DOI:
10.1039/C8RA10011J
(Paper)
RSC Adv., 2019,
9, 4824-4831
A new synthetic pathway based on one-pot sequential aza-Michael addition and photoCuAAC click reactions
Received
5th December 2018
, Accepted 29th January 2019
First published on 7th February 2019
Abstract
A solvent-free process is described for the synthesis of tailor-made molecules from a one-pot, two-step approach combining aza-Michael addition and photoinduced copper(I) catalysed azide–alkyne (photo-CuAAC) reactions. After the first reaction between an amine and an acrylate, cycloaddition between an azide and an alkyne is activated by light irradiation in the presence of a copper complex. The kinetics of the aza-Michael addition and photo-CuAAC reaction were investigated by liquid state 1H NMR spectroscopy and real-time Fourier transform infrared spectroscopy. This new process represents a well-defined spatio-temporal pathway to the synthesis of bespoke intermediate molecules for various applications.
Introduction
The concept of click chemistry was first introduced by Sharpless in 2001 to describe several classes of chemical transformations that share a number of important properties, including very high efficiency in terms of both conversion and selectivity under very mild reaction conditions, along with a simple workup.1 Cycloaddition reactions (Huisgen 1,3-dipolar addition,2,3 Diels–Alder reactions4–6), addition to carbon–carbon multiple bonds (thiol–ene/yne reactions and Michael additions),7–10 nucleophilic ring-opening reactions of strained heterocyclic electrophiles (epoxides, aziridines and aziridinium ions),11 and reactions of non-aldolcarbonyl compounds (including ureas, oximes and hydrazones)12–14 meet these criteria.
The most well-known click reaction is the copper catalysed alkyne–azide cycloaddition (CuAAC) that leads to the regioselective formation of 1,4-disubstituted 1,2,3-triazoles. This reaction typically proceeds through the in situ generation of Cu(I) from Cu(II) in the presence of a reducing agent such as sodium ascorbate in an aqueous solvent (water and alcohol). The reaction takes place under mild conditions and gives high yields with little or no byproducts.1,2 However, a large quantity of a catalyst is generally required, the reaction rate is relatively slow, and residual copper is not easily removed after the reaction.15 Nevertheless, CuAAC is widely implemented in organic chemistry,3,16,17 biosciences,18 drug discovery1 and materials science.19 Efforts have been made to accelerate the CuAAC reaction rate, using thermal heating,20 the addition of podand-like ionic liquids21 or ultrasound,22 and such methods have been found to be efficient. Ligands (amines, pyridines, triazoles and N-heterocyclic carbenes) are usually employed to stabilise Cu(I), to avoid side reactions, and to accelerate Cu(I) catalysis.15 As an alternative to thermal promotion of the reaction, a photochemical route to the in situ generation of Cu(I) was reported in 2006.23 In addition, Yagci's24,25 and Bowman's groups26 have suggested a new photochemical pathway based on the in situ formation of Cu(I) from a Cu(II)/L complex, using light to trigger the CuAAC reaction. This photoinduced reaction can proceed either via the direct irradiation of the Cu(II) complex or by indirect photoreduction in the presence of a photoinitiator. In the direct approach, the Cu(I) active species is generated upon UV irradiation, as a result of electron transfer from the π electron molecular orbitals of the ligand–metal complex to the metal ion.27–29 In the indirect pathway, Cu(II) is reduced through a photoinduced electron transfer process with radical species generated via the irradiation of both Norrish Type I and Type II photoinitiators.25,30–33 This so-called photoCuAAC process offers a facile means of controlling the reaction rate by changing the photoinitiator or the light intensity. Thus, the photoCuAAC reaction is a powerful technique that combines the classical benefits of CuAAC and the myriad advantages of a photochemical process.
The Michael addition reaction is a versatile and effective pathway for the coupling of alkenes, involving activation by an electron-withdrawing group with a nucleophile,34 such as a carbanion (as in Michael addition) or a heteroatom centre (hetero-Michael addition), including nitrogen (aza-Michael), sulfur (thiol-Michael), and phosphorus. The Michael addition offers high yields, mild conditions, and favourable reaction rates, and so can be said to fulfil the criteria of a click reaction.1 In particular, amines can act as both nucleophiles and bases and so no additional catalyst is needed in reactions involving such compounds.35,36 Primary amines can react with two equivalents of an acceptor in a two-step process. The initial reaction is with an electron-deficient olefin to form the corresponding mono-adduct (the AZ1 reaction), which subsequently reacts further to give the bis-adduct of a tertiary amine (the AZ2 reaction).37 The compounds obtained via an aza-Michael process are widely used in organic chemistry to synthesise biomolecules, and in polymer chemistry for the design of new functional materials such as hyperbranched polymers and hydrogels.38 As an example, this reaction was recently used to create a shape memory polymer.39
Over the last several years, different synthetic protocols have been proposed based on combinations of the photoCuAAC or Michael addition reactions with photoactivated chain-growth. A process including two-stage sequential thiol-acrylate and photoCuAAC reactions has been described by Alzahrani et al.40 for wrinkle formation. Doran et al.27 developed a one-pot double click sequential photoCuAAC and thiol–ene process for preparing biologically active block copolymers. In addition, the one-pot formation of InterPenetrated Networks (IPNs) by coupling a photoCuAAC reaction and methacrylate polymerisation using a single photoinitiator was described by Shete and co-workers in 2017.41 Maetz et al.42 proposed a one-step methodology based on a combination of photoCuAAC and photosol–gel reactions to obtain hybrid organic–inorganic materials. As well, González et al.43 reported a dual curing method for coupling aza-Michael addition with the photoinduced radical polymerisation of excess acrylate groups.
