification process. At the initial pH value of 6.5, the reduction rate of NO3−-N also decreased after t = 3 h in the denitrification process. The nitrate concentration did no longer decrease after t = 5 h and the average reduction rate was only 2.85 ± 0.19 mg (L h)−1. The nitrate-nitrogen concentration under these two conditions even rebounded in the later stage. Under alkalescent/alkaline conditions at initial pH values of 7.3, 8.5, and 9.5, the nitrate-nitrogen concentration decreased with varying reduction rates of 1.21 ± 0.11 mg (L h)−1, 1.96 ± 0.89 mg (L h)−1, and 2.77 ± 0.27 mg (L h)−1 at t = 3–10 h, which demonstrated that a higher initial pH value resulted in a higher reduction rate of nitrate in this stage. However, the reduction rate of nitrate nitrogen was not directly affected by the pH. The reduction of 1 g NO3−-N to N2 theoretically requires 2.86 g BOD5 but the reduction of 1 g NO3−-N to NO2−-N requires only 1.14 g 5 day biological oxygen demand (BOD5). Since a higher initial pH value results in a higher accumulation rate of NO2−-N, less NO3−-N is directly reduced to N2 and less carbon is consumed. As a result, the residual concentration of the carbon source is higher, which contributes to the higher reduction rate of NO3−-N. One reason for the higher NO2−-N accumulation rate as a result of the higher initial pH value is that nitrite reductase is more sensitive to a high pH value than nitrate reductase. Another reason is that the production of alkalinity occurs during denitrification when NO2−-N is reduced to N2. The higher OH− concentration at a higher pH value slows down the process of reducing NO2−-N to N2.37 At higher pH values, more nitrite accumulation occurred in this study, which is in agreement with the research results of Glass et al.37
Table 4 The characteristic parameters under different initial pH during partial denitrification
pH |
NO3−-N reduction rate [mg (L h)−1] |
0–3 h |
3–10 h |
5.5 |
5.07 ± 0.12 |
0.42 ± 0.51 |
6.5 |
5.38 ± 0.78 |
0.45 ± 0.62 |
7.3 |
4.83 ± 0.29 |
1.21 ± 0.11 |
8.5 |
4.34 ± 0.04 |
1.96 ± 0.89 |
9.5 |
4.8 ± 0.71 |
2.77 ± 0.27 |
4. Conclusions
Due to the different inhibitory effect of DO on nitrate reductase and nitrite reductase, partial denitrification was more prevalent in the acclimatized R2 and R3 sludge, which contained DO due to aeration, than in R1. The nitrite concentration in the R3 acclimatized reactor increased steadily until the end of the reaction, thus ensuring more complete partial denitrification. The high-throughput sequencing results indicated that Candidatus saccharibacteria was the dominant bacterial genus in R2 and R3; this is the first report of Candidatus saccharibacteria as the dominant bacterial genus in denitrifying sludge. These bacteria prefer aerobic environments, can utilize glucose under anaerobic/anoxic/aerobic conditions, and reduce nitrate under anoxic conditions. It accounted for only 5.35% in the anoxic reactor R1 but reached 34.96% in reactor R3 under low DO conditions and 45.44% in the aerobic reactor R2 with intermittent aeration. The initial pH value had a significant influence on the nitrite accumulation rate in the acclimatized sludge in R3. Under initial acid conditions, the nitrite was continuously degraded but the accumulation rate decreased after reaching the maximum, which was unfavorable for denitrification. Under alkalescent/alkaline conditions, the nitrite accumulation increased continuously during the denitrification process. A higher initial pH value resulted in a higher accumulation rate of nitrite-nitrogen, which was favorable for complete partial denitrification.
Conflicts of interest
There are no conflicts of interest to declare.
Acknowledgements
This research was supported by the National Major Project [2017ZX07103] and Natural Science Foundation of Beijing Municipality [8172012].
References
- S. Cao, R. Du, Y. Peng, B. Li and S. Wang, Chem. Eng. J., 2019, 362, 107–115 CrossRef CAS.
- R. Du, S. Cao, B. Li, H. Zhang, S. Wang and Y. Peng, Bioresour. Technol., 2019, 274, 386–394 CrossRef CAS.
- R. Du, S. Cao, Y. Peng, H. Zhang and S. Wang, Environ. Int., 2019, 126, 707–716 CrossRef CAS PubMed.
- W. Wang, X. Wang, S. Wang and J. Li, RSC Adv., 2018, 8, 32016–32021 RSC.
- S. Kalyuzhnyi, M. Gladchenko, A. Mulder and B. Versprille, Water Res., 2006, 40, 3637–3645 CrossRef CAS PubMed.
- R. Du, S. Cao, B. Li, M. Niu, S. Wang and Y. Peng, Water Res., 2017, 108, 46–56 CrossRef CAS PubMed.
- J. S. Almeida, M. A. M. Reis and M. J. T. Carrondo, Biotechnol. Bioeng., 1995, 46, 476–484 CrossRef CAS PubMed.
- M. Blaszczyk, Appl. Environ. Microbiol., 1993, 59, 3951–3953 CAS.
- H. Sun, Q. Yang, Y. Peng, X. Shi, S. Wang and S. Zhang, Chin. J. Chem. Eng., 2009, 17, 1027–1031 CrossRef CAS.
