DOI:
10.1039/C9RA00361D
(Paper)
RSC Adv., 2019,
9, 13297-13303
Clouding observed for surface active, mPEG-grafted silica nanoparticles†
Received
15th January 2019
, Accepted 29th March 2019
First published on 30th April 2019
Abstract
Temperature-dependent phase-separation, clouding, has been observed in suspensions of silica nanoparticles surface-functionalized with methyl-poly(ethylene glycol) silane. Interparticle interactions and conformational changes of the grafted poly(ethylene glycol) chains influence the observed cloud points, and can be controlled by electrolyte concentration and pH. These findings open new routes to tailoring properties of Pickering emulsions.
Introduction
It is well established that nonionic surfactants and polymers containing poly(ethylene glycol) (PEG) exhibit clouding and possess cloud points in aqueous solutions at defined temperature regions.1–4 In contrast to the extensive literature available concerning surfactant systems, cloud point studies in particle suspensions are apparently missing. In this study, we observe an electrolyte-dependent clouding behaviour exhibited by PEG-functionalized silica nanoparticles, and explore its dependence on electrolyte concentration and pH.
The cloud points of PEG-based systems are strongly dependent on co-solutes such as electrolytes, since the solubility of the PEG chains is governed by the hydration of the ethylene oxide (EO) units. Addition of ions that interact strongly with water leads to dehydration of the EO-units at lower temperatures, known as salting-out. Salting-out causes clouding to occur at lower temperatures, and salt-addition can therefore be used to control the clouding temperature.2–6 The type of controlled phase separation that occurs during clouding has applications such as extraction and pre-concentration of for example pollutants, biological material and metal ions.2 Another area where cloud points are important is in emulsion fabrication: knowledge of the cloud point of the emulsifier in an emulsion system can be used to explore phase inversion temperatures (PITs), since clouding occurs close to the PIT.4 At or around the PIT, ultralow surface tension and optimum solubilization of oil or water in surfactant solutions are achieved, enabling low energy emulsification.4,5 Particles can be used as emulsion stabilizers in place of surfactants, in so-called Pickering emulsions.7,8 To the best of our knowledge, clouding behaviour has not been observed for particle suspensions until now. Such behaviour would enable exploiting PIT and clouding properties in surfactant-free systems, and allow for interparticle interactions and particle surface charge to be used as tools to influence emulsion properties.
Results and discussion
By grafting colloidal silica with methyl poly(ethylene glycol) (mPEG) silane, surface active particles are obtained.9 Through this functionalization, we are also able to give the particles clouding behaviour. The sample properties of the functionalized silica particles used in this study are presented in Table 1. Between 18–40% of the added mPEG silane was covalently bound to the silica surface, which means that free mPEG silane was present in all samples.
Table 1 Silica samples used in this study, functionalized with mPEG silane. The specific surface area (SSA) was measured using Sears titration,10 before and after the surface functionalization, and the reduction in available silanol groups is acquired by comparing the obtained SSA values
Sample |
Levasil silica sol |
Amount mPEG silane added (μmol m−2) |
Surface coverage (μmol bound mPEG silane per m2) |
Reduction in available silanol groups |
DLS particle size (volume average, nm) |
|
i |
CS 40-213 |
4 |
0.74 |
78% |
33 |
ii |
CS 40-213 |
2 |
0.85 |
63% |
30 |
iii |
CS 40-213 |
0.9 |
0.16 |
35% |
31 |
CS 40-213, non-functionalized |
— |
— |
— |
23 |
Confirmation of clouding behavior of functionalized particles
The results consistently show that cloud points exist in suspensions of mPEG-grafted silica nanoparticles, if the surface coverage and mPEG content is high enough to induce a steric stabilization towards irreversible aggregation. Fig. 1a–d shows the reversible cloud point phenomena of sample ii, which has a high mPEG coverage, giving the appearance before clouding (a), during clouding (b and c), and after cooling, when its original, low turbidity is regained (d). Fig. 1e shows sample iii, which has a low mPEG-coverage, at room-temperature after it has been heated and then cooled. The addition of moderate amounts of electrolyte (0–0.4 M CaCl2) together with an elevated temperature resulted in a distinct increase of turbidity, but upon cooling the sample remained cloudy. At higher salt concentrations (≥0.5 M) aggregation of the silica particles in sample iii occurs at room-temperature, probably due to salt-induced gelation.11 It could thus be determined that, in contrast to sample ii and i, particles with low surface coverage of mPEG (sample iii) do not show reversible phase separation, why only sample ii and i were used for further cloud point studies. Addition of low amounts of calcium chloride to non-functionalized silica particles results in particle aggregation and gelation, why such particles cannot be explored for cloud point studies.
