DOI:
10.1039/C8RA09830A
(Paper)
RSC Adv., 2019,
9, 3337-3344
Acetylacetone functionalized magnetic carbon microspheres for the highly-efficient adsorption of heavy metal ions from aqueous solutions
Received
29th November 2018
, Accepted 11th January 2019
First published on 24th January 2019
Abstract
Herein, Acac-C@Fe3O4, a magnetic carbon (C@Fe3O4) modified with acetylacetone (Acac), was first prepared and used as a solid-phase adsorbent for adsorbing some heavy metal ions from aqueous solution. The adsorbent was characterized by Fourier transform infrared spectroscopy (FTIR), X-ray diffraction (XRD), scanning electron microscopy (SEM), transmission electron microscopy (TEM), X-ray photoelectron spectroscopy (XPS), vibrating sample magnetometry (VSM) and BET studies. Some parameters affecting the adsorption and desorption processes were studied in Pb2+ solution, including sample pH, contact time, initial concentration, type and connection of the desorption solution. Absorption results showed that removal of Pb2+ was 100% under optimal conditions at an initial concentration of 10.0 mg L−1. The adsorption mechanism conformed well to a pseudo-second order kinetic model. The adsorption capacity of the sorbent also showed promising results with Hg2+, Cr3+, Fe2+, Cd2+, Mn2+, Zn2+, Cu2+ and Pb2+, where maximum adsorption capacities reached 98.0, 151.2, 188.9, 202.2, 286.3, 297.2, 305.1 and 345.3 mg g−1, respectively. The Acac-C@Fe3O4 microsphere material was successfully applied to the adsorption of heavy metal ions in aqueous solution.
Introduction
With the growth of industrial activities, the phenomenon of highly toxic heavy metal ions release to water resources becomes more and more serious.1 Heavy metals are difficult to biodegrade and can be enriched hundreds of times during biological amplification of the food chain before entering the human body. Eventually, they will make proteins and enzymes inactive and also cause chronic poisoning.2 Thus, it becomes even more necessary to establish a facile and reliable method to deal with environmental pollution of heavy metal ions. Over decades, several technologies have been developed to remove heavy metal ions from wastewaters such as chemical precipitation (including hydroxide precipitation,3 sulphide precipitation4 and chelating precipitation5), ion exchange,6 membrane filtration (including ultrafiltration,7 reverse osmosis and nano-filtration8 and electrodialysis9), coagulation and flocculation,10 flotation,11 electrochemical treatment12 and adsorption.13 Adsorption methods draw more attention, because they are simple to pursue without additional consumption, high performing and cost-effective.
With all of the absorbing materials, magnetic nanomaterials are different from others as they can be collected by external magnetic fields.14 This performance provides both adsorption and desorption post-processing for convenience. Meanwhile, low-cost nanosized ferric oxides (Fe3O4 or γ-Fe2O3) possess non-toxicity and superparamagnetism and therefore they have gained even more interest.15 Aggregation of these particles can be regulated and controlled by a surface modified process and so far abundant materials have been used as a modified layer including silicon, carbon, synthetic polymers and metals. Among these materials, carbon-based materials are popularly selected because they are easily synthesized and eco-friendly, especially when they have numerous hydrophilic groups and can provide favourable conditions for further modification.16 Wang et al. prepared two different structures of carbon-coated Fe3O4 for lithium storage; both composite materials have good performance and represent a good future for applications. There are also many applications of Fe3O4@C nanomaterials in the environmental treatment fields.17 Liu et al. designed 1D Fe3O4/C/CdS coaxial nanochains for the degradation of RhB.18 Bystrzejewski et al. prepared carbon-encapsulated magnetic nanoparticles to adsorb Cu2+, Co2+, and Cd2+ and their adsorption capacities were 3.21, 1.23 and 1.77 mg g−1, respectively.19 Chen et al. synthesized magnetic core–shell Fe3O4@C nanoparticles with –SO3H/–COOH groups modified for adsorbing Pb2+, Hg2+ and Cd2+ ions and their maximum adsorptions were 90.7, 83.1 and 39.7 mg g−1, respectively.20 The above-mentioned carbon coated magnetic nanomaterials could be used to adsorb heavy metal ions, but their absorption capacities are limited, which may be improved by some new methods.
In order to improve adsorption properties of adsorbents, some chelate molecules, especially multidentate ligands, were selected to modify nanomaterials, such as ethylene diamine tetraacetic acid (EDTA), ethanediamine (EDA) and acetylacetone (Acac), which showed strong coordination with metal ions. Among them, the Acac molecules are superior to other molecules because their active hydrogen and the dicarbonyl structures can be retained during the modification process.21 In this work, the magnetic carbon composite (C@Fe3O4) was synthesized by a hydrothermal method and functionalized with Acac for adsorbing heavy metal ions in an aqueous solution. Behaviours of the adsorption in different analytical conditions and performance under optimized conditions were investigated by FAAS determination. Meanwhile, the adsorption capacities of the as-prepared composites for Cu2+, Zn2+, Mn2+, Cd2+, Fe2+, Hg2+ and Cr3+ were measured and had ideal results. Thus the Acac modified C@Fe3O4 microspheres (Acac-C@Fe3O4) may be used in the metal ion wastewater treatment.