In the present work, we developed a one-pot double click synthesis by combining photoCuAAC and Michael addition reactions. This strategy requires the judicious selection of the initial molecules so as to properly control the process and improve the efficiency of the chemical reactions. Starting from a model system, the reaction kinetics were initially investigated separately using real-time Fourier transform infrared spectroscopy (RT-FTIR) and liquid state 1H NMR, and key factors controlling both reactions were identified. Subsequently, a one-pot, two-step process was identified by coupling the two click reactions.
Experimental
Materials
Ethyl 2-bromopropionate (99%, Sigma Aldrich) and NaN3 (≥99.5%, Sigma Aldrich) were used to synthesise ethyl 2-azidopropionate according to a procedure in the literature.44 Propargyl alcohol (99%, Alfa Aesar), copper(II) chloride, copper(II) sulfate anhydrous, N,N,N′,N′′,N′′-pentamethyldiethylenetriamine (99%, PMDETA), tris[2-(dimethylamino)ethyl]amine (97%, Me6TREN), 1,5-pentanediamine (≥97%, PDA), diethylamine (≥99.5%, DEA), methyl acrylate (99%) and deuterochloroform (≥99%, CDCl3) were obtained from Sigma-Aldrich, and were used without further purification. Isopropylthioxanthone (ITX, Fluka, a mixture of 2- and 4-isomers) was used as a photoinitiator.
Synthesis of Cu(II) complexes
The Cu(II) complexes were prepared by dissolving 1
:
1 molar ratios of CuCl2 and various amine ligands (such as PMDETA and Me6TREN) in acetonitrile (c = 0.37 mol L−1). The resulting mixtures were stirred for 3–6 h at room temperature to form turquoise solutions that were subsequently concentrated under reduced pressure to obtain Cu(II)/L powders.
General procedure for the preparation of samples for photo-CuAAC reactions
Ethyl 2-azidopropionate (200 mg, 1.38 mmol), propargyl alcohol (77.4 mg, 1.38 mmol), CuCl2/L (8.4 mg, 1.7 mmol%) and ITX (3.9 mg, 1.4 wt% relative to the total mass of azide and alkyne) were mixed in a glass vial. This mixture was stirred for 5 min in the dark to obtain a homogenous formulation.
General procedure for aza-Michael addition
Diethylamine (101 mg, 1.38 mmol) was introduced into a round-bottom flask after which methyl acrylate (119 mg 1.38 mmol) was added. The mixture was magnetically stirred until completion of the reaction verified by liquid state 1H NMR spectroscopy.
General method of implementing the two simultaneous reactions
In the absence of light, ethyl 2-azidopropionate (200 mg, 1.38 mmol), propargyl alcohol (77.4 mg, 1.38 mmol), CuCl2/L (8.4 mg, 1.7 mmol%), ITX (3.9 mg, 1.4 wt% relative to the total mass of azide and alkyne), diethylamine (101 mg, 1.38 mmol) and methyl acrylate (119 mg 1.38 mmol) were transferred into a round-bottom flask equipped with a magnetic stirrer. The mixture was stirred at room temperature until the aza-Michael reaction was complete. The formulation was subsequently laminated between two BaF2 pellets and exposed to UV radiation for 20 min.
Real-time Fourier transform infrared spectroscopy (RT-FTIR)
RT-FTIR spectroscopy is a powerful and simple technique for the analysis of photo-CuAAC and aza-Michael kinetics. During these trials, samples were laminated between two BaF2 pellets and exposed simultaneously to UV radiation and the IR beam of the instrument (Bruker Vertex 70, 4 cm−1 resolution). UV light was generated by a mercury-xenon arc lamp (200 W) in air at room temperature, using a 365 nm reflector and a flexible light guide to provide an incident irradiance of 180 mW cm−2. During irradiation, the evolution of the photo-CuAAC process was monitored by observing the decrease in the IR band corresponding to the vibrational mode of the azide group (2100 cm−1). Similarly, the disappearance of the acrylate C
C double bonds was followed by tracking the peak at 814 cm−1, corresponding to the H–C
C stretching mode. All spectra were baseline corrected prior to integration using the OPUS 7.0 software package. Conversions of azide and acrylate functional groups were then calculated according to the equation
where A0 is the absorbance prior to irradiation and At is the absorbance at time t. All measurements were repeated at least three times and reproducible results were obtained.
