DOI:
10.1039/C9RA01111K
(Paper)
RSC Adv., 2019,
9, 13820-13828
Rosette-like MoS2 nanoflowers as highly active and stable electrodes for hydrogen evolution reactions and supercapacitors†
Received
13th February 2019
, Accepted 28th April 2019
First published on 3rd May 2019
Abstract
MoS2 is regarded as one of the cost-effective materials for many important applications. In this work, we report a simple one-step hydrothermal method for the directed synthesis of a rosette-like MoS2 nanoflower modified electrode without using adhesion agents. Interestingly, owing to the hierarchical structures, the as-prepared MoS2-based electrode exhibits significantly enhanced performance for both the hydrogen evolution reaction in acidic environments and supercapacitors. When used in the hydrogen evolution reaction, the electrode shows a low overpotential of ∼0.25 V at 10 mA cm−2, a Tafel slope of ∼71.2 mV per decade, and long-term durability over 20 h of hydrogen evolution reaction operation at 10 mV cm−2. In addition, as a supercapacitor electrode, it exhibits a good capacity of 137 mF cm−2 at a current density of 10 mA cm−2 and excellent stability in 1 M H2SO4 at a scan rate of 50 mV s−1. The outstanding performances of the as-prepared materials may be ascribed to the unique 3D architectures of the rosette-like MoS2 nanoflowers. This work could provide a strategy to explore low-cost and highly efficient electrocatalysts with desired nanostructures for the hydrogen evolution reaction and supercapacitors applications.
1. Introduction
Nowadays, owing to concerns regarding the environment and the gradual depletion of nonrenewable resources, for example coal, oil and natural gas, developing sustainable and clean energy sources has become an effective way for human beings to deal with the energy crisis.1–3 Hydrogen, as a clean and sustainable energy carrier,4,5 is an economic fuel in electric devices.6 The low cost, high efficiency and renewable electric devices, such as supercapacitors,7 lithium ion batteries (LIBs)8,9 and fuel cells, have become important alternative electrochemical devices to fossil fuels. The hydrogen evolution reaction (HER) is an effective and convenient process to produce molecular hydrogen from water. To our knowledge, noble Pt and Pt-based materials are regarded as the most effective and catalytically stable electrocatalysts for HER,10 particularly in acid solution.11,12 However, global-scale application is challenged by their high cost and limited supply.13–15 Recently, tremendous effort has focused on investigating the possibility of earth abundant elements catalytic performances HER electrocatalysts for large-scale hydrogen production,16 such as sulfide,17,18 nitride,19 boride,20 and phosphide.21 Among them, molybdenum disulfide (MoS2) based on molybdenum element possesses attracting electrochemical properties, for instance, defective nanosheets,22 porous films,23 double-gyroid structures,24 nanowires25 and amorphous films.26 Especially, the MoS2 of single-layer, few-layer or re-stacked layer forms held together by van der Waals forces has been widely used as electrocatalyst for HER.27–30 It is well known that two-dimensional (2D) layered MoS2 possesses unique electrical and chemical properties due to their large exposure of active sites31 caused by the coordinatively unsaturated sulfur atoms at the edge sites.32 Meanwhile, MoS2 has also been considered as an ideal material for supercapacitors, which is due to that the ultrathin layer forms structure has large surface area for double-layer charge storage and the central Mo atoms has great potential for pseudocapacitance from the H+ intercalation into the stacked layers.33 And the interlayer spacing (002) is crucial for the insertion of foreign atoms34 and electrolyte diffusion. Nevertheless, the synthesis of large layer forms MoS2 nanostructure is still a challenge because of the difficulty of preparation of these species.
Also, the utilization of 2D nanomaterials suffers from inadequate contact with electrolyte and low surface-area-utilization efficiency. In order to resolve this problem, extensive efforts have been devoted to assembling 2D materials into 3D architecture.35,36 The construction of 3D framework is a best strategy to increase the diffusion of the ions and electrolyte into materials, which is favorable for promoting the electrochemical performance of the materials.
Herein, we report a simple strategy to synthesize an interlayer spacing-enlarged rosette-like MoS2 nanoflowers for both hydrogen evolution reaction and supercapacitive energy storage. In the synthesis, (NH4)6Mo7O24·4H2O was used as a Mo resource and thiourea was used as a S resource, and the rosette-like MoS2 nanoflowers modified electrode was prepared by a one-step hydrothermal method. The synthesized novel MoS2 structure has the advantages as following: (i) simple and environmental friendly preparation route; (ii) 3D interconnected frameworks to facilitate the electrolyte diffusion; (iii) much more electrochemically active sites to deliver excellent catalytic properties in HER; (iv) the active materials grown on Ti foil directly used as electrode for electrochemical performance testing; (v) larger interlayer spacing which is beneficial for ion intercalation into S–Mo–S stacked layer; (vi) more importantly, the as-prepared MoS2 composites are suitable for both HER and supercapacitors.
2. Experimental
2.1 Chemicals
(NH4)6Mo7O24·4H2O (Aladdin, 99%) and thiourea (Aladdin, ≥99%) were of analytical grade and used without further purification.
2.2 Synthesis of MoS2
Typically, 0.57 mmol (NH4)6Mo7O24·4H2O and 6 mmol thiourea were dissolved into 36 mL of deionized (DI) water in 40 mL Teflon-lined stainless-steel autoclave. After sonicating 20 min, a titanium sheet (0.2 mm, 1 × 5 cm) which was sonicated in 2 M HCl, ethanol and DI water respectively was immersed into the solution. Then, the autoclave was kept at 180 °C for 12 h. After naturally cooling down, the titanium sheet was washed with a large amount of ethanol and DI water, and dried in a vacuum oven at 70 °C for several hours. For comparison, the MoS2 was also synthesized at the same conditions with synthesis time of 6 and 20 h.