Experimental
Materials
All reagents were of analytical grade including FeCl3·6H2O, Pb(NO3)2·6H2O, FeSO4·7H2O, CuSO4·5H2O, ZnCl2, HgCl2, CrCl3·6H2O, MnSO4, CdCl2·2.5H2O, NaOH, CH3COONa, ethylene glycol (C2H6O2), D(+)-glucose monohydrate (C6H12O6·H2O), ethanediamine (C5H8O2), (3-chloropropyl)triethoxysilane (C9H21ClO3Si, KH230), acetylacetone (Acac), and HCl solution and all were purchased from Sinopharm Chemical Reagent Corporation (Shanghai, China). A target stock solution of Pb2+ with 1000.0 mg L−1 was prepared by dissolving proper amounts of Pb(NO3)2·6H2O and nitric acid in distilled water. Then the solution was further diluted to the given concentrations for testing adsorption capacity and analysing the pre-concentration process.
Instrumentation
The morphology of a sorbent was detected by a field emission scanning electron microscope (SEM, VEGA3 TESCAN, CZ) and a transmission electron microscope (TEM, H800EM Hitachi, JP). Surface area was measured by a BET instrument (TriStar 3020 Micromeritics, CN). XPS spectra were measured by an X-ray photoelectron spectroscope (XPS, Thermo ESCALAB 250XI, CN). A Fourier transform infrared spectrophotometry analysis was performed using a FTIR spectrophotometer (FTIR, Nicolet, USA) equipped with a KBr beam splitter. X-ray diffraction patterns of the samples were recorded by an X-ray powder diffractometer (XRD, D8 advance, DE). Magnetic properties of the sorbent were tested using a vibrating sample magnetometer (VSM, EZ-VSM, MicroSense, USA) at room temperature. Concentrations of metal ions were measured using a flame atomic absorption spectrophotometer with air-acetylene (FAAS, TAS990, China). A digital pH meter was used for pH adjustment of the solutions.
Preparation of Acac-C@Fe3O4
The synthetic process is shown in Scheme 1. First, a solvothermal method was adopted for synthesizing Fe3O4 magnetic composites according to a previously reported procedure.22 In a typical procedure, 36 mmol of FeCl3·6H2O and 200 mmol of CH3COONa were dissolved in 350.0 mL ethylene glycol. The mixture was sonicated for 30 min and then stirred at room temperature for 2 h. Afterword, the homogeneous solution was transferred into a 500 mL Teflon-lined stainless-steel autoclave and reacted at 200 °C for 10 h followed by cooling down to room temperature naturally. The product was washed with ethanol and distilled water for several times, and then dried in a vacuum freeze-drying apparatus for 5 h.
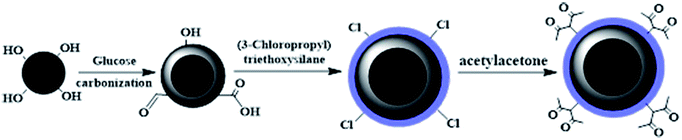 |
| Scheme 1 Diagram of the Acac-C@Fe3O4 magnetic material synthesis process. | |
Secondly, C@Fe3O4 was prepared by a facile hydrothermal method.23,24 Concretely, 0.5 g Fe3O4 nanoparticles were dispersed in 0.1 mol L−1 HNO3 solution and sonicated for 10 min, then were rinsed with distilled water several times until neutral. Subsequently, 8.2 g glucose was dissolved in 80.0 mL distilled water, followed by the addition of 0.5 g Fe3O4 under sonication. The pH value of the mixture was adjusted to 8.0 by a 0.1 mol L–1 NaOH solution. Afterwards, the mixture solution was transferred into a Teflon-lined stainless-steel autoclave (100 mL capacity) and heated at 170 °C for 4 h. The precipitate was washed with distilled water for several times and dried in a vacuum freeze-drying apparatus for 5 h, and then the carbon coated Fe3O4 nanomaterials were obtained.
Thirdly, 1.0 g C@Fe3O4 was dissolved in 100.0 mL water–ethanol under mechanical stirring. Then, 1.0 mL ammonia (25%) and 3.0 mL KH230 were added dropwise to the reaction mixture under constant stirring at 45 °C. After 1 h, the reaction mixture was heated to 60 °C and stirred constantly for 1 h, and then cooled to room temperature. Finally, the magnetic samples (KH230-C@Fe3O4) were isolated by using an external magnet, washed thoroughly with distilled water and ethanol several times, and then dried in a vacuum freeze-drying apparatus.
Finally, 1.0 g NaOH was dissolved in 10.0 mL distilled water and then was added to 40.0 mL ethanol in an ice-water bath, 10.0 mL Acac was added into the mixture, followed by 1.0 g KH230-C@Fe3O4 which was dispersed into the suspension under constant stirring for 0.5 h. Then the mixture was heated to 33 °C for 7 h. The sample was separated using an external magnetic field and washed several times with distilled water and ethanol and dried in a vacuum freeze-drying apparatus. Finally, Acac-C@Fe3O4 composites were prepared successfully.
Adsorption procedure
Aqueous solutions with different Pb2+ concentrations were prepared and adjusted to a given pH value by HCl and NaOH, and then 5.0 mg sorbent was dispersed into the above solution (25.0 mL). The time dependence of the adsorption capacity was also examined as a function of contact time from 1 to 8 min at room temperature using 25.0 mL of Pb2+ solution (20.0 mg g−1) mixed with 5.0 mg of sorbent. The Pb2+ concentration of a sample was measured by FAAS. The adsorption capacity of the material for Cu2+, Zn2+, Mn2+, Cd2+, Fe2+, Hg2+ and Cr3+ were researched under the same conditions. The adsorption process is shown in Fig. 1.