Liquid state 1H NMR spectroscopy
NMR was used to identify the photoCuAAC triazole products and to investigate the aza-Michael addition. In each trial, a specimen of approximately 5 mg was dissolved in 0.6 mL CDCl3 then transferred to an NMR tube for 1H NMR analysis (Bruker 300 MHz spectrometer) at 25 °C. All chemical shifts are reported in ppm relative to tetramethylsilane (TMS).
Results and discussion
The photo-CuAAC reaction
The spontaneous CuAAC reaction in the absence of light and at room temperature. In prior work, our group determined that PDA is reactive with acrylates, generating extremely homogeneous polymer networks, when aza-Michael addition is combined with free radical photopolymerisation.37 We therefore attempted to study the reactivity of PDA in the present project as well. To examine the compatibility of the aza-Michael and photoCuAAC reagents, PDA was added to the propargyl alcohol in the presence of the CuCl2/PMDETA complex. Interestingly, a yellowish precipitate was observed to form during the mixing of the compounds. A similar result was obtained when DEA was added to a combination of CuCl2/PMDETA and propargyl alcohol. In both cases, the absorption of the mixture at 665 nm, which is associated with the d → d transition of Cu(II),31 was found to decrease (Fig. 1), and this decrease could be used to monitor the extent of Cu(II) reduction. The reduction of Cu(II) to Cu(I) by terminal alkynes in the presence of tetramethylethylenediamine (TMEDA) has already been reported in the literature, and TMEDA has been shown to act simultaneously as a Cu ligand and a base.45 Similarly, in our system, Cu(II) coordination by C
C bonds activated the C–H bond and facilitated deprotonation by the amine and the formation of the Cu-acetylene solid.
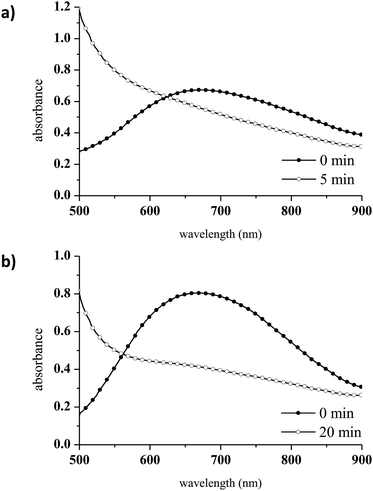 |
| Fig. 1 The UV-vis spectra (in methanol) of CuCl2/PMDETA mixed with propargyl alcohol, together with 1,5-pentanediamine (a) or diethylamine (b). A 1/1 alkyne/amine molar ratio and a 10 mM copper complex concentration were used. | |
To avoid CuAAC activation at room temperature, a different Cu complex was selected. Tris[2-(dimethylamino)ethyl]amine (Me6TREN), a branched tetradentate ligand which has been widely used as an active ligand for Cu(II) in atom transfer radical polymerisation (ATRP), was chosen for this purpose.46 CuCl2/Me6TREN was employed as the Cu(II) complex in the present work because tetradentate ligands stabilise Cu(I) to a greater extent than tridentate ligands such as PMDETA.47 The spectroscopic features of CuCl2/Me6TREN were found to differ from those of the CuCl2/PMDETA complex. This was not unexpected, as the absorption characteristics of Cu complexes vary with changes in structure.48,49 Specifically, the CuCl2/Me6TREN spectrum exhibits two distinct absorption bands at 740 nm (the d → d transition of Cu(II))50 and 929 nm, as shown in Fig. 2.
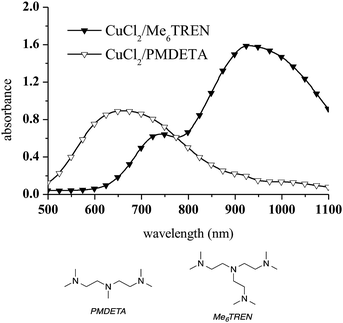 |
| Fig. 2 UV-vis spectra (in methanol) of CuCl2/Me6TREN and CuCl2/PMDETA. A 1/1 CuCl2/ligand molar ratio and a 10 mM copper complex concentration were used. | |
The addition of propargyl alcohol to CuCl2/Me6TREN in the presence of 1,5-pentanediamine induced a dramatic change in the UV-vis spectrum at 740 nm (Fig. 3a). Conversely, diethylamine was not able to reduce Cu(II) to Cu(I) via alkyne deprotonation and formation of a Cu-acetylene solid (Fig. 3b). Therefore, diethylamine was selected as the precursor for the aza-Michael reaction and CuCl2/Me6TREN was used for the first time as a catalyst in a photoCuAAC reaction.