2.3 Characterizations
The crystalline structure of the as-prepared MoS2 nanoflowers was characterized by power X-ray diffraction (XRD) on a D/max2550VB3+/PC X-ray diffractometer with a Cu Kα radiation source (λ = 0.15418 nm). The general morphologies of samples were analyzed by scanning electron microscopy (SEM, Hitachi S-4800) with an X-ray energy dispersive spectroscopy (EDS) analysis system. Transmission electron microscopy (TEM, FEI TF30) and selected area electron diffraction (SAED) were used to observe the morphological properties of the samples. X-ray photoelectron spectroscopy spectra (XPS, EP13-002) were used to investigate the chemical composition.
2.4 Electrochemical measurements
All the electrochemical experiments were conducted using a CHI660C electrochemical workstation (Shanghai Chenhua Instruments Co.) with a standard three-electrode cell. And the rosette-like MoS2 nanoflowers grown on titanium sheet were directly used as working electrodes and graphite rod was used as counter electrode for both HER performance and supercapacitor testing.
2.4.1 HER measurements. The saturated calomel electrode was used as reference electrode and all the tests were performed in a N2 saturated 0.5 M H2SO4 electrolyte. Linear sweep voltammetry (LSV) was performed at scan rates of 2 mV s−1 with the potential range of –0.6 V to 0 V. The electrochemical impedance spectroscopy (EIS) were performed at open circuit potentials by using the frequency range of 0.1 to 100 kHz with an AC voltage of 5 mV. The stability of the HER was evaluated by amperometric I–t measurement at the current density of 10 mA cm−2 for 20 h. All the potentials were converted to the RHE based on the equation E(RHE) = E(SCE) + (0.242 + 0.059pH) V.
2.4.2 Supercapacitor measurements. The reference electrode was Ag/AgCl electrode, and a 1 M H2SO4 was employed as the electrolyte. Cyclic voltammetry (CV) measurements was performed from 10 to 800 mV s−1 within a potential range between −0.2 and 0.4 V. EIS tests were carried out with frequency ranging from 0.01 Hz to 100 kHz with an ac signal amplitude being set as 5 mV. Galvanostatic discharge/charge (GCD) curves were collected at current densities ranging from 1 to 25 mA cm−2. The stability of the supercapacitor was evaluated by repeating the CV measurement at a scan rate of 50 mV s−1 for 10
000 cycles. In a three-electrode system, the specific capacitance was calculated based on CV and GCD curves by eqn (1) and (2), respectively: |
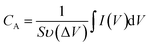 | (1) |
where S is the geometrical area of each film, υ is the scan rate, ΔV is the working potential window, I(V) is the response current. |
 | (2) |
where I is the discharge current, Δt is the discharge duration, S is geometrical area of each film and ΔV is the discharge potential window with deduction of IR drop.
3. Results and discussion
3.1 Synthesis of rosette-like MoS2 nanoflowers on Ti foil
Scheme 1 describes the entire fabrication process of rosette-like MoS2 nanoflowers architectures. Briefly, ammonium molybdate and thiourea were used as Mo and S resources, respectively. With temperature increasing, H2S was generated through the reaction of thiourea and water (reaction (3)). Due to the strong reducibility of the H2S, the Mo(VI) atom in Mo7O246− ion could be reduced to Mo(IV) atom, and the Mo(IV) atom reacted with H2S to produce MoS2 according to the reaction (4).37 As a result, the interlayer S–Mo–S atoms is strongly covalently linked by weak van der Waals forces, and the 3D hierarchical of the rosette-like MoS2 nanoflowers directly grown on the bottom of the titanium sheet (Fig. S1, ESI†) by the bridge bonds.38 The whole process was simple, and the hydrothermal reaction is excellent environment-friendly and low-cost. |
C2H5NS + H2O → CH3CONH2 + H2S
| (3) |
|
Mo7O246− + H2S → MoS2 + 3H2 + SO42−
| (4) |
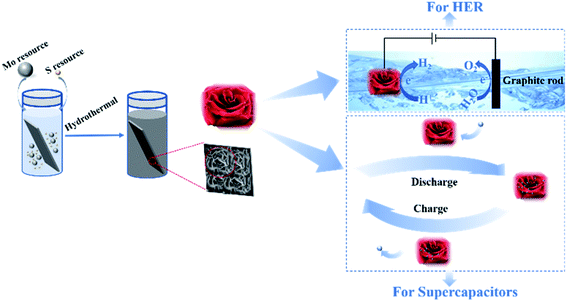 |
| Scheme 1 Scheme description of the synthetic route of the spacing-enlarged rosette-like MoS2 nanoflowers that exhibit a remarkable electrochemical performance in both HER and supercapacitors. | |
The morphology and structure of the as-prepared samples is displayed in Fig. 1a, indicating the successful synthesis of rosette-like MoS2 nanoflowers. In addition, the image (Fig. 1a) clearly reviews that the diameter of the nanoflowers is about 300 nm. The high-magnification SEM images of the nanoflowers in Fig. 1b and c show that the nanoflowers consists of ultrathin nanosheets with the thickness less than 15 nm. The novel architecture of the MoS2 is in favor of rapid electron transfer during charge/discharge processes.39 In addition, the MoS2 nanomaterials did not exhibit the rosette-like morphology (Fig. S2, ESI†) when the reaction time was 6 h and 20 h. It is speculated that the rosette-like MoS2 nanoflowers morphology cannot be formed with a relatively shorter reaction time. However, the surface of the nanosheets became disordered with a longer reaction time of 20 h. The uniform distribution of elements Mo and S throughout the whole rosette-like MoS2 nanoflowers can also be evidenced by elemental mapping images (Fig. S3, ESI†). Additionally, the crystalline structure of rosette-like MoS2 nanoflowers is confirmed by the X-ray diffraction (XRD) pattern. As shown in Fig. S4,† the diffraction peaks at 2θ = 10.7° is indexed to the (002) peak of the MoS2, which appears a large shift to the left compared with the hexagonal-phase MoS2 (JCPDS card no. 37-1492), confirming that the interlayer spacing between the (002) planes are sufficiently expanded. Fig. 1d–f show the TEM and high resolution TEM (HRTEM) of the rosette-like MoS2 nanoflowers. The regular lattices in the SAED ring patterns (the inset of Fig. 1d) verify that the hexagonal-structured MoS2 is generated.40,41 Fig. 1e clearly reveals that typical stripe-like nanocrystals of layered MoS2 are formed. In addition, the interlayer spacing of the MoS2 nanoflowers is sufficiently increasing to 0.66 nm (Fig. 1f) which is larger than the spacing 0.61 nm of bulk MoS2, indicating the low ion diffusion resistance.42 Therefore, the aforementioned analysis confirms the formation of rosette-like MoS2 nanoflowers with interlayer distance can be easily fabricated by the one-step hydrothermal method.