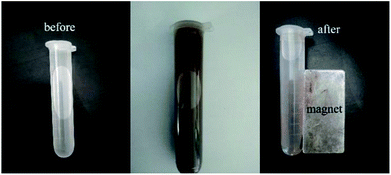 |
| Fig. 1 Schematic diagram of the adsorption process. | |
Result and discussion
Characterization of the magnetic microspheres
VSM analysis. Fig. 2a depicts the magnetization hysteresis loops of typical samples including Fe3O4, C@Fe3O4 and Acac-C@Fe3O4 microspheres at room temperature. The curves indicated that all prepared samples had superparamagnetic properties with negligible magnetic remanence and coercivity. Saturation magnetization of samples decreased from 30 to 14 emu g−1 with an increase of coating layer which showed that the obtained samples had enough magnetic response characteristics to meet magnetic separation process.
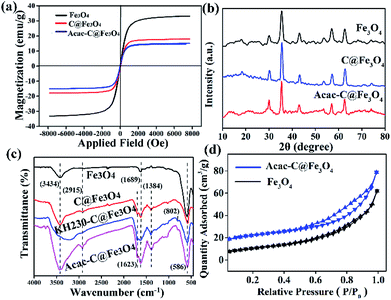 |
| Fig. 2 (a) Magnetization curves of typical prepared samples: Fe3O4, C@Fe3O4 and Acac-C@Fe3O4. (b) XRD patterns of Fe3O4, C@Fe3O4 and Acac-C@Fe3O4. (c) FT-IR spectra of Fe3O4, C@Fe3O4, KH230-C@Fe3O4 and Acac-C@Fe3O4. (d) Nitrogen adsorption–desorption isotherm of Fe3O4 and Acac-C@Fe3O4. | |
Meanwhile, the coercivities of samples were almost stable below 20 Oe. The above suggested the modified layer did not affect the superparamagnetic feature of the composites, but just reduced the saturation magnetization of a sample due to the decreased content of Fe3O4 component in the microspheres.
X-ray diffraction analysis. The XRD patterns of Fe3O4, C@Fe3O4 and Acac-C@Fe3O4 magnetic particles are presented in Fig. 2b. The similar diffraction peaks of all patterns, including 30.1°, 35.5°, 43.0°, 53.6°, 57.0° and 62.6°, indicated the crystal structures of all composites were coincided with a Fe3O4 standard specimen as a cubic spinel structure (JCPDS, no. 19-0629).25 The X-ray diffraction patterns of C@Fe3O4 and Acac-C@Fe3O4 were similar with the pattern of Fe3O4 magnetic particles, which illuminated that they had the same crystal structure and it was not changed during the modification procedures.
FTIR analysis. Composition of the Acac-C@Fe3O4 composite was further characterized by FT-IR spectrophotoscopy, and the relevant results are shown in Fig. 2c. The specific stretching vibration absorption peak of Fe–O in Fe3O4 was obviously visible in four spectra at 586 cm−1, which indicated all samples contained Fe3O4. The peaks at 1623 cm−1 and 1689 cm−1 were attributed to C
C and C
O vibrations and illustrated that carbon was successfully coated on the magnetic core. Meanwhile the absorption peak at 3434 cm−1 implied the existence of residual hydroxyl groups, which confirmed that some hydrophilic groups (–OH) and carbonyl group (–C
O) remained after the process of hydrothermal carbonization treatment.26–28 The peak at 802 cm−1 shown in Fig. 2c indicated the existence of C–Cl groups and suggested that KH230 were grafted on the surface of C@Fe3O4 composites. Curves of Acac-C@Fe3O4, KH230-C@Fe3O4 and Fe3O4 are compared and shown in Fig. 2c and the enhancement of a peak at 1623 cm−1 is attributed to the carbonyl stretching vibration29 while the peak at 2915 cm−1 indicated the existence of –CH3 of Acac. These FTIR data indicated that Acac successfully functionalized the C@Fe3O4 magnetic microspheres.
BET analysis. The ratio surface areas of Fe3O4 and Acac-C@Fe3O4 were analysed by nitrogen adsorption–desorption techniques. As Fig. 2d shows, the nitrogen adsorption–desorption isotherms of Fe3O4 and Acac-C@Fe3O4 have representative type-IV curves. The hysteretic loop in the range of 0.6–0.9 P/P0 is a type of H1 hysteresis. The BET specific surface area of Acac-C@Fe3O4 was measured to be 68.41 m2 g−1, which is higher than that of Fe3O4 (37.41 m2 g−1). This change might be attributed to the porous structure of Acac-C@Fe3O4 coming from carbon coating.
XPS analysis. The surface element composition of Acac-C@Fe3O4 was determined by XPS spectra. As shown in Fig. 3a, the elements of Fe, C, O, and Si were detected on the surfaces of the nanocomposites. The signals of Fe and Si were weaker than that of C, indicating that the Fe3O4 core is coated completely by the carbon shell and a small amount of KH230 was modified. The high-resolution XPS spectrum of Fe 2p can be fitted into two main doublet peaks, as shown in Fig. 3b. In the high-resolution spectrum, it can be seen that the doublet peak at 710.8 eV and 724.2 eV are assigned to Fe 2p3/2 and Fe 2p1/2, which can be attributed to Fe(II) and Fe(III) of the Fe3O4 core. These results were consistent with the XRD analysis. In Fig. 3c, there are more than one chemical state of C in the C 1s region; there peaks were identified and assigned to C–C and C–H (284.8 eV), C–O (286.5 eV), and C
O species (288.4 eV), respectively. These may be ascribed to a carbon shell and acetylacetone.30 The XRD results and previous conclusions were combined and provide supportive evidence for the successful modification of carbon and Acac.