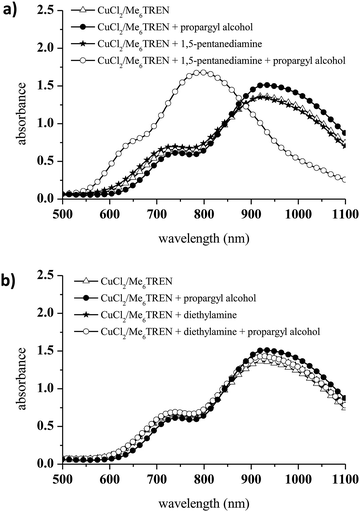 |
| Fig. 3 The UV-vis spectra (in methanol) of 10 mM CuCl2/PMDETA at a 1/1 molar ratio (triangles), with propargyl alcohol at a 1/60 molar ratio (filled circles), with different amines at a 1/60 molar ratio (filled stars), and with different amines and propargyl alcohol at a 1/60/60 molar ratio (circles). Amines: (a) 1,5-pentanediamine; (b) diethylamine. | |
PhotoCuAAC with CuCl2/Me6TREN as the catalyst. Different approaches have been proposed for the photosensitisation of Cu(II) complexes with various ligands to obtain redox reactions upon light exposure.35 Yagci's group demonstrated that the photoreduction of Cu(II) to Cu(I) can be used as a unique activator in ATRP as well as a catalyst for the CuAAC reaction, removing the need for a reducing agent.16To limit degradation of the azide under UV irradiation,24 wavelengths below 300 nm were removed using borosilicate glass. ITX28 was selected for use in these trials due to its ability to photoreduce Cu(II) and its commercial availability. Therefore, Cu(I) was generated by a bimolecular electron transfer to the Cu(II) complex either from the excited photosensitiser or from a photogenerated radical.36,37 It should be noted that other photoinitiators, such as 2,4,6-trimethylbenzoyldiphenylphosphine oxide (TMDPO)38 and bis(2,4,6-trimethylbenzoyl)-phenylphosphine oxide (Irgacure 819),34 also have the potential to photoreduce Cu(II).
The photoCuAAC reaction kinetics in the presence of CuCl2/Me6TREN were investigated using RT-FTIR by monitoring the disappearance of the azide band at 2100 cm−1 as a function of time. For comparison, a photoCuAAC reaction catalysed by CuCl2/PMDETA was also assessed (Fig. 4). In the case of the CuCl2/Me6TREN, the azide groups were completely consumed after only 120 s. Evidently, the Me6TREN stabilised the Cu(II)34 to a significant extent, rendering the complex much more reactive. This effect may be associated with the small entropic penalty involved with ligand rearrangement from the Cu(I) to Cu(II) state.34,39
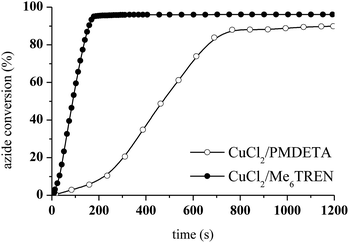 |
| Fig. 4 The effect of the copper complex on the photoCuAAC kinetics. The conversion of ethyl 2-azidopropionate was followed by RT-FTIR at 2100 cm−1 as a function of time. The system consisted of a 1/1 2-azidopropionate/propargyl alcohol molar ratio, 1.7 mol% copper complex and 1.4 wt% ITX (based on the total quantity of azide + alkyne). Irradiation conditions: Hg-Xe lamp filtered with borosilicate glass and total exposure of 20 min at 180 mW cm−2 at room temperature. | |
Effects of the copper complex and photosensitiser concentrations on the photoCuAAC kinetics. Different Cu(II)/L concentrations were employed during the photoCuAAC reaction. Increasing the concentration to 1.7 mol% significantly enhanced the rate of azide consumption and decreased the time needed to reach maximum conversion (between 85 to 96% for all systems). An ITX concentration of 1.4 wt% was found to be sufficient to efficiently sensitise the Cu complex and activate the Cu(II) reduction. Only a slight increase in the reaction rate was observed at an ITX concentration of 2.0 wt% (Fig. 5).
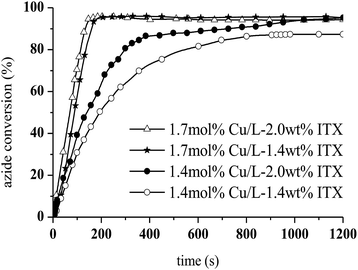 |
| Fig. 5 Conversions of ethyl 2-azidopropionate during the photoCuAAC reaction for different copper complex and ITX proportions, using a 1/1 2-azidopropionate/propargyl alcohol molar ratio. The concentrations of Cu/L and ITX are expressed relative to the total quantity of azide + alkyne. Irradiation conditions: Hg-Xe lamp filtered with borosilicate glass, 180 mW cm−2 and 20 min at room temperature. | |
The resulting triazole product was also characterised by 1H NMR spectroscopy in the liquid state (Fig. 6). Peaks are evident at 7.8 ppm, corresponding to the triazole aromatic group, and at 4.83 and 5.46 ppm, due to the benzylic CH2 and –CH–N groups, respectively. At 1.7 mol% CuCl2/Me6TREN and 1.4 wt% ITX, the photoCuAAC reaction went to completion. These concentrations were thus selected for the following experiments.