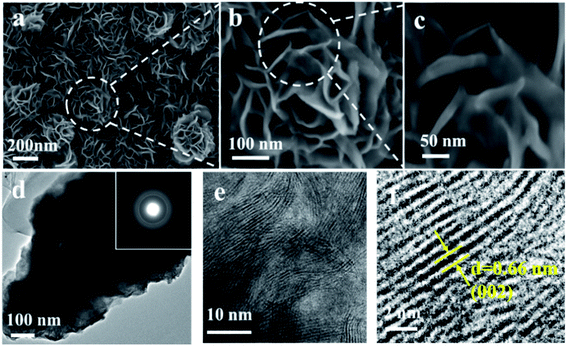 |
| Fig. 1 (a–c) SEM images of the rosette-like MoS2 nanoflowers; (d) TEM and (e and f) HRTEM images of a rosette-like MoS2 nanoflowers. The insets in (d) is the SAED pattern of the as-prepared samples. | |
To confirm the chemical composition of the as-prepared rosette-like MoS2 nanoflowers, X-ray photoelectron spectroscopy (XPS) was conducted (Fig. 2). The wide scan XPS spectrum (Fig. 2a) revealed five typical peaks corresponding to the binding energies of Mo 3d, Mo 3p, S 2p, C 1s and O 1s orbitals. The signals of the C and O originated from the CO2 and H2O impurities caused by surface adsorption and contamination.43 High-resolution Mo 3d XPS spectrum in Fig. 2b, the peaks at around 228.6 and 232 eV are corresponding to the 3d5/2 and 3d3/2 states, respectively. Besides these two peaks, the small peak located at 225.9 eV is indexed as S 2s. Similarly, in Fig. 2c, the peaks at 161.5 and 162.6 eV are assigned to the S 2p3/2 and S 2p1/2 states, respectively. The binding energies are in excellent agreement with the values for MoS2, confirm the formation of MoS2.44,45
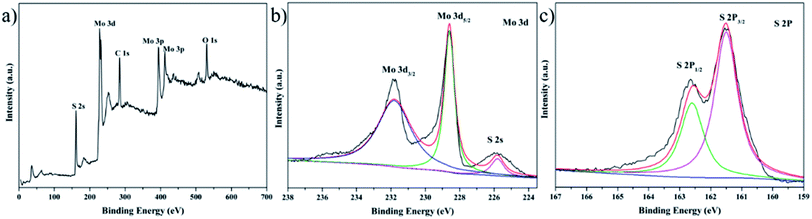 |
| Fig. 2 Structure characterization of the MoS2 for 12 h. (a) XPS survey spectrum of the rosette-like MoS2 nanoflower; (b) Mo 3d and (c) S 2p. | |
3.2 Electrochemical characterizations of rosette-like MoS2 nanoflowers
The electrocatalytic HER activities of the as-prepared rosette-like MoS2 nanoflowers catalysts were evaluated in acidic (0.5 M H2SO4) condition with a typical three-electrode configuration. The linear sweep voltammetry (LSV) curves of as-prepared catalysts and bare Ti foil are displayed in Fig. 3a. As expected, the rosette-like MoS2 nanoflowers catalyst with a growing time of 12 h exhibits excellent catalytic activity with a low overpotential of 0.25 V vs. RHE to drive a current density of 10 mA cm−2. For comparison, similar measurements on nanomaterials for 6 h and 20 h were also performed. The nanoflowers for 12 h exhibit much larger current density at the same potential than those of nanomaterials for 6 h and 20 h, suggesting that the exceptional HER electrocatalytic activity of the nanoflowers for 12 h. When compare with previous notable works of the MoS2 catalyst, the performance of the nanoflowers for 12 h is comparable (Table S1†). The excellent catalytic activity is attributed to the ‘petal effect’ of the rosette-like nanoflowers of the sample for 12 h, which can raise the adhesion of the sample to water.46,47 Also, the enlarged interlayer spacing of the rosette-like MoS2 nanoflowers could accelerate the transmission of the materials in the materials, which is profitable to enhance the HER activity of the sample for 12 h. In order to estimate the reaction kinetics and the rate-determining step for the HER process, the Tafel slope of the samples is plotted using the equation η = a + b
log(j), where a is the intercept, b is the Tafel slope and j is the current density.48 Based on the equation, the linear parts of the Tafel plots are shown in Fig. 3b, revealing that the Tafel slopes are about ∼67.5, 71.4 and 71.2 mV per decade for the three as-prepared nanomaterials with synthesis time of 6 h, 12 h and 20 h, respectively. The results indicate that the Volmer reaction is taking place and the hydrogen generation reaction follows a fast Volmer–Heyrovsky mechanism.49,50 Importantly, the low Tafel slope confirms the favorable HER kinetics,48 which is ascribed to the unique 3D hierarchical architectures that provide more pathways for ion transport.