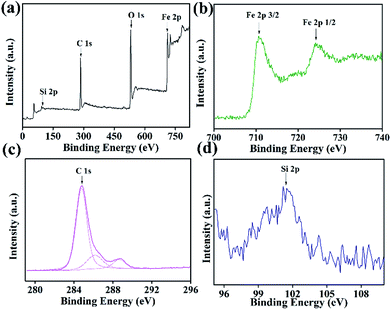 |
| Fig. 3 XPS spectra of Acac-C@Fe3O4. | |
SEM and TEM analysis. Morphology and structures of the Fe3O4, C@Fe3O4 and Acac-C@Fe3O4 microspheres were characterized by scanning electron microscopy and transmission electron microscopy. Fig. 4 indicated the as-prepared Fe3O4 materials were monodispersed microspheres, which were further proved by the relative TEM image of Fe3O4 shown in Fig. 5. The average size of a microsphere is about 260 nm. Fig. 4 also confirmed that the Acac-C@Fe3O4 sample still kept spherical morphology with a broader size distribution. The particle distribution range increased slightly, and the average of size of a microsphere increased to 400 nm. The increase of particle sizes and edges of C@Fe3O4 and Acac-C@Fe3O4 looked like a light shadow layer should be attributed to the carbon coating on the surface of the magnetic core, which was confirmed directly by the TEM image in Fig. 5. This further indicated that the Acac-C@Fe3O4 composite was prepared successfully.
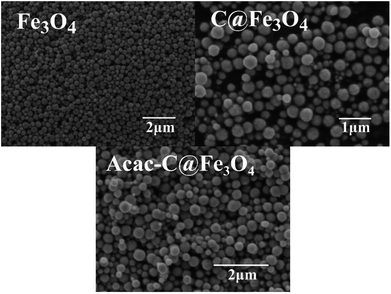 |
| Fig. 4 SEM images of Fe3O4, C@Fe3O4 and Acac-C@Fe3O4. | |
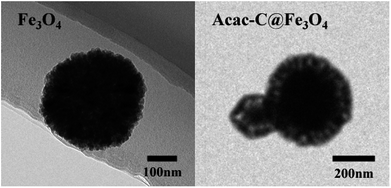 |
| Fig. 5 SEM images of Fe3O4 and Acac-C@Fe3O4. | |
Optimization of pre-concentration conditions
Effect of pH. Acidity of a solution shows strong influence on the adsorption capacity of the adsorbent. To detect influence of the acidity of a solution, Pb2+ was used as the target ion, and the absorbing process was performed at 25 °C for about 20 minutes. The pH of the solution was set in a range from 2.0 and 10.0, and concentrations and amounts of solutions were given at 20 mg L−1 and 25.0 mL in turn with the same amounts of sorbent. The relevant results are shown in Fig. 6a. The curves obviously indicated the absorptivity of C@Fe3O4 was more than Fe3O4 microsphere in a set range and this may be due to the porous structure of the carbon layer where there are rich oxygenic groups on the surface. The absorptivity of Acac-C@Fe3O4 was higher than C@Fe3O4. The absorptivity of Acac-C@Fe3O4 was raised from 25% to 90% with pH values from 2.0 to 6.0 and it slightly declined when the pH changed from 6.0 to 9.0 so the absorptivity was obviously decreased with a pH beyond 9.0. The above indicated that the proper pH range should be controlled between 6.0 and 9.0 for better performance of the adsorbent. Compared with the three curves, the result should be attributed to Acac modified on the surface of C@Fe3O4 composites. Acac has convertible enolyl and dicarbonyl structures with the change in acidity. It is beneficial to resist acidity, which helps to form coordination with lead ions.21 However, the maximum absorptivity of Fe3O4 and C@Fe3O4 microspheres ca. 80% appeared at an acidity around 8.0. Since Pb2+ ions can precipitate under basic conditions, this cannot indicate the as-prepared Fe3O4 microsphere are a suitable lead ion adsorbent although the adsorption capacity is higher in an alkaline setting.