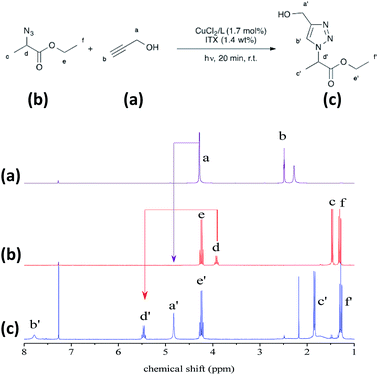 |
| Fig. 6 1H NMR spectra of propargyl alcohol (a), ethyl 2-azidopropionate (b) and the resulting photoCuAAC product (c) after 20 min UV irradiation in CDCl3. The system consisted of a 1/1 2-azidopropionate/propargyl alcohol molar ratio, 1.7 mol% copper complex and 1.4 wt% ITX (relative to the total quantity of azide + alkyne). | |
PhotoCuAAC in the presence of the Michael-addition precursors. As demonstrated by Fig. 7 (circles), the presence of diethylamine did not affect the photoCuAAC kinetics. In contrast, adding methyl acrylate slightly slowed the reaction (Fig. 7, stars) because a concomitant reaction occurred, resulting in a viscosity increase. Indeed, ITX involved in the Cu(II) reduction can also react with the amine acting in this case as a co-initiator to generate aminoalkyl radicals capable of initiating the methyl acrylate polymerisation22 (see Fig. 7, filled circles). Thus, to avoid radical polymerisation during the UV irradiation, a two-step process was considered.
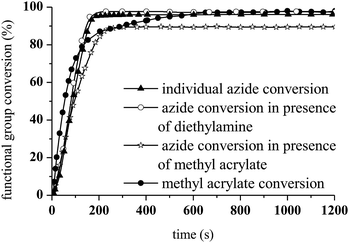 |
| Fig. 7 Effect of the aza-Michael precursor (diethylamine or methyl acrylate) on the photoCuAAC kinetics. The functional group conversions (2100 and 814 cm−1 for the azide and the acrylate groups, respectively) were followed by RT-FTIR as a function of time. The azide/alkyne/amine or acrylate molar ratio was 1/1/1 along with 1.7 mol% copper complex and 1.4 wt% ITX (relative to the total quantity of azide + alkyne). Irradiation conditions: Hg-Xe lamp filtered with borosilicate glass, 180 mW cm−2 and 20 min at room temperature. | |
Aza-Michael reaction
Aza-Michael reaction between diethylamine and methyl acrylate. The reaction between diethylamine and methyl acrylate was initially investigated using liquid state 1H NMR. It should be noted that dissolving the sample in CDCl3 stopped the reaction, thus allowing tracking of the reaction kinetics. The signals between 5.8 and 6.5 ppm correspond to the vinylic (a1, b1, c1) protons in methyl acrylate, while those at 2.44–2.56 ppm and 2.78–2.83 ppm are attributed to protons linked to the α-carbons (a2, f2) and β-carbons (c2) in the aza-Michael product, respectively. The progress of the Michael addition, and thus the generation of the tertiary amine, was followed by monitoring the disappearance of the acrylate peaks at 6.4 ppm (dd), 6.1 ppm (dd) and 5.8 ppm (dd), as shown in Fig. 8.
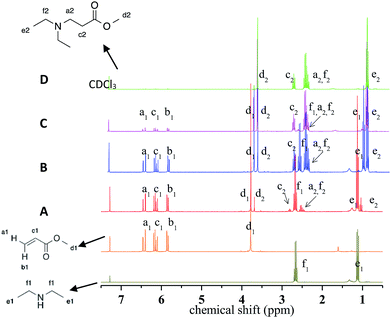 |
| Fig. 8 Evolution of the 1H NMR spectra during the aza-Michael addition between diethylamine and methyl acrylate at a 1/1 molar ratio, after (A) 10 min, (B) 2.5 h, (C) 7.5 h and (D) 24.5 h reaction times. | |
Michael addition in the presence of photoCuAAC precursors. The aza-Michael reaction was performed in the presence of the CuAAC compounds, either added separately or together. As shown in Fig. 9, curve 1, the acrylate conversion reached 50% after 2 h. The addition of 1.7 mol% Me6TREN decreased the rate of the aza-Michael reaction (curve 2), presumably due to a dilution effect. Conversely, the aza-Michael addition was significantly accelerated by the presence of the copper complex (1.7 mol% CuCl2/Me6TREN, curve 3). The same trend was observed when simultaneously adding all the photoCuAAC reactants (alkyne, azide, Cu/L and ITX) (curve 4). Under these conditions, the CuCl2 acted as a Lewis acid catalyst to activate the Michael acceptor, thus promoting the aza-Michael addition.40
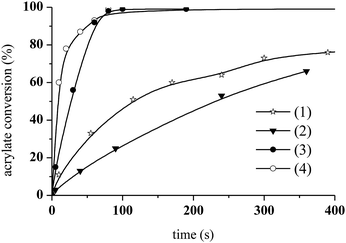 |
| Fig. 9 Kinetics of the aza-Michael reaction (1/1 amine/acrylate molar ratio) as determined by 1H NMR spectroscopy: (1) without any other compounds, (2) with 1.7 mol% Me6TREN, (3) with 1.7 mol% CuCl2/Me6TREN, and (4) with all the photoCuAAC starting compounds. | |
Combination of aza-Michael addition and photoCuAAC reactions. The relationship between the aza-Michael and photoCuAAC reactions was demonstrated in the work described in the preceding sections. Subsequently, a one-pot, two-step sequential process was developed. This strategy can be considered as a combination of thermal aza-Michael and photochemical CuAAC reactions, as shown in Scheme 1.