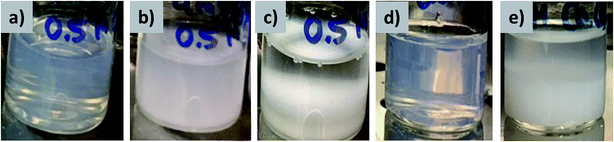 |
| Fig. 1 The appearance of mPEG functionalized silica particles at 2 wt% SiO2 and containing 0.5 M CaCl2 at varying temperatures. (a) Sample ii (high mPEG coverage) at 70 °C; before the cloud point, (b) sample ii at 79 °C; at the onset of clouding, (c) particle sedimentation that occurs upon storing at the elevated temperature for sample ii. When the suspension has been cooled down to room temperature the original turbidity is regained (d). (e) Shows sample iii (low mPEG coverage) containing 0.1 M CaCl2, cooled to room temperature, after it has been heated. This suspension remains cloudy and sedimentation of silica aggregates occurs. | |
To evaluate the reversibility of the clouding, the particle size of selected samples was measured using dynamic light scattering (DLS) and the results are shown in Table 2. The original particle size is regained after the heat treatment, except for sample ii at 1.5 M CaCl2, where a slight increase in particle size was noted, due to the very high electrolyte content, which together with the elevated temperature leads to a slight particle aggregation. UV-Vis measurements confirm the reversibility, where the initial absorbance is regained after a sample has been passed through its cloud point (see ESI, Fig. S1† for details).
Table 2 Particle sizes measured using DLS (average of five replicates), at varying pH and salt contents. The particle sizes were measured twice; (i) after salt addition but before heating, and (ii) at room temperature after heating the samples to a temperature above their cloud points
Sample |
pH |
CaCl2 concentration (M) |
dp,vol before heating (nm) |
Heated to (°C) |
dp,vol after heating (nm) |
Sample i |
8 |
0.3 |
34.3 ± 0.9 |
80 |
34.9 ± 0.9 |
Sample ii |
7 |
0.3 |
34.1 ± 0.9 |
95 |
34.3 ± 0.9 |
Sample ii |
4 |
1.5 |
38.9 ± 0.5 |
80 |
41.7 ± 0.9 |
Salt influence on cloud point
In agreement with the behaviour of nonionic surfactants, a clear influence of the electrolyte concentration on the cloud point is observed, shown in Fig. 2. A linear correlation between the cloud point and the salt concentration is normally obtained for surfactant systems.12,13 Here, however, a non-linear dependence is observed, where at low salt concentrations (<0.5 M), a 4–6 times steeper slope is obtained compared to the high salt concentration range (0.5–1.5 M), where the steeper slope deviates from the behaviour of surfactants. In surfactant systems, temperature-controlled, intramolecular conformational changes of the PEG-chain is the cause of clouding; less polar conformations are dominating at higher temperatures.1
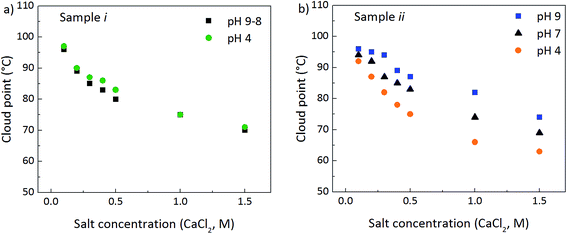 |
| Fig. 2 Observed cloud points in suspensions of mPEG-functionalized silica particles, as a function of salt concentration and at different pH conditions. Since visual inspection was used for cloud point determination, an error of ± 2 °C should be accounted for. (a) Sample i with 0.74 μmol bound mPEG silane per m2 at 3.5 wt% SiO2 as a function of CaCl2 concentration. The samples contain roughly 160 mm of free, unreacted mPEG silane. (b) Sample ii, with 0.85 μmol bound mPEG silane per m2 at 2 wt% SiO2, containing roughly 60 mm of free, unreacted mPEG silane. | |
In our case intramolecular, intermolecular as well as interparticle forces may influence the colloidal behaviour of the suspensions, which is why the addition of salt affects the system in several ways. On one hand, the silica particles as such are affected and on the other hand the mPEG chains are influenced through salting-in and salting-out effects as well as increased or decreased adsorption of the polymer onto to silica surface.4,14,15 At the lower salt concentration range, where the steeper slope is observed, the high particle charge counteracts clouding, and salt addition is vital; when no salt was added to the suspensions, no cloud points were observed at the temperatures studied (the samples were not heated above 110 °C for practical reasons). Zackrisson et al.16,17 found for unfunctionalized colloidal silica that if the ionic strength is low, an initial reversible aggregation or cluster formation occurs, until a certain electrolyte