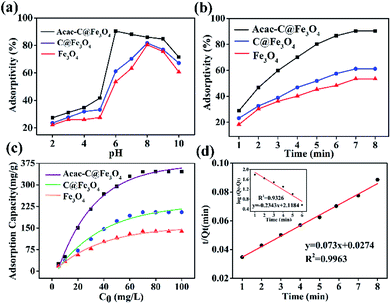 |
| Fig. 6 Effect of pH (a), adsorption time (b), and initial concentration of Pb2+ (c) on the adsorption of Pb2+ onto Acac-C@Fe3O4, C@Fe3O4 and Fe3O4; (d) pseudo-second-order kinetic model for Pb2+ adsorption on Acac-C@Fe3O4 sorbent (inset: pseudo-first-order kinetic model curve). | |
Effect of adsorption time. Adsorption time is an important factor in the application of the as-prepared sorbent. Fig. 6b demonstrates the results about absorptivity versus absorbing time at given conditions including the pH at 6.0, temperature at 25 °C and concentration of Pb2+ at 20 mg L−1. These three curves indicated that the absorptivity increased rapidly within 7 min but after that it remained almost unchanged. The adsorption equilibrium of the absorbing system was reached after around 7 minutes, which suggests the Acac-C@Fe3O4 adsorbent can achieve the maximum adsorption efficiency within 7 minutes in the testing system, and the adsorption rate of lead ions under the conditions investigated was more than 90%. Relatively, the adsorption rate of lead ions was 61% absorbed by the C@Fe3O4 microsphere and only 54% by naked Fe3O4 at same time. The above indicated that the as-prepared Acac-C@Fe3O4 adsorbent had excellent capacity for lead ions, so it could reach the absorption equilibrium quickly. It is beneficial to use the sorbent in the field of separation of heavy metal ions. As the initial concentration of lead ions was raised, the adsorption amount of Acac-C@Fe3O4 sorbent also increased gradually and reached to a maximum value greater than 345.3 mg g−1 with a lead ion concentration at 70 mg L−1, thus basically remaining unchanged. Meanwhile, the adsorption capacity of C@Fe3O4 and Fe3O4 microspheres only reached 205.0 mg g−1 and 138.4 mg g−1, respectively. The maximum adsorption amount of Acac-C@Fe3O4 was obviously higher than C@Fe3O4 and the naked Fe3O4 compared to the three curves. This indicated the saturated adsorption capacity of Acac-C@Fe3O4 can be acquired with a lead ion concentration beyond 70.0 mg L−1. Because all available adsorption sites of as-prepared adsorbent were occupied by ions in a high concentration ion solution, it cannot achieve more amounts of ionic adsorption.31 This suggested the absorption process should belong to chemical absorption.
Adsorption kinetics study. An adsorption isotherm expresses the relationship between adsorption capacity and time equilibrium. The experimental data were obtained by detecting the absorbing amount with a series of given absorbing times with the concentration of Pb2+ ions at 70 mg L−1. The corresponding data were calculated according to two Langmuir isotherm model formula including pseudo-first-order (1) and pseudo-second-order eqn (2):32 |
log(Qe − Qt) = log Qe − k1t/2.303
| (1) |
|
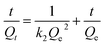 | (2) |
where Qe and Qt refer to the adsorption amount of Pb2+ at equilibrium and time t, respectively, and k1, k2 are the rate constants of pseudo-first-order and pseudo-second-order.According to the model formula, two linear fitting curves and equations are separately shown in Fig. 6d. The analysis results suggested that the pseudo-second-order model (R2 = 0.9915) provided a better description of the Pb2+ adsorption isotherm process than the pseudo-first-order model based on the fitted correlation coefficients (R2) 0.996 for the second order and 0.933 for the first order model. These results further suggested the absorbing process belongs to a chemical process.
Adsorption of other heavy metal ions. Due to Acac having a strong coordination capacity with many metal ions, the sorption capacity of the as-prepared absorbent for various common metal ions (Hg2+, Cr3+, Fe2+, Cd2+, Mn2+, Zn2+ and Cu2+) were investigated in parallel under the same conditions. The concentration of all them was given at 70 mg L−1 with the same optimal conditions as the Pb2+ adsorbing procedures. All data are shown in Fig. 7. This showed that the absorbing capacities of the Acac-C@Fe3O4 sorbent on Hg2+, Cr3+, Fe2+, Cd2+, Mn2+, Zn2+ and Cu2+ were 98.0, 151.2, 189.9, 202.2, 286.3, 297.2 and 305.1 mg g−1, respectively. These results illustrated that the Acac-C@Fe3O4 composites can be applied as a highly efficient sorbent for absorbing some common metal ions, which was beneficial for enriching some metal ions in aqueous solution. In order to measure the quality of the prepared adsorbents, saturated adsorbents were compared with some reported metal ion adsorbents and are shown in Table 1. These data indicated that the as-prepared Acac-C@Fe3O4 adsorbent presented outstanding advantages in adsorption capacity which should be attributed to the functionalization of Fe3O4 microspheres by Acac and carbon.