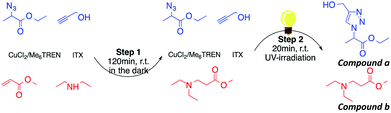 |
| Scheme 1 The combined aza-Michael and photoinduced click reactions in a one-pot two-step sequence. | |
Aza-Michael addition was carried out at room temperature and in the dark. The structure of the tertiary amine thus obtained was confirmed by liquid state 1H NMR, and full conversion of the acrylate was achieved in 80 min (Fig. 10, step 1). Once the aza-Michael reaction was complete, the intermediate mixture was laminated between two BaF2 pellets and exposed to irradiation at room temperature. The photoCuAAC reaction was followed by RT-FTIR analysis and the chemical structure of the final product (a mixture of compounds a and b, Scheme 1) was investigated by liquid state 1H NMR (Fig. 11).
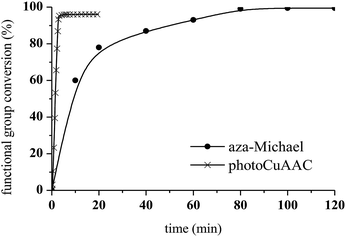 |
| Fig. 10 Reaction kinetics of the one-pot, two-step reaction. The aza-Michael addition proceeds in the dark for 120 min (followed by 1H NMR), after which the light is switched on and the photoCuAAC reaction proceeds for 3 min to give 100% conversion (followed by RT-FTIR). The azide/alkyne/amine/acrylate molar ratio was 1/1/1/1 along with 1.7 mol% copper complex and 1.4 wt% ITX (relative to the total quantity of azide + alkyne). Irradiation conditions: Hg-Xe lamp filtered with borosilicate glass, 180 mW cm−2 and 20 min at room temperature. | |
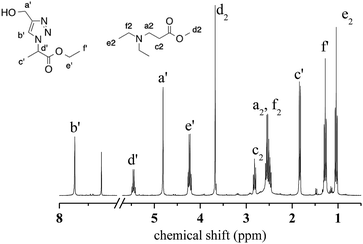 |
| Fig. 11 1H NMR spectrum of the final products resulting from the combination of aza-Michael addition and photoCuAAC reactions. | |
As shown in Fig. 10, the presence of the aza-Michael product in step 2 resulted in a more rapid photoCuAAC reaction compared with the results reported in Fig. 4. The in situ tertiary amine generated via the aza-Michael reaction acted as an oxygen scavenger or co-initiator29 of the photoCuAAC process in the presence of ITX, thus providing a more efficient reaction.
To examine the viability of designing a tailor-made molecule, we investigated the potential of using a bifunctional molecule (propargyl acrylate) capable of reacting with both an amine and an azide (Scheme 2). The concentration of ITX and the copper complex remained unchanged in these trials.
 |
| Scheme 2 One-pot, two-step sequential click reactions with propargyl acrylate, diethylamine and ethyl 2-azidopropionate (1/1/1 molar ratio). Irradiation conditions: Hg-Xe lamp filtered with borosilicate glass, 180 mW cm−2 and 20 min at room temperature. | |
During the aza-Michael addition, full acrylate conversion was obtained after 40 min. This result was confirmed by 1H NMR via the complete disappearance of the acrylate peaks between 6.4 and 5.8 ppm (Fig. 12a). This intermediate mixture was then exposed to UV radiation for 20 min. The presence of the triazole group is demonstrated by the peak at 7.79 ppm in the NMR spectrum, along with the –O–CH2–triazole and –azido peaks at 5.25 ppm (m′) and 5.45 ppm (d′), respectively (Fig. 12b). Peak integration reveals that the photoCuAAC process also achieved 100% conversion. In addition, proton signals attributed to –N–CH2–CH2– groups at 2.52 and 2.82 ppm (h′, k′, i′) are visible. These results clearly confirm that our one-pot, two-step process in which an aza-Michael addition and photoCuAAC reaction are combined is a rapid, simple method that requires no purification step while allowing the synthesis of new triazole-containing materials.