concentration is reached, where irreversible aggregation follows. The effect of increasing the salt concentration is therefore expected to be more effective when the ionic strength is low, explaining the steeper slope in that region. A high ionic strength promotes clouding, since the particle charges are screened, leading to the lower clouding temperatures observed. The observation that the behaviour is more similar to surfactant systems at the higher ionic strengths indicates that clouding is here more affected by salting-out effects of the PEG-polymer chains present in the sample. In the present study, irreversible aggregation of the silica particles is avoided due to the steric stabilization achieved by the mPEG functionalization.
Most experiments were performed using CaCl2. Exchanging the salt for NaCl gives a slight increase in the cloud point temperature at lower salt concentrations, for both samples i and ii (Fig. 3). According to from Schulze–Hardy rule, a higher valency of the ion should significantly decrease the critical coagulation concentration of colloidal particles.6 Here, the valency of the cation has a relatively small effect on the clouding. This, together with the DLS results shown in Table 2, demonstrates that the salt does not cause aggregation of the particles, it merely reduces the influence of particle–particle repulsion due to the screening of particle surface charge, and what we observe is a phase separation.
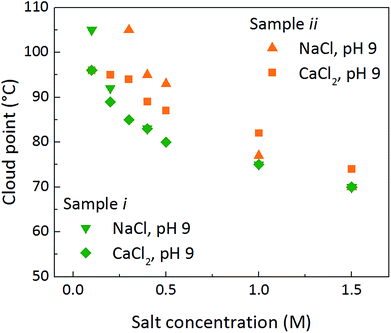 |
| Fig. 3 Cloud points observed at pH 9, as a function of NaCl and CaCl2 concentrations (sample i, with high mPEG coverage and high free mPEG silane content, at 3.5 wt% SiO2 and sample ii, with high mPEG coverage but lower free mPEG content, at 2 wt% SiO2). Since visual inspection was used for cloud point determination, an error of ± 2 °C should be accounted for. | |
pH effects
The pH of the suspensions also has effects on the particle surface charge, as well as on the PEG-silica interactions, in turn affecting the cloud point. PEG-chains are attracted to silica surfaces below, but not above, pH 8.18–21 Since the attraction decreases with increasing pH, PEG chains will protrude out from the particle surfaces at pH 9. A low pH, on the other hand, decreases the silica surface charge and promotes adsorption of the mPEG chains, enabling PEG-interparticle interactions. A pH induced flocculation behaviour of PEG-grafted silica particles is known to occur for low surface coverages, whereas for high coverages steric stabilization is achieved.9 Slightly lower cloud point temperatures can therefore be observed at lower pH conditions, but irreversible aggregation is avoided.
Particle surface charge effects. The colloidal stability is affected by the pH; although silica is metastable around pH 2, lower pH conditions results in reduced stability due to the lower particle surface charge density. Varying the pH has no significant effect on the cloud points of sample i (Fig. 2a), but a decreased pH results in slightly decreased cloud point temperatures for sample ii (Fig. 2b). Even though sample ii has a higher amount of bound mPEG silane, it has a lower total amount of mPEG silane content, reducing the possibility of the stabilizing effect achieved by the presence of free mPEG in the suspension at low pH, where the particle surface charge is reduced.Fig. 4a shows the surface charge densities (SCD) per square meter of particle surface, over the measured pH range, obtained from potentiometric titrations. The point of zero charge (PZC) is set to be located at pH = 3 for all samples and the influence of salt was eliminated through titrations of blank salt solutions.22 A small difference in the shape of the curve is observed when comparing sample iii to non-modified silica, and the sample had an increased turbidity and viscosity after the titration due to the above mentioned flocculation phenomenon. The measurement error is relatively low; however, very small differences must still be considered with care (see ESI, Fig. S3† for SCDs of repeated experiments). When the surface coverage of mPEG is increased (sample i and ii), an effect on the SCD can be seen at high pH conditions.
The addition of salt to samples i and ii results in the screening of particle surface charge, enabling the particle to hold a higher SCD (Fig. 4b). The mPEG modification, which protects the system from particle aggregation, provides the possibility to use the salt concentration for controlling the cloud point. The results are in line with previous discussion, where the influence of the PEG polymer is more important at the higher salt concentrations: the effect of adding 0.2 M CaCl2 to the suspensions without added salt is much more pronounced compared to increasing the salt concentration from 0.2 to 1 M, since most of the particle charge is screened already 0.2 M CaCl2.
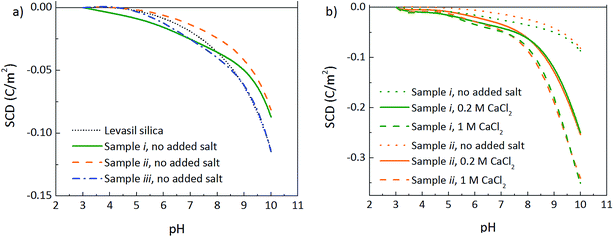 |
| Fig. 4 Surface charge densities (SCDs), per square metre of silica surface, as a function of measured pH, performed at 2 wt% SiO2; (a) without added salt and (b) in the presence of CaCl2. | |
It was further noted that the addition of CaCl2 to the silica suspensions leads to a decrease in pH, i.e. from pH 9.8 when no salt has been added, to pH 7.7 at 1.5 M CaCl2. A possible explanation is that the salt addition to the silica suspensions leads to a screening of the silica surface charges, allowing adsorption of the OH− on the silica surface, in turn reducing the pH.10,23 Also, addition of salt to the charged silica particles results in a higher concentration of counter ions, available to counterbalance the surface charge. H+ ions can be more easily released and are available for neutralizing OH−, thereby shifting the pH.
mPEG stabilization and salting-out effects
The influence of free mPEG silane. The colloidal stability of the silica particles is affected by the amount of free, unbound mPEG silane present in the sample, which in turn influences the cloud point. At high pH, the samples with a lower amount of free mPEG present (sample ii) remain cloudy after being passed through their cloud points, if kept at high temperature for more than 20 minutes before being cooled, but this does not occur for sample i. High pH catalyses the formation of ion-bridges and siloxane bonds through condensation reactions, which promotes aggregation of the silica particles,24,25 but this process can be avoided with a higher total amount mPEG in the suspension. A steric effect preventing aggregation can therefore exist for sample i, where the free silanes present in the suspension aid this stabilization mechanism though adsorbing in thicker layers, or possibly double layers. The particles cannot come close enough to gel and the pH dependence is suppressed. When the suspensions of sample ii were cooled quickly on ice after clouding, the original, low, turbidity was regained and gelation avoided, since the aggregation process is time-dependent.16To further assess the clouding behaviour in the absence of free mPEG, part of sample ii was purified through ultra-filtration (UF) before the cloud point experiments, removing around 80% of the un-reacted mPEG silane and reducing the inherent ionic strength of the suspension. The purification treatment gives a reduction in the cloud point of 2–12 °C, where the effect is larger at higher salt concentrations (see Fig. 5). The purified samples remained somewhat cloudy, despite fast cooling, indicating that slight aggregation of the silica particles occurs, verified by DLS measurements (see Table S2, ESI,† for DLS sizes of heated and cooled, purified samples). This suggests an irreversible aggregation effect caused by a slight gelation of silica particles, due to an insufficient amount of bound mPEG in the sample.
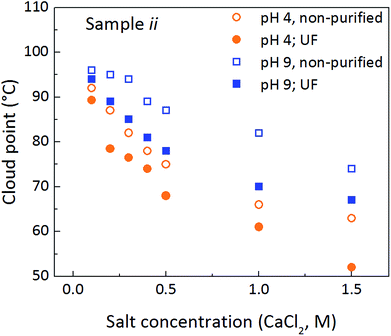 |
| Fig. 5 Observed cloud points in suspensions of mPEG-functionalized silica particles purified using ultra-filtration (UF), as a function of salt concentration and at different pH conditions, with 0.85 μmol bound mPEG silane per m2 at 2 wt% SiO2. Non-purified samples are shown for comparison (open symbols). Since visual inspection was used for cloud point determination, an error of ± 2 °C should be accounted for. | |
Solutions with 0.1–1.5 M CaCl2, without silica particles, containing very low amounts of free mPEG (3 mM) were analysed and found not to cloud, even when heated to 110 °C. Clouding is, however, observed for solutions with higher free mPEG concentrations (100, 200 and 295 mM), also without salt addition, but at lower temperatures compared to the functionalized silica particle system (see ESI, Fig. S2†). Solutions of free mPEG, without particles, containing 1–1.5 M CaCl2 cloud at 40–50 °C, but remain cloudy after the heat treatment, indicating irreversible aggregation of the mPEG silanes, possibly due to condensation of the silanol groups. With silica particles present in the samples, adsorption of the mPEG silanes on the available surface of the particles is possible, which can counteract the phase separation.
Based on the discussion above, we find that a synergistic effect between bound and free mPEG silane is active. The mPEG silane alone clouds, but at lower temperatures compared to the particle-mPEG system. At high salt contents irreversible aggregation of the silanes occurs. Functionalized silica particles, which have been purified to remove free mPEG silane, also display clouding, also at lower temperatures compared to the non-purified samples. However, irreversible particle aggregation occurs at high salt concentrations. Systems containing both surface functionalized silica particles and free mPEG silane, display a controllable and reversible clouding behavior, where only a very slight aggregation is observed at very high salt contents (1.5 M CaCl2).
mPEG adsorption and salting-out. 1H NMR measurements were conducted, to shed more light on the PEG-silica interaction, PEG adsorption onto the silica surface and possible salting-out effects, since, by comparing the integral intensities of the PEG-polymer peak, information regarding the mobility of the PEG polymer chain can be obtained.9 Spectra of sample ii were recorded at increasing temperatures (30–90 °C), at four different pH conditions (pH 2, 4, 7 and 9) and at two different salt concentrations (without added CaCl2 and at 1 M CaCl2). Fig. 6 shows the integral intensity of the PEG polymer signal, where a decrease in signal intensity of about 50% occurs when CaCl2 is added to the suspensions, compared to the suspensions without added salt. When the samples display clouding, an additional decrease in intensity, of 40–60%, occurs.
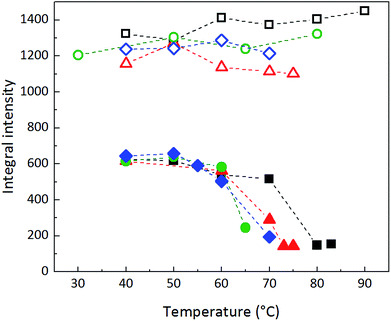 |
| Fig. 6 The integral intensities, obtained from 1H NMR measurements, of the peak arising from the poly(oxy ethylene) units of the mPEG silane of sample ii as a function of temperature, with no added salt (open symbols) and at 1 M CaCl2, at the following pH conditions: pH 9 (black ■/□); pH 7 (red ▲/Δ); pH 4 (green ●/○); pH 2 (blue ♦/◊). The intensity values have been compensated for signal loss due to temperature increase. | |
The NMR results point to a clear salting-out effect of adding CaCl2 to the suspension: water becomes a poorer solvent to the mPEG silane, making the PEG chains seek each other and possibly adsorb as thicker layers around the particle.14,15 The increased PEG adsorption onto the silica surface and the increased PEG–PEG interactions decrease the mobility of the PEG chains and thereby the integral intensity (see also Fig. S5, ESI†). In contrast, changing the pH of the suspensions gives no apparent change in the mPEG mobility, since the pH is not affecting the solvent abilities of the water. The pH only has an effect when the silica surface is sparsely covered with mPEG, but not when the surface coverage is high.9 At the cloud point, particle-rich and PEG-rich domains are created, and the mobility is reduced further.
Conclusions
Silica particles functionalized with mPEG silane exhibit an interesting and reversible clouding phenomenon, influenced by the interplay between particle–particle interactions, PEG-silica attractions and intramolecular effects. The grafting density, pH and salt concentration are all parameters that influence the behaviour and can be tuned; high salt concentrations and low pH promote clouding, while a high amount of grafted mPEG counteracts particle aggregation. A synergistic effect is found when free mPEG is present in the suspension of functionalized silica particles, allowing for a reversible clouding behaviour at very high salt concentrations (0.5–1.5 M CaCl2). These findings show the versatility of grafted silica particles and could find use for development of emulsion systems using for example the PIT method.
Experimental section
Functionalization procedure and determination of surface coverage
Sodium ion-stabilized silica sol having a specific surface area (SSA) of 130 m2 g−1, as measured by Sears titration,10 with the trade name Levasil CS 40-213 were provided by Nouryon. The particles were functionalized with methyl poly(ethylene glycol) silane (Silquest A-1231 from Momentive). The silane has a molecular weight of 679 g mol−1, with 11 ethylene oxide units.9 The surface coverage was determined using a combination of ultracentrifugation and elemental analysis. This methodology and the functionalization procedure are described in ESI.†
Cloud point determination
To study the cloud point behaviour, diluted and pH-adjusted suspensions with varying electrolyte concentrations were heated in an oven with controlled temperature and their appearance was visually inspected. The temperature at which a distinct increase in turbidity occurred was recorded as the cloud point. Upon cooling, the suspension must regain its original, low, turbidity. If the suspension remains turbid, an irreversible aggregation distinct from phase separation has occurred. The pH was adjusted using HCl (aq) or NaOH (aq) and the salts examined were NaCl and CaCl2. In addition, the particle size of selected systems was monitored using dynamic light scattering (DLS) and UV-Vis spectroscopy, to confirm that severe particle aggregation did not occur.
Potentiometric titrations
Titration experiments were performed using the titration device Titrando 905 (Metrohm) equipped with a pH glass electrode of model Unitrode easyClean (Pt1000). The suspensions were diluted to 2 wt% SiO2 and a total volume of 75 ml was titrated from pH 10 to 3 with HCl (0.1 M). The suspension was purged with N2 gas for 10 min and the pH was adjusted with NaOH (0.1 M) prior to titration. The atmosphere inside the glass vessel was kept inert throughout the entire experiment, through a flow of N2 gas. Optimized titration settings were used, based on the work from Simonsson et al.22 The surface charge density (SCD) was calculated based on the difference between the volumes of acid required for obtaining a certain pH in the silica suspensions and in blank solutions without particles, but with the corresponding salt concentrations.22,26
Nuclear magnetic resonance (NMR) spectroscopy
Sample ii was diluted from 35 wt% to 10 wt% SiO2 in D2O with the desired amount of CaCl2, and ion-exchanged using Amber-Jet 1500H (Dow), to adjust the pH. 1H NMR spectra were recorded on a 500 Varian spectrophotometer, with the relaxation delay set to 10 s, at increasing temperatures. The samples were allowed to equilibrate for 10 min after each change in temperature. The integral intensities were compensated for the loss of signal due to the increased temperature.
Conflicts of interest
There are no conflicts to declare.
Acknowledgements
The authors would like to acknowledge the Swedish Research Council (VR) and Nouryon (Bohus, Sweden) for financial support of this project, Drs Kristina Lundahl and Anders Törncrona, and Adj. Prof. Michael Persson, Nouryon, for fruitful discussions, Isabelle Simonsson (Prof. Zareen Abbas group, Gothenburg University) for lab-help during the potentiometric titration measurements, and Christian Sögaard (Prof. Zareen Abbas group, Gothenburg University) also for lab-help as well as for providing a MatLab program for processing the potentiometric titration data, Drs Bijan Nekoueishahraki (Gothenburg University) and Yuan Fang (Chalmers University of Technology) for assistance during the NMR measurements, and the analysis lab in Bohus for performing elemental analysis and HPLC measurements.
Notes and references
- K. Holmberg, B. Jönsson, B. Kronberg and B. Lindman, Surfactants and Polymers in Aqueous Solution, John Wiley & Sons, Ltd, 2 edn, 2002 Search PubMed.
- P. Mukherjee, S. K. Padhan, S. Dash, S. Patel and B. K. Mishra, Adv. Colloid Interface Sci., 2011, 162, 59–79 CrossRef CAS PubMed.
- K. Shinoda and H. Arai, J. Phys. Chem., 1964, 68, 3485–3490 CrossRef CAS.
- L. Marszall, in Nonionic Surfactants: Physical Chemistry, ed. M. J.Schick, Marcel Dekker Inc., New York, 1987, vol. 23 Search PubMed.
- A. Perazzo, V. Preziosi and S. Guido, Adv. Colloid Interface Sci., 2015, 222, 581–599 CrossRef CAS PubMed.
- Y. Chu, J. Chen, F. Haso, Y. Gao, J. E. S. Szymanowski, P. C. Burns and T. Liu, Chem.– Eur. J., 2018, 24, 5479–5483 CrossRef CAS PubMed.
- S. U. Pickering, J. Chem. Soc., Trans., 1907, 91, 2001–2021 RSC.
- W. Ramsden, Proc. R. Soc. London, 1903, 72, 156–164 CAS.
- S. M. S. Björkegren, L. Nordstierna, A. Törncrona, M. E. Persson and A. E. C. Palmqvist, J. Colloid Interface Sci., 2015, 452, 215–223 CrossRef PubMed.
- G. W. Sears, Anal. Chem., 1956, 28, 1981–1983 CrossRef CAS.
- Colloidal Silica: Fundamentals and Applications, ed. H. E.Bergna and W. O.Roberts, Taylor & Francis, 2006 Search PubMed.
- M. Mohsen-Nia, H. Rasa and H. Modarress, J. Chem. Eng. Data, 2006, 51, 1316–1320 CrossRef CAS.
- S. Nozary, H. Modarress and A. Eliassi, J. Appl. Polym. Sci., 2003, 89, 1983–1990 CrossRef CAS.
- B. R. Postmus, F. A. M. Leermakers and M. A. Cohen Stuart, Langmuir, 2008, 24, 1930–1942 CrossRef CAS PubMed.
- Z. Chang, X. Chen and Y. Peng, Miner. Eng., 2018, 121, 66–76 CrossRef CAS.
- A. Schantz Zackrisson, A. Martinelli, A. Matic and J. Bergenholtz, J. Colloid Interface Sci., 2006, 301, 137–144 CrossRef CAS PubMed.
- A. S. Zackrisson, J. S. Pedersen and J. Bergenholtz, Colloids Surf., A, 2008, 315, 23–30 CrossRef CAS.
- F. Lafuma, K. Wong and B. Cabane, J. Colloid Interface Sci., 1991, 143, 9–21 CrossRef CAS.
- M. Malmsten, P. Linse and T. Cosgrove, Macromolecules, 1992, 25, 2474–2481 CrossRef CAS.
- J. Rubio and J. A. Kitchener, J. Colloid Interface Sci., 1976, 57, 132–142 CrossRef CAS.
- A. Sundblom, A. E. C. Palmqvist and K. Holmberg, Langmuir, 2010, 26, 1983–1990 CrossRef CAS PubMed.
- I. Simonsson, C. Sögaard, M. Rambaran and Z. Abbas, Colloids Surf., A, 2018, 559, 334–341 CrossRef CAS.
- R. K. Iler, The Chemistry of Silica, Wiley, New York, 1979 Search PubMed.
- J. Depasse and A. Watillon, J. Colloid Interface Sci., 1970, 33, 430–438 CrossRef.
- Y. G. Frolov and N. A. Shabanova, Langmuir, 1987, 3, 640–644 CrossRef CAS.
- J. Lützenkirchen, T. Preočanin, D. Kovačević, V. Tomišić, L. Lövgren and N. Kallay, Croat. Chem. Acta, 2012, 85, 391–417 CrossRef.
Footnote |
† Electronic supplementary information (ESI) available: The functionalization procedure of the silica particles and the methodology for determining the surface coverage, DLS particle sizes of selected samples, UV-Vis measurements, cloud points of free mPEG silane, additional potentiometric titrations, and additional integral intensities (from NMR measurements). See DOI: 10.1039/c9ra00361d |
|
This journal is © The Royal Society of Chemistry 2019 |
Click here to see how this site uses Cookies. View our privacy policy here.