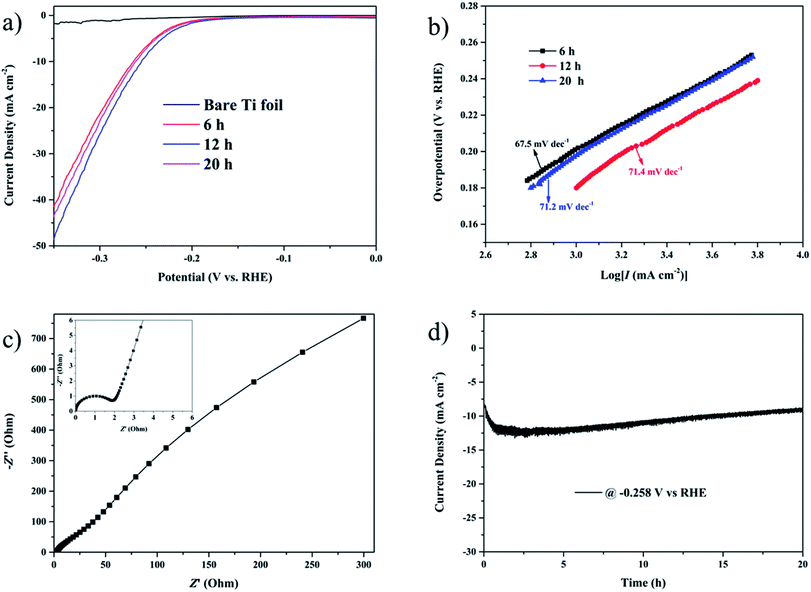 |
| Fig. 3 Electrochemical performance of catalysts for HER in 0.5 M H2SO4. (a) Linear sweep voltammetry (LSV) curves of bare Ti foil and the rosette-like MoS2 nanoflowers with different synthesis time; (b) the relevant Tafel curves derived from the polarization curves; (c) Nyquist plots of the catalyst for 12 h at open circuit potentials; (d) amperometric I–t curves of the catalyst for 12 h over 20 h. | |
The Nyquist plots can demonstrate the rate of the ion diffusion during HER, and the shorter the length of the Warburg-type line, the faster of the ion diffusion process from the outside electrolyte into the inner of the catalyst. To gain more insights into the roles of rosette-like structure in promoting the HER activity, the electrochemical impedance spectroscopy was explored. The charge transfer resistance (Rct) from the Fig. 3c coupled the contact resistance (Rs) from the inset in Fig. 3c demonstrate that rosette-like MoS2 nanoflowers exhibits superior HER kinetics due to the enhanced ion transport in the materials. Very importantly, the long-term electrochemical durability is another important criterion to evaluate the catalytic performance of the HER catalyst, and the stability of rosette-like MoS2 nanoflowers was examined at the current density of 10 mV cm−2 in acid condition (Movie 1, ESI†). As shown in Fig. 3d, the current density is stabilized after 20 h and only a very slight negative shift is observed in the polarization curve of the sample comparing with the initial polarization curve (Fig. S5, ESI†), indicating its superior durability for HER in acid condition. With regard to both activity and stability, the rosette-like MoS2 nanoflowers for 12 h represent as an outstanding HER electrocatalyst.