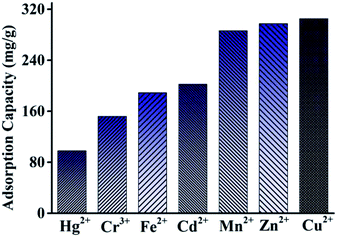 |
| Fig. 7 The adsorption capacity of the as-prepared sorbent for other heavy metal ions. | |
Table 1 Comparison of the adsorption capacities of different adsorbents for the removal of heavy metal ions
Adsorbent |
Adsorption capacity of metal ions (mg g−1) |
Ref. |
Hg2+ |
Cr3+ |
Fe2+ |
Cd2+ |
Mn2+ |
Zn2+ |
Cu2+ |
Pb2+ |
Acac-C@Fe3O4 |
98.0 |
151.2 |
188.9 |
202.2 |
286.3 |
297.2 |
305.1 |
345.3 |
This work |
PEI-magnetic porous |
— |
— |
— |
105.2 |
— |
138.8 |
157.8 |
— |
33 |
Fe3O4@C–SO3H/–COOH |
83.1 |
— |
— |
39.7 |
— |
— |
— |
90.7 |
20 |
Fe3O4@APS@AA-co-CA |
— |
— |
— |
29.6 |
— |
43.4 |
126.9 |
166.1 |
34 |
Magnetic PSSQ hybrid |
— |
— |
— |
5.2 |
13.3 |
— |
30.0 |
66.1 |
35 |
Poly(MMA-co-MA)/Fe3O4 |
— |
90.91 |
— |
— |
— |
111.11 |
109.89 |
— |
36 |
Coir fibers |
— |
— |
11.11 |
— |
— |
— |
9.43 |
24.91 |
37 |
Regeneration of the sorbent
Selection of a desorption solution. Regeneration property and method are important parameters to evaluate an adsorbent. Regeneration of the Acac-C@Fe3O4 composite materials was investigated. First, the type of desorption solution was selected from four kind of common solutions (H2SO4, HNO3 and HCl at 1 mol L−1 and EDTA at 0.5 mol L−1). They were used to desorb Pb2+ from the magnetic sorbent and the desorption efficiencies of them are shown in Fig. 8a. This proved that a HCl solution was the most suitable for use for desorption because it had the highest effective desorption among those tested. On this basis, the influence of the concentration of a HCl solution was further explored and the data are shown in Fig. 8a. With an increase of the HCl concentration desorption efficiency increased as the solution concentration changed from 0.2 to 2.0 mol L−1, and then the desorption efficiency decreased slightly. The above results indicated that desorption solution acidity was an important factor for desorbing lead ion from the as-prepared magnetic sorbent. The coordination sites with Pb2+ were more and more occupied by H+ with increased concentration of the HCl solution, so high acidity was an advantage to regenerate the sorbent. However, when concentration of desorption solution was beyond 2.0 mol L−1, the desorption performance could keep a high level, but the colour of the desorption solution changed to pale yellow, which might be due to Fe3+ ion being generated and suggesting that Acac-C@Fe3O4 materials were damaged in a high acidic setting. So, the concentration of the HCl solution used as a desorption solution should be controlled between 1.0 and 2.0 mol L−1 when desorption efficiency of Acac-C@Fe3O4 sorbent is beyond 95%.
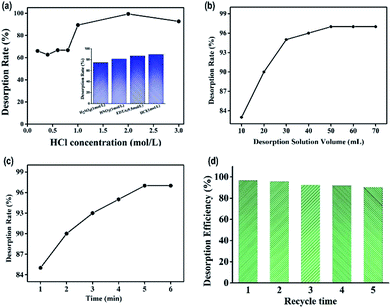 |
| Fig. 8 (a) Influence of the concentration of HCl solution and the kind of desorption solution (inset) on the desorption efficiency; the influence of desorption solution volume (b) and desorption time (c) on the desorption efficiency; (d) desorption and regeneration of the as-prepared sorbent. | |
Meanwhile, the effects of HCl solution volume and desorption time on desorption were also studied. The desorbed efficiency was obviously raised with the increase of HCl solution volume under 50.0 mL as shown in Fig. 8b. There was no change when the volume was more than 50.0 mL. Hence, 50.0 mL desorption solution was used in subsequent research. The desorbed time also affects the desorption process. Fig. 8c showed the recovery of the lead ions was up to 97% when the desorbed time was 5 min. And then, the desorption efficiency kept stable even if time was increased. So we choose 5 min as optimum desorption.
Reuse studies. The reusability of a sorbent is crucial in practical applications. Fig. 8d indicated the results from five time's consecutive adsorption–desorption cycles of Pb2+ on the Acac-C@Fe3O4 microspheres. The desorption efficiency still maintained beyond 90% after 5 times which clearly indicated that absorption capacity just decreased slightly and this decrease of sorption efficiency may be ascribed to loss of adsorbents in a recycled application. The above experimental results suggest our as-prepared Acac-C@Fe3O4 microspheres sorbent possess fine reusability.
Conclusions
An Acac-C@Fe3O4 magnetic adsorbent was successfully prepared by a simple and sustainable procedure and its structure and properties were detected via numerous instrumental measurements. The adsorbent demonstrated outstanding adsorption capacity for some metal ions. Under given conditions, the maximum adsorption capacities for Hg2+, Cr3+, Fe2+, Cd2+, Mn2+, Zn2+, Cu2+ and Pb2+ were 98.0, 151.2, 188.9, 202.2, 286.3, 297.2, 335.1 and 345.3 mg g−1, respectively. The adsorption process reached adsorption equilibrium within 7 min and conforms to a pseudo-second-order kinetics model. The adsorbent had excellent stability and reusability. The Acac-C@Fe3O4 magnetic adsorbent may provide an alternative for applications in the adsorption and separation of heavy metal ions in aqueous solutions. What's more, the material can be used as a substrate and in the further synthesis of metal–organic frameworks.
Conflicts of interest
There are no conflicts to declare.
Acknowledgements
This work was financially supported by the Natural Science Foundation of Shanghai (No. 15ZR1428500) and the National Natural Science Foundation of China (No. 20906061).
Notes and references
- Z. S. Kardar, M. H. Beyki and F. Shemirani, Takovite-aluminosilicate@MnFe2O4 nanocomposite, a novel magnetic sorbent for efficient preconcentration of lead ions in food samples, Food Chem., 2016, 209, 241–247 CrossRef CAS PubMed.
- F. L. Fu and Q. Wang, Removal of heavy metal ions from wastewaters: a review, J. Environ. Manage., 2011, 92(3), 407–418 CrossRef CAS PubMed.
- F. R. Peligro, I. Pavlovic, R. Rojas and C. Barriga, Removal of heavy metals from simulated wastewater by in situ formation of layered double hydroxides, Chem. Eng. J., 2016, 336, 1035–1040 CrossRef.