- T. Le, B. Peng, C. Su, A. Massoudieh, A. Torrents, A. Al-Omari, S. Wett, K. Chandran, C. DeBarbadillo, C. Bott and H. Clippeleir, Water Environ. Res., 2019, 91, 185–197 CrossRef CAS PubMed.
- W. Qian, B. Ma, X. Li, Q. Zhang and Y. Peng, Bioresour. Technol., 2019, 278, 444–449 CrossRef CAS PubMed.
- X. Cao, D. Qian and X. Meng, Environ. Technol., 2013, 34, 45–51 CrossRef CAS PubMed.
- Y. Chen, H. He, H. Liu, H. Li, G. Zeng, X. Xia and C. Yang, Bioresour. Technol., 2018, 249, 890–899 CrossRef CAS PubMed.
- W. Li, H. Li, Y. Liu, P. Zheng and J. Shapleigh, Environ. Sci. Technol., 2018, 52, 10665–10672 CrossRef CAS PubMed.
- J. Ji, Y. Peng, B. Wang, W. Mai, X. Li, Q. Zhang and S. Wang, Chemosphere, 2018, 209, 53–60 CrossRef CAS PubMed.
- B. P. Gayle, G. D. Boardman, J. H. Sherrard and R. E. Benoit, J. Environ. Eng., 1989, 115, 930–943 CrossRef CAS.
- M. S. Coyne and J. M. Tiedje, FEMS Microbiol. Lett., 1990, 73, 263–270 CrossRef CAS.
- S. Cao, S. Wang, Y. Peng, C. Wu, R. Du, L. Gong and B. Ma, Bioresour. Technol., 2013, 149, 570–574 CrossRef CAS PubMed.
- D. E. Andrew and H. F. M. Ann, Standard Methods for the Examination of Water and Wastewater, APHA (American Public Health Association), AWWA (American Water Works Association), WEF (Water Environment Federation), 21st edn, 2005 Search PubMed.
- S. Ge, Y. Peng, S. Wang, C. Lu, X. Cao and Y. Zhu, Bioresour. Technol., 2012, 114, 137–143 CrossRef CAS PubMed.
- P. Li, Q. Li, P. Zhu, J. Wu and S. Liang, J. Dalian Univ. Technol., 2009, 49, 180–186 Search PubMed.
- V. Lazarova, B. Capdeville and L. Nikolov, Water Res., 1994, 28, 1189–1197 CrossRef CAS.
- J. M. Bollag and E. J. Kurek, Appl. Environ. Microbiol., 1980, 39, 845–849 CAS.
- Y. Lam and D. J. D. Nicholas, Biochim. Biophys. Acta, Bioenerg., 1969, 172, 450–461 CrossRef CAS.
- S. Wang, S. Cao, R. Du, C. Wu and Y. Peng, J. Beijing Univ. Technol., 2014, 40, 743–750 CAS.
- T. Zhang, M. F. Shao and L. Ye, ISME J., 2012, 6, 1137–1147 CrossRef CAS PubMed.
- L. Li, Y. Dong, G. Qian, X. Hu and L. Ye, Bioresour. Technol., 2018, 258, 168–176 CrossRef CAS PubMed.
- T. Kindaichi, S. Yamaoka, R. Uehara, N. Ozaki, A. Ohashi, M. Albertsen, P. Nielsen and J. L. Nielsen, FEMS Microbiol. Ecol., 2016, 92, 1–12 CrossRef PubMed.
- J. Zhao, Y. Li, X. Chen and Y. Li, Bioresour. Technol., 2018, 255, 22–28 CrossRef CAS PubMed.
- X. Li, Y. Lu, H. Luo, G. Liu and R. Zhang, Bioresour. Technol., 2017, 241, 384–390 CrossRef CAS PubMed.
- Y. Gu, Y. Wei, Q. Xiang, K. Zhao, X. Yu, X. Zhang, C. Li, Q. Chen, X. Hong and X. Zhang, Sci. Total Environ., 2019, 651, 625–633 CrossRef CAS PubMed.
- X. Ma, X. Wang, Y. Liu, J. Gao and Y. Wang, Ecotoxicol. Environ. Saf., 2017, 138, 163–169 CrossRef CAS PubMed.
- P. Lv, J. Luo, X. Zhuang, D. Zhang, Z. Huang and Z. Bai, Sci. Rep., 2017, 7, 10032 CrossRef PubMed.
- B. Li, X. Pan, D. Zhang, D. J. Lee, F. A. Al-Misned and M. G. Mortuza, Ecol. Eng., 2015, 77, 196–201 CrossRef.
- X. Cao, K. Fu, D. Qian, Z. Zhu and X. Meng, J. Chem. Ind. Eng., 2010, 61, 2938–2943 CAS.
- S. Li, L. Lu, F. Wang, X. Du and M. Ji, Environ. Chem., 2016, 35, 1657–1662 CAS.
- C. Glass and J. Silverstein, Water Res., 1998, 32, 831–839 CrossRef CAS.
|
This journal is © The Royal Society of Chemistry 2019 |
Click here to see how this site uses Cookies. View our privacy policy here.