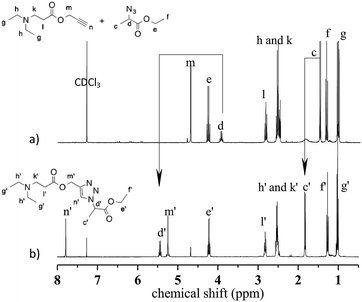 |
| Fig. 12 1H NMR spectra in CDCl3 before (a) and after (b) irradiation of a mixture of propargyl β-diethylaminopropionate and ethyl 2-azidopropionate. | |
Conclusion
A new one-pot, two-step sequential double click process combining aza-Michael and photoCuAAC reactions has been demonstrated. The sequential reactions occurred without separation of intermediates and afford high catalyst selectivity and stability. The aza-Michael addition is a powerful step-growth polymerisation technique that delays the early vitrification commonly encountered in free radical polymerisations. Once the aza-Michael reaction was complete (dark reaction), Cu(I) species required for the photoCuAAC were generated in situ by reduction of the Cu(II)/L complex. Indeed, taking advantage of the orthogonality of the two reactions, it was possible to use light as a trigger and therefore prompt this second reaction on demand in conjunction with high yields under simple conditions at room temperature. These two reactions proceed rapidly under ambient conditions and without any solvent. This combined pathway required optimisation of the reaction conditions and selection of a suitable catalyst for the photoCuAAC reaction, and Me6TREN was used, for the first time, as a copper ligand. This resulted in improved stabilisation of Cu(II), rendering the complex much more reactive and the click reaction faster. Moreover, CuCl2 was found to act as a Lewis acid catalyst for the activation of the Michael acceptor, thus promoting the aza-Michael addition. This synergic effect was taken advantage of in conjunction with a bifunctional molecule capable of participating in both the aza-Michael addition and photoCuAAC reactions. This new approach clearly provides significant advantages that will be applicable to various macromolecular syntheses, including polymer end-group functionalisation and step-growth polymerisation, with the use of appropriate click components. We believe that this process could lead to new possibilities with regard to the synthesis of novel polymeric materials.
Conflicts of interest
There are no conflicts to declare.
Notes and references
- H. C. Kolb, M. G. Finn and K. B. Sharpless, Angew. Chem., Int. Ed., 2001, 40, 2004–2021 CrossRef CAS.
- V. V. Rostovtsev, L. G. Green, V. V. Fokin and K. B. Sharpless, Angew. Chem., 2002, 114, 2708–2711 CrossRef.
- C. W. Tornøe, C. Christensen and M. Meldal, J. Org. Chem., 2002, 67, 3057–3064 CrossRef.
- O. Diels and K. Alder, Justus Liebigs Ann. Chem., 1928, 460, 98–122 CrossRef CAS.
- G. Stork, E. E. V. Tamelen, L. J. Friedman and A. W. Burgstahler, J. Am. Chem. Soc., 1951, 73, 4501 CrossRef CAS.
- G. Stork, E. E. van Tamelen, L. J. Friedman and A. W. Burgstahler, J. Am. Chem. Soc., 1953, 75, 384–392 CrossRef CAS.
- L. M. Campos, K. L. Killops, R. Sakai, J. M. J. Paulusse, D. Damiron, E. Drockenmuller, B. W. Messmore and C. J. Hawker, Macromolecules, 2008, 41, 7063–7070 CrossRef CAS.
- K. L. Killops, L. M. Campos and C. J. Hawker, J. Am. Chem. Soc., 2008, 130, 5062–5064 CrossRef CAS PubMed.
- C. E. Hoyle, A. B. Lowe and C. N. Bowman, Chem. Soc. Rev., 2010, 39, 1355–1387 RSC.
- A. B. Lowe, C. E. Hoyle and C. N. Bowman, J. Mater. Chem., 2010, 20, 4745–4750 RSC.
- G. Kumaraswamy, K. Ankamma and A. Pitchaiah, J. Org. Chem., 2007, 72, 9822–9825 CrossRef CAS PubMed.
- K. L. Heredia, Z. P. Tolstyka and H. D. Maynard, Macromolecules, 2007, 40, 4772–4779 CrossRef CAS.
- A. Dirksen and P. E. Dawson, Bioconjugate Chem., 2008, 19, 2543–2548 CrossRef CAS PubMed.
- M. Bahta, F. Liu, S.-E. Kim, A. G. Stephen, R. J. Fisher and T. R. Burke Jr, Nat. Protoc., 2012, 7, 686–702 CrossRef CAS PubMed.
- C. Wang, D. Ikhlef, S. Kahlal, J.-Y. Saillard and D. Astruc, Coord. Chem. Rev., 2016, 316, 1–20 CrossRef CAS.
- K. Kamata, Y. Nakagawa, K. Yamaguchi and N. Mizuno, J. Am. Chem. Soc., 2008, 130, 15304–15310 CrossRef CAS PubMed.
- K. Yamaguchi, T. Oishi, T. Katayama and N. Mizuno, Chem.–Eur. J., 2009, 15, 10464–10472 CrossRef CAS PubMed.
- M. Grammel and H. C. Hang, Nat. Chem. Biol., 2013, 9, 475–484 CrossRef CAS PubMed.
- E. E. Beauvilliers, M. R. Topka and P. H. Dinolfo, RSC Adv., 2014, 4, 32866–32875 RSC.
- Y. Jiang, D. Kong, J. Zhao, W. Zhang, W. Xu, W. Li and G. Xu, Tetrahedron Lett., 2014, 55, 2410–2414 CrossRef CAS.