- H. Wang, F. Chen, S. Mu, D. Zhang, X. Pan, D. J. Lee and J. S. Chang, Removal of antimony (Sb (V)) from Sb mine drainage: biological sulfate reduction and sulfide oxidation–precipitation, Bioresour. Technol., 2013, 146, 799–802 CrossRef CAS PubMed.
- F. Fu, L. Xie, B. Tang, Q. Wang and S. Jiang, Application of a novel strategy—Advanced Fenton-chemical precipitation to the treatment of strong stability chelated heavy metal containing wastewater, Chem. Eng. J., 2012, 189, 283–287 CrossRef.
- S. J. Kim, K. H. Lim, K. H. Joo, M. J. Lee, S. G. Kil and S. Y. Cho, Removal of heavy metal-cyanide complexes by ion exchange, Korean J. Chem. Eng., 2002, 19(6), 1078–1084 CrossRef CAS.
- X. Li, G. M. Zeng, J. H. Huang, C. Zhang, Y. Y. Fang, Y. H. Qu, F. Luo, D. Lin and H. L. Liu, Recovery and reuse of surfactant SDS from a MEUF retentate containing Cd2+ or Zn2+ by ultrafiltration, J. Membr. Sci., 2009, 337(1–2), 92–97 CrossRef CAS.
- C. M. Zhong, Z. L. Xu, X. H. Fang and L. Cheng, Treatment of acid mine drainage (AMD) by ultra-low-pressure reverse osmosis and nanofiltration, Environ. Eng. Sci., 2007, 24(9), 1297–1336 CrossRef CAS.
- K. I. Dermentzis, A. E. Davidis, A. S. Dermentzi and C. D. Chatzichristou, An electrostatic shielding-based coupled electrodialysis/electrodeionization process for removal of cobalt ions from aqueous solutions, Water Sci. Technol., 2010, 62(8), 1947–1953 CrossRef CAS PubMed.
- C. Y. Teh, P. M. Budiman, K. P. Y. Shak and T. Y. Wu, Recent advancement of coagulation–flocculation and its application in wastewater treatment, Ind. Eng. Chem. Res., 2016, 55(16), 4363–4389 CrossRef CAS.
- H. Polat and D. Erdogan, Heavy metal removal from waste waters by ion flotation, J. Hazard. Mater., 2007, 148(1–2), 267–273 CrossRef CAS PubMed.
- T. K. Tran, H. J. Leu, K. F. Chiu and C. Y. Lin, Electrochemical Treatment of Heavy Metal-containing Wastewater with the Removal of COD and Heavy Metal Ions, J. Chin. Chem. Soc., 2017, 64(5), 493–502 CrossRef CAS.
- H. B. Bradl, Adsorption of heavy metal ions on soils and soils constituents, J. Colloid Interface Sci., 2004, 277(1), 1–18 CrossRef CAS PubMed.
- X. Li, S. F. Wang, Y. G. Liu, L. H. Jiang, B. Song, M. F. Li, G. M. Zeng, X. F. Tan, X. X. Cai and Y. Ding, Adsorption of Cu (II), Pb (II), and Cd (II) ions from acidic aqueous solutions by diethylenetriamine- pentaacetic acid-modified magnetic graphene oxide, J. Chem. Eng. Data, 2016, 62(1), 407–416 CrossRef.
- M. Hua, S. J. Zhang, B. C. Pan, W. M. Zhang, L. Lv and Q. X. Zhang, Heavy metal removal from water/wastewater by nanosized metal oxides: a review, J. Hazard. Mater., 2012, 211, 317–331 CrossRef PubMed.
- Y. L. Wang, X. Zhang, L. Gao, Y. Mao, X. Hu and W. D. Lou, One-Pot Magnetic Field Induced Formation of Fe3O4/C Composite Microrods with Enhanced Lithium Storage Capability, Small, 2014, 10(14), 2815–2819 CrossRef CAS PubMed.
- Y. L. Wang, Y. Zhang, Y. Wu, Y. Zhong, X. Hu and W. D. Lou, Carbon-coated Fe3O4 microspheres with a porous multideck-cage structure for highly reversible lithium storage, Chem. Commun., 2015, 51(32), 6921–6924 RSC.
- Y. Liu, L. Zhou, Y. Hu, C. Guo, H. Qian, F. X. Zhang and W. D. Lou, Magnetic-field induced formation of 1D Fe3O4/C/CdS coaxial nanochains as highly efficient and reusable photocatalysts for water treatment, J. Mater. Chem., 2011, 21(45), 18359–18364 RSC.
- M. Bystrzejewski, K. Pyrzyńska, A. Huczko and H. Lange, Carbon-encapsulated magnetic nanoparticles as separable and mobile sorbents of heavy metal ions from aqueous solutions, Carbon, 2009, 47(4), 1201–1204 CrossRef CAS.
- Z. Chen, Z. Geng, Z. Zhang, L. b. Ren, T. T. Tao, R. C. Yang and Z. X. Guo, Synthesis of magnetic Fe3O4@ C nanoparticles modified with –SO3H and –COOH groups for fast removal of Pb2+, Hg2+, and Cd2+ ions, Eur. J. Inorg. Chem., 2014,(20), 3172–3177 CrossRef CAS.