Besides utilization in HER, the as-prepared rosette-like MoS2 nanoflowers also plays an important role in energy storage. When used as an electrode material in supercapacitors, the electrochemical behavior of the rosette-like MoS2 nanoflowers is studied in 1.0 M aqueous H2SO4 with a potential interval from −0.2 to 0.4 V in a three-electrode system. Fig. 4a shows the CV curves at different scan rates, ranging from 10 to 800 mV s−1. Obviously, all the CV curves display an irregular rectangular shape with a little reduction peak, proving the presence of a double-layer capacitor (EDLC)51 along with a pseudocapacitive and irreversible faradaic reactions. In addition, the area under the CV curves increases with the increasing of scan rate,52 and the estimated area specific capacitance (CA) values in Fig. 4b based on the CV curves decrease steadily with the increased scan rate, which can be ascribed to the difficulty in ion transport at high scan rates.53 The CA value can achieve 71 mF cm−2 at the scan rate of 50 mV s−1, which is larger than the values of the samples for 6 and 20 h based on Fig. S6a.† This indicated that the as-prepared sample for 12 h has superior performance when compared with the samples for other hydrothermal time. Also, the shape of CV curves retains a good rectangular CV shape even at a higher scan rate of 800 mV s−1, which indicates a good electrochemical stability and rate performance of the sample. Furthermore, the typical capacitance enhancement at negative potentials indicates the easy charge/discharge of positively charges ions.54 Also, the galvanostatic charging–discharging (GCD) curves of the rosette-like MoS2 nanoflowers in Fig. 4c are performed in the potential window from −0.2 to 0.4 V to determine the capacitance dependence on different current density, ranging from 1 to 25 mA cm−2. And the quasi-triangular shapes can also indicate the mechanism for the EDLC-pseudocapacitance combined behavior of the supercapacitors. Based on eqn (2), the specific capacitances (CA) at the corresponding current densities are calculated and plotted in Fig. 4d. The CA of the sample is 290, 204, 163, 137 and 117 mF cm−2 at current densities of 1, 2, 5, 10 and 25 mA cm−2, respectively. The CA decreases with increasing the current density, which is due to that the ion transport is difficulty in nanostructured materials with large surface area at high current density. The CA of the samples for 6 and 20 h are only 120 and 174 mF cm−2 at current densities of 1 mA cm−2 calculated from the curves in Fig. S6b,† respectively, which can also demonstrate the superiority of the as-prepared samples for 12 h. In addition, the specific capacitance achieves a maximum value of 137 mF cm−2 at a low current density of 10 mA cm−2. This value is appreciable when compared with previously reported supercapacitors based on MoS2 materials (Table S2, ESI†). The high capacitance is attributed to the large surface area and the hierarchically layer structure of the sample, which provide shorter pathways for fast and efficient ion transport. Moreover, the specific capacitance kept on decreasing with the increase of current density, which is owning to the fact that almost all the available pores of the electrode are occupied by electrolyte ions at lower current densities.55 To validate its practical application, the cyclic stability of samples is examined by CV measurement at 50 mV s−1 in a potential window between −0.4 and 0.2 V. As shown in Fig. 4e, the specific capacitance of the sample continues to decrease between 3000 and 4000 cycles, and then stabilize constant. After 10
000 cycles, the specific capacitance remains a high capacitance retention of 81.6% after 10
000 cycles. The morphological properties of the samples after 3000 and 4000 cyclability are analyzed by SEM, and the images of the samples are displayed in Fig. S7.† From Fig. S7a,† the samples are still maintaining the original morphology of rosette-like nanoflowers after 3000 cycles, but the nanoflowers are covered by the substances after 4000 cycles (Fig. S7b†). So, the active site of the samples reduces after 3000 cycles, which results that the capacity continue to decrease between 3000 and 4000 cycles. After 3000 cycles, the pores are generated on the samples (Fig. S8†), which is beneficial to the transport of the electrons53 and enhance the performance of the electrode material. Therefore, the capacity stabilizes after 4000 cycles. Fig. 4f shows the electrochemical impedance spectroscopy (EIS) curve of the MoS2 nanoflowers from 0.01 Hz to 10 kHz (inset shows the equivalent circuit and the fitted equivalent circuit), which indicates an excellent EDLC behavior of the device and a small equivalent series resistance facilitating electrostatic adsorption of ions.56 Obviously, such MoS2 nanomaterial presents great potential in energy storage applications.
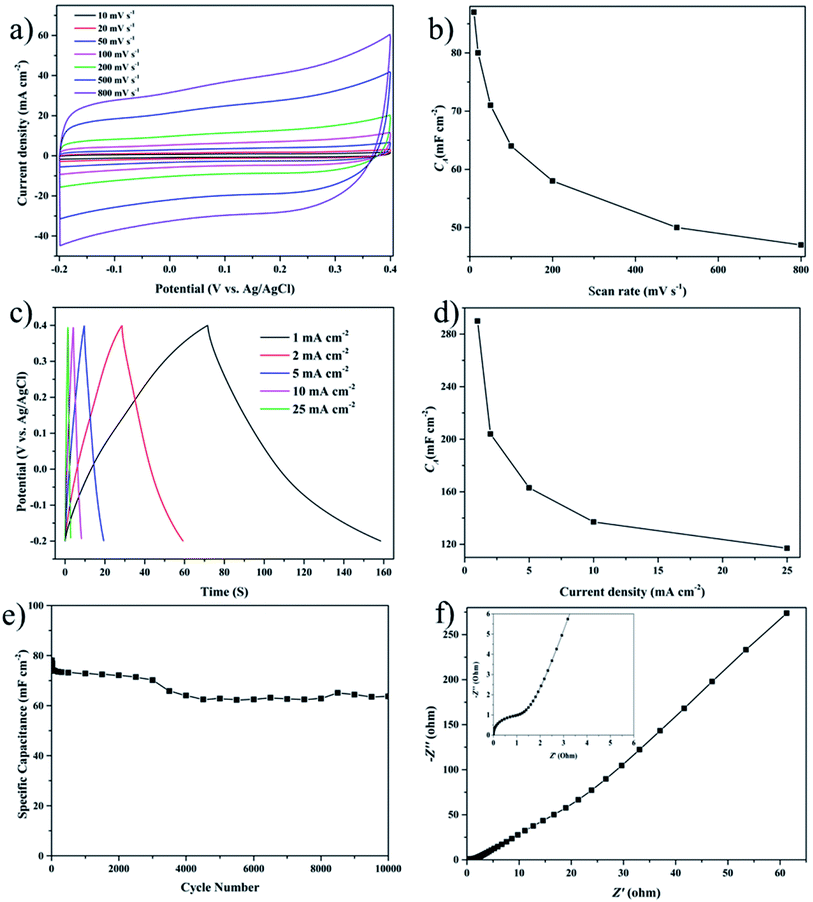 |
| Fig. 4 Electrochemical energy storage performance of the rosette-like MoS2 nanoflowers. (a) CV curves at different scan rates from 10 to 800 mV s−1; (b) corresponding specific capacitances at different scan rates; (c) galvanostatic charging–discharging (GCD) curves at current densities of 1, 2, 5, 10, and 25 mA cm−2; (d) the variation of capacitance at different current densities; (e) cycle stability analysis at a scan rate of 50 mV s−1; (f) Nyquist plots in the frequency range from 0.01 Hz to 100 kHz. | |
4. Conclusions
In summary, the rosette-like MoS2 nanoflowers on Ti foil have been directly synthesized for the first time via a simple hydrothermal reaction. The as-prepared materials obtained by optimizing the synthesis time delivered excellent electrochemical performance towards the HER and supercapacitor. This is attributed to the unique 3D architectures and the enlarged interlayer spacing of the rosette-like MoS2 nanoflowers, which is beneficial to the transport of ions and electrolyte. This work provided a new way to develop well-designed nanostructured materials for both efficient energy production and storage.