- C. Rutz, L. Schmolke, V. Gvilava and C. Janiak, Z. Anorg. Allg. Chem, 2017, 643, 130–135 CrossRef CAS.
- M. F. Mady, G. E. A. Awad and K. B. Jørgensen, Eur. J. Med. Chem., 2014, 84, 433–443 CrossRef CAS PubMed.
- S. C. Ritter and B. König, Chem. Commun., 2006, 4694–4696 RSC.
- M. A. Tasdelen and Y. Yagci, Tetrahedron Lett., 2010, 51, 6945–6947 CrossRef CAS.
- M. A. Tasdelen, G. Yilmaz, B. Iskin and Y. Yagci, Macromolecules, 2012, 45, 56–61 CrossRef CAS.
- B. J. Adzima, Y. Tao, C. J. Kloxin, C. A. DeForest, K. S. Anseth and C. N. Bowman, Nat. Chem., 2011, 3, 258–261 CrossRef PubMed.
- S. Doran, E. Murtezi, F. B. Barlas, S. Timur and Y. Yagci, Macromolecules, 2014, 47, 3608–3613 CrossRef CAS.
- S. Doran and Y. Yagci, Polym. Chem., 2015, 6, 946–952 RSC.
- B. Sandmann, B. Happ, J. Vitz, M. D. Hager, P. Burtscher, N. Moszner and U. S. Schubert, Polym. Chem., 2013, 4, 3938 RSC.
- Y. Yagci, M. A. Tasdelen and S. Jockusch, Polymer, 2014, 55, 3468–3474 CrossRef CAS.
- G. Demirci and M. A. Tasdelen, Eur. Polym. J., 2015, 66, 282–289 CrossRef CAS.
- M. Arslan, G. Yilmaz and Y. Yagci, Polym. Chem., 2015, 6, 8168–8175 RSC.
- H. B. Song, A. Baranek and C. N. Bowman, Polym. Chem., 2016, 7, 603–612 RSC.
- B. D. Mather, K. Viswanathan, K. M. Miller and T. E. Long, Prog. Polym. Sci., 2006, 31, 487–531 CrossRef CAS.
- M. Retailleau, A. Ibrahim, C. Croutxé-Barghorn and X. Allonas, Prog. Org. Coat., 2016, 100, 51–55 CrossRef CAS.
- M. Retailleau, J. Pierrel, A. Ibrahim, C. Croutxé-Barghorn and X. Allonas, Polym. Adv. Technol., 2017, 28, 1208 CrossRef CAS.
- M. Retailleau, A. Ibrahim, C. Croutxé-Barghorn, X. Allonas, C. Ley and D. Le Nouen, ACS Macro Lett., 2015, 4, 1327–1331 CrossRef CAS.
- P. Ferruti, S. Bianchi, E. Ranucci, F. Chiellini and A. M. Piras, Biomacromolecules, 2005, 6, 2229–2235 CrossRef CAS PubMed.
- M. Retailleau, A. Ibrahim, C. Croutxé-Barghorn and X. Allonas, RSC Adv., 2016, 6, 47130–47133 RSC.
- A. A. Alzahrani, D. P. Nair, D. J. Smits, M. Saed, C. M. Yakacki and C. N. Bowman, Chem. Mater., 2014, 26, 5303–5309 CrossRef CAS.
- A. U. Shete and C. J. Kloxin, Polym. Chem., 2017, 8, 3668–3673 RSC.
- E. Maetz, C. Croutxé-Barghorn, C. Delaite and X. Allonas, Polym. Chem., 2016, 7, 7383–7390 RSC.
- G. González, X. Fernández-Francos, À. Serra, M. Sangermano and X. Ramis, Polym. Chem., 2015, 6, 6987–6997 RSC.
- S. Dadashi-Silab, B. Kiskan, M. Antonietti and Y. Yagci, RSC Adv., 2014, 4, 52170–52173 RSC.
- G. Zhang, H. Yi, G. Zhang, Y. Deng, R. Bai, H. Zhang, J. T. Miller, A. J. Kropf, E. E. Bunel and A. Lei, J. Am. Chem. Soc., 2014, 136, 924–926 CrossRef CAS PubMed.
- K. Matyjaszewski, Macromolecules, 2012, 45, 4015–4039 CrossRef CAS.
- W. Tang and K. Matyjaszewski, Macromolecules, 2006, 39, 4953–4959 CrossRef CAS.
- B. P. Kennedy and A. B. P. Lever, J. Am. Chem. Soc., 1973, 95, 6907–6913 CrossRef CAS.
- P. Comba, T. W. Hambley, M. A. Hitchman and H. Stratemeier, Inorg. Chem., 1995, 34, 3903–3911 CrossRef CAS.
- Y. Muto and H. B. Jonassen, Bull. Chem. Soc. Jpn., 1966, 39, 58–64 CrossRef CAS.
|
This journal is © The Royal Society of Chemistry 2019 |
Click here to see how this site uses Cookies. View our privacy policy here.