- M. H. Xu, J. Chai, N. T. Hu, D. Huang, Y. X. Wang, X. L. Huang, H. Wei, Z. Yang and Y. F. Zhang, Facile synthesis of soluble functional graphene by reduction of graphene oxide via acetylacetone and its adsorption of heavy metal ions, Nanotechnology, 2014, 25(39), 395602 CrossRef PubMed.
- S. H. Liu, R. M Xing, F. Lu, R. K. Rana and J. J. Zhu, One-pot template-free fabrication of hollow magnetite nanospheres and their application as potential drug carriers, J. Phys. Chem. C, 2009, 113(50), 21042–21047 CrossRef CAS.
- X. Y. Zhang, S. C. Zhu, C. H. Deng and X. M. Zhang, Highly sensitive thrombin detection by matrix assisted laser desorption ionization-time of flight mass spectrometry with aptamer functionalized core–shell Fe3O4@C@Au magnetic microspheres, Talanta, 2012, 88, 295–332 CrossRef CAS PubMed.
- Q. H. Li, X. Z. Ren, L. Z. Tong, X. D. Chen, H. Ding and H. Yang, Deposition of luminescence YBO3: Eu3+, Gd3+ on ferromagnetic Fe@ C nanoparticles, Dyes Pigm., 2014, 107, 161–165 CrossRef CAS.
- X. F. Lu, H. Mao, D. M. Chao, W. J. Zhang and Y. Wei, Ultrasonic synthesis of polyaniline nanotubes containing Fe3O4 nanoparticles, J. Solid State Chem., 2006, 179(8), 2609–2615 CrossRef CAS.
- Z. Y. Zhang and J. L. Kong, Novel magnetic Fe3O4@C nanoparticles as sorbents for removal of organic dyes from aqueous solution, J. Hazard. Mater., 2011, 193, 325–329 CrossRef CAS PubMed.
- J. R. Meng, C. Y. Shi, B. W. Wei, W. J. Yu, C. H. Deng and X. M. Zhang, Preparation of Fe3O4@C@PANI magnetic microspheres for the extraction and analysis of phenolic compounds in water samples by gas chromatography–mass spectrometry, J. Chromatogr. A, 2011, 1218(20), 2841–2847 CrossRef CAS PubMed.
- S. K. Li, F. Z. Huang, Y. Wang, Y. H. Shen, L. G. Qiu, A. J. Xie and S. J. Xu, Magnetic Fe3O4@C@Cu2O composites with bean-like core/shell nanostructures: synthesis, properties and application in recyclable photocatalytic degradation of dye pollutants, J. Mater. Chem., 2011, 21(20), 7459–7466 RSC.
- D. Saberi, S. Mahdudi, S. Cheraghi and A. Heydari, Cu (II)–acetylacetone complex covalently anchored onto magnetic nanoparticles: Synthesis, characterization and catalytic evaluation in amide bond formation via oxidative coupling of carboxylic acids with N, N-dialkylformamides, J. Organomet. Chem., 2014, 772, 222–228 CrossRef.
- C. Guo, W. Lu, G. Wei, L. Jiang, Y. Yu and Y. Hu, Formation of 1D chain-like Fe3O4@C/Pt sandwich nanocomposites and their magnetically recyclable catalytic property, Appl. Surf. Sci., 2018, 457, 1136–1141 CrossRef CAS.
- K. Wang, J. W. Gu and N. Yin, Efficient Removal
of Pb (II) and Cd (II) Using NH2-Functionalized Zr-MOFs via Rapid Microwave-Promoted Synthesis, Ind. Eng. Chem. Res., 2017, 56(7), 1880–1887 CrossRef CAS.
- G. X. Chen, C. D. Qiao, Y. Wang and J. S. Yao, Synthesis of magnetic gelatin and its adsorption property for Cr (VI), Ind. Eng. Chem. Res., 2014, 53(40), 15576–15581 CrossRef CAS.
- Y. Pang, G. Zeng, L. Tang, Y. Zhang, Y. Y. Liu, X. X. Lei, Z. Li, J. C. Zhang and G. X. Xie, PEI-grafted magnetic porous powder for highly effective adsorption of heavy metal ions, Desalination, 2011, 281, 278–284 CrossRef CAS.
- F. Ge, M. M. Li, H. Ye and B. X. Zhao, Effective removal of heavy metal ions Cd2+, Zn2+, Pb2+, Cu2+ from aqueous solution by polymer-modified magnetic nanoparticles, J. Hazard. Mater., 2012, 211, 366–372 CrossRef PubMed.
- S. Nagappan, H. M. Ha, S. S. Park, N. J. Jo and C. S. Ha, One-pot synthesis of multi-functional magnetite–polysilsesquioxane hybrid nanoparticles for the selective Fe3+ and some heavy metal ions adsorption, RSC Adv., 2017, 7(31), 19106–19116 RSC.
- A. Masoumi, M. Ghaemy and A. N. Bakht, Removal of metal ions from water using poly (MMA-co-MA)/modified-Fe3O4 magnetic nanocomposite: isotherm and kinetic study, Ind. Eng. Chem. Res., 2014, 53(19), 8188–8197 CrossRef CAS.
- P. M. Shukla and S. R. Shukla, Biosorption of Cu (II), Pb (II), Ni (II), and Fe (II) on alkali treated coir fibers, Sep. Sci. Technol., 2013, 48(3), 421–428 CrossRef CAS.
|
This journal is © The Royal Society of Chemistry 2019 |
Click here to see how this site uses Cookies. View our privacy policy here.