Conflicts of interest
There are no conflicts to declare.
Acknowledgements
This work was supported by the National Natural Science Foundation of China (21764007, 21662018), the Natural Science Foundation of the Jiangxi Province of China (20161BAB203082) and Jiangxi Provincial Department of Education's Item of Science and Technology (GJJ160759, GJJ170653 and GJJ150760).
References
- G. Gershinsky, E. Bar, L. Monconduit and D. Zitoun, Operando electron magnetic measurements of Li-ion batteries, Energy Environ. Sci., 2014, 7, 2012–2016 RSC.
- Q. Jiang, N. Kurra, M. Alhabeb, Y. Gogotsi and H. N. Alshareef, All pseudocapacitive MXene-RuO2 asymmetric supercapacitor, Adv. Energy Mater., 2018, 7, 1703043 CrossRef.
- R. R. Salunkhe, Y. V. Kaneti and Y. Yamauchi, Metal-organic framework-derived nanoporous metal oxides toward supercapacitor applications: progress and prospects, ACS Nano, 2017, 11, 5293–5308 CrossRef CAS PubMed.
- Y. Y. Feng, H. J. Zhang, L. Fang, Y. P. Mu and Y. Wang, Uniquely Monodispersing NiFe Alloyed Nanoparticles in Three-Dimensional Strongly Linked Sandwiched Graphitized Carbon Sheets for High-Efficiency Oxygen Evolution Reaction, ACS Catal., 2016, 6, 4477–4485 CrossRef CAS.
- Y. Jiao, Y. Zheng, K. Davey and S. Z. Qiao, Activity origin and catalyst design principles for electrocatalytic hydrogen evolution on heteroatom-doped graphene, Nat. Energy, 2016, 1, 16130 CrossRef CAS.
- D. Y. Wang, M. Gong, H. L. Chou, C. J. Pan, H. A. Chen, Y. P. Wu, M. C. Lin, M. Y. Guang, J. Yang, C. W. Chen, Y. L. Wang, B. J. Hwang, C. C. Chen and H. J. Dai, Highly Active and Stable Hybrid Catalyst of Cobalt-Doped FeS2 Nanosheets-Carbo Nanotubes for Hydrogen Evolution Reaction, J. Am. Chem. Soc., 2015, 137, 1587–1592 CrossRef CAS PubMed.
- Y. G. Wang, Y. F. Song and Y. Y. Xia, Electrochemical capacitors: mechanism, materials, systems, characterization and applications, Chem. Soc. Rev., 2016, 45, 5925–5950 RSC.
- F. C. Zheng, Y. Yang and Q. W. Chen, High lithium anodic performance of highly nitrogen-doped porous carbon prepared from a metal-organic framework, Nat. Commun., 2014, 5261 CrossRef CAS PubMed.
- Z. X. Wu, W. Lei, J. Wang, R. Liu, K. D. Xia, C. J. Xuan and D. L. Wang, Various structured molybdenum-based nanomaterials as advanced anode materials for lithium ion batteries, ACS Appl. Mater. Interfaces, 2017, 9, 12366–12372 CrossRef CAS PubMed.
- H. F. Lv, Z. Xi, Z. Z. Chen, S. J. Guo and Y. S. Yu, A New Core/Shell NiAu/Au Nanoparticle Catalyst with Pt-like Activity for Hydrogen Evolution Reaction, J. Am. Chem. Soc., 2015, 137, 5859–5862 CrossRef CAS PubMed.
- Y. Y. Feng, H. J. Zhang, Y. X. Guan, Y. P. Mu and Y. Wang, Novel three-dimensional flower-like porous Al2O3 nanosheets anchoring NiS2 nanoparticles for high-efficiency hydrogen evolution, J. Mater. Chem. A, 2016, 4, 11507 RSC.
- Y. Jiao, Y. Zheng, M. Jaronies and S. Z. Qiao, Design of electrocatalysts for oxygen- and hydrogen-involving energy conversion reactions, Chem. Soc. Rev., 2015, 44, 2060–2086 RSC.
- G. Li, X. L. Wang, J. Fu, J. D. Li, M. G. Park, Y. N. Zhang, G. Lui and Z. W. Chen, Pomegranate-Inspired Design of Highly Active and Durable Bifunctional Electrocatalysts for Rechargeable Metal-Air Batteries, Angew. Chem., Int. Ed., 2016, 55, 4977–4982 CrossRef CAS PubMed.
- D. Voiry, J. Yang and M. Chhowalla, Recent strategies for improving the catalytic activity of 2D TMD nanosheets toward the hydrogen evolution reaction, Adv. Mater., 2016, 28, 6197–6206 CrossRef CAS PubMed.
- L. L. Feng, G. T. Yu, Y. Y. Wu, G. Li, Y. H. Sun, T. Asefa, W. Chen and X. X. Zou, High-Index Faceted Ni3S2 Nanosheet Arrays as Highly Active and Ultrastable Electrocatalysts for Water Splitting, J. Am. Chem. Soc., 2015, 137, 14023–14026 CrossRef CAS PubMed.
- E. J. Popczun, C. G. Read, C. W. Roske, N. S. Lewis and R. E. Schaak, Highly Active Electrocatalysis of the Hydrogen Evolution Reaction
by Cobalt Phosphide Nanoparticles, Angew. Chem., Int. Ed., 2014, 53, 5427–5430 CrossRef CAS PubMed.
- M. S. Faber and S. Jin, Earth-abundant inorganic electrocatalysts and their nanostructures for energy conversion applications, Energy Environ. Sci., 2014, 7, 3519–3542 RSC.
- M. S. Faber, R. Dziedzic, M. A. Lukowski, N. S. Kaiser, Q. Ding and S. Jin, High-Performance Electrocatalysis Using Metallic Cobalt Pyrite (CoS2) Micro- and Nanostructures, J. Am. Chem. Soc., 2014, 136, 10053–10061 CrossRef CAS PubMed.
- W. F. Chen, K. Sasaki, C. Ma, A. I. Frenkel, N. Marinkovic, J. T. Muckerman, Y. M. Zhu and R. R. Adzic, Hydrogen-Evolution Catalysts Based on Non-Noble Metal Nickel–Molybdenum Nitride Nanosheets, Angew. Chem., Int. Ed., 2012, 51, 6131–6135 CrossRef CAS PubMed.
- H. Vrubel and X. L. Hu, Molybdenum Boride and Carbide Catalyze Hydrogen Evolution in both Acidic and Basic Solutions, Angew. Chem., Int. Ed., 2012, 51, 12703–12706 CrossRef CAS PubMed.
- E. J. Popczun, J. R. McKone, C. G. Read, A. J. Biacchi, A. M. Wiltrout, N. S. Lewis and R. E. Schaak, Nanostructured Nickel Phosphide as an Electrocatalyst for the Hydrogen Evolution Reaction, J. Am. Chem. Soc., 2013, 135, 9267–9270 CrossRef CAS PubMed.
- J. F. Xie, H. Zhang, S. Li, R. X. Wang, X. Sun, M. Zhou, J. F. Zhou, X. W. Lou and Y. Xie, Defect-Rich MoS2 ultrathin nanosheets with additional active edge sites for enhanced electrocatalytic hydrogen evolution, Adv. Mater., 2013, 25, 5807–5813 CrossRef CAS PubMed.
- Z. Y. Lu, H. C. Zhang, W. Zhu, X. Y. Yu, Y. Kuang, Z. Chang, X. D. Lei and X. M. Sun, In situ fabrication of porous MoS2 thin-films as high-performance catalysts for electrochemical hydrogen evolution, Chem. Commun., 2013, 49, 7516–7518 RSC.
- J. Kibsgaard, Z. B. Chen, B. N. Reinecke and T. F. Jaramillo, Engineering the surface structure of MoS2 to preferentially expose active edge sites for electrocatalysis, Nat. Mater., 2012, 11, 963–969 CrossRef CAS PubMed.
- Z. B. Chen, D. Cummins, B. N. Reinecke, E. Clark, M. K. Sunkara and T. F. Jaramillo, Core–shell MoO3-MoS2 nanowires for hydrogen evolution: a functional design for electrocatalytic materials, Nano Lett., 2011, 11, 4168–4175 CrossRef CAS PubMed.
- J. D. Benck, Z. B. Chen, L. Y. Kuritzky, A. J. Forman and T. F. Jaramillo, Amorphous molybdenum sulfide catalysts for electrochemical hydrogen production: Insights into the origin of their catalytic activity, ACS Catal., 2012, 2, 1916–1923 CrossRef CAS.
- H. T. Wang, C. Tsai, D. S. Kong, K. Chan, F. Abild-Pedersen, J. K. Nørskov and Y. Cui, Transition-metal doped edge sites in vertically aligned MoS2 catalysts for enhanced hydrogen evolution, Nano Res., 2015, 8, 566–575 CrossRef CAS.
- M. Khan, A. B. Yousaf, M. M. Chen, C. S. Wei, X. B. Wu, N. D. Huang, Z. M. Qi and L. B. Li, Molybdenum sulfide/graphene-carbon nanotube nanocomposite material for electrocatalytic applications in hydrogen evolution reactions, Nano Res., 2016, 9, 837–848 CrossRef CAS.
- Z. X. Wu, J. Wang, R. Liu, K. D. Xia, C. J. Xuan, J. P. Guo, W. Lei and D. L. Wang, Facile preparation of carbon sphere supported molybdenum compounds (P, C and S) as hydrogen evolution electrocatalysts in acid and alkaline electrolytes, Nano Energy, 2017, 32, 511–519 CrossRef CAS.
- Z. X. Wu, J. Wang, K. D. Xia, W. Lei, X. Liu and D. L. Wang, MoS2-MoP heterostructured nanosheets on polymer-derived carbon as an electrocatalyst for hydrogen evolution reaction, J. Mater. Chem. A, 2018, 6, 616–622 RSC.
- G. Wang, J. Y. Tao, Y. J. Zhang, S. P. Wang, X. J. Yan, C. C. Liu, F. Hu, Z. Y. He, Z. J. Zuo and X. W. Yang, Engineering Two-Dimensional Mass-Transport Channels of the MoS2 Nanocatalyst toward Improved Hydrogen Evolution Performance, ACS Appl. Mater. Interfaces, 2018, 10, 25409–25414 CrossRef CAS PubMed.
- J. Deng, H. B. Li, J. P. Xiao, Y. C. Tu, D. H. Deng, H. X. Yang, H. F. Tian, J. Q. Li, P. J. Ren and X. H. Bao, Triggering the electrocatalytic hydrogen evolution activity of the inert two-dimensional MoS2 surface via single-atom metal doping, Energy Environ. Sci., 2015, 8, 1594–1601 RSC.
- J. Kibsgaard, Z. B. Chen, B. N. Reinecke and T. F. Jaramillo, Engineering the surface structure of MoS2 to preferentially expose active edge sites for electrocatalysis, Nat. Mater., 2012, 11, 963–969 CrossRef CAS PubMed.
- M. Saraf, K. Natarajan, A. K. Saini and S. M. Mobin, Small biomolecule sensors based on an innovative MoS2-rGO heterostructure modified electrode platform: a binder-free approach, Dalton Trans., 2017, 46, 15848–15858 RSC.
- F. P. Wang, G. F. Li, J. F. Zheng, J. Ma, C. X. Yang and Q. Z. Wang, Hydrothermal synthesis of flower-like molybdenum disulfide microspheres and their application in electrochemical supercapacitors, RSC Adv., 2018, 8, 38945–38954 RSC.
- Z. Y. Ma, X. F. Zhou, W. Deng, D. Lei and Z. P. Liu, 3D Porous MXene (Ti3C2)/Reduced Graphene Oxide Hybrid Films for Advanced Lithium Storage, ACS Appl. Mater. Interfaces, 2018, 10, 3634–3643 CrossRef CAS PubMed.
- Y. Zhang, T. He, G. L. Liu, L. H. Zu and J. H. Yang, One-pot mass preparation of MoS2/C aerogels for high-performance supercapacitors and lithium-ion batteries, Nanoscale, 2017, 9, 10059–10066 RSC.
- G. L. Liu, Z. Y. Wang, L. H. Zu, Y. Zhang, Y. T. Feng, S. H. Yang, Y. Jia, S. Y. Wang, C. Zhang and J. H. Yang, Hydrogen evolution reactions boosted by bridge bonds between electrocatalysts and electrodes, Nanoscale, 2018, 10, 4068–4076 RSC.
- X. Q. Xie, T. Makaryan, M. Q. Zhao, K. L. Van Aken, Y. Gogotsi and G. X. Wang, MoS2 Nanosheets Vertically Aligned on Carbon Paper: A Freestanding Electrode for Highly Reversible Sodium-Ion Batteries, Adv. Energy Mater., 2016, 6, 1502161 CrossRef.
- B. Ahmeda, D. H. Anjuma, Y. Gogotsi and H. N. Alshareef, Atomic layer deposition of SnO2 on MXene for Li-ion battery anodes, Nano Energy, 2017, 34, 249–256 CrossRef.
- K. Ma, H. Jiang, Y. J. Hu and C. Z. Li, 2D Nanospace Confined Synthesis of Pseudocapacitance-Dominated MoS2-in-Ti3C2 Superstructure for Ultrafast, Adv. Funct. Mater., 2018, 28, 1804306 CrossRef.
- C. T. Zhao, C. Yu, M. D. Zhang, Q. Sun and S. F. Li, Enhanced sodium storage capability enabled by super wide-interlayer-spacing MoS2 integrated on carbon fibers, Nano Energy, 2017, 41, 66–74 CrossRef CAS.
- Z. Pu, Q. Liu, A. M. Asiri, Y. Luo, X. Sun and Y. He, 3D microporous MoS2 thin film: in situ hydrothermal preparation and application as a highly active hydrogen evolution electrocatalyst at all pH values, Electrochim. Acta, 2015, 168, 133–138 CrossRef CAS.
- Y. H. Chang, C. T. Lin, T. Y. Chen, C. L. Hsu, Y. H. Lee, W. Zhang, K. H. Wei and L. J. Li, Highly Efficient electrocatalytic hydrogen production by MoSx grown on graphene-protected 3D Ni foams, Adv. Mater., 2013, 25, 756–760 CrossRef CAS PubMed.
- K. K. Liu, W. Zhang, Y. H. Lee, Y. C. Lin, M. T. Chang, C. Y. Su, H. Li, Y. Shi, H. Zhang, C. S. Lai and L. J. Li, Growth of large-area and highly crystalline MoS2 thin layers on insulating substrates, Nano Lett., 2012, 12, 1538–1544 CrossRef CAS PubMed.
- J. L. Yong, F. Chen, Y. Fang, J. L. Huo, Q. Yang, J. Z. Zhang, H. Bian and X. Hou, Bioinspired Design of Underwater Superaerophobic and Superaerophilic Surfaces by Femtosecond Laser Ablation for Anti- or Capturing Bubbles, ACS Appl. Mater. Interfaces, 2017, 9, 39863–39871 CrossRef CAS PubMed.
- C. Huang and Z. G. Guo, The wettability of gas bubbles: from macro behavior to nano structures to applications, Nanoscale, 2018, 10, 19659–19672 RSC.
- J. L. Huang, D. M. Hou, Y. C. Zhou, W. J. Zhou, G. Q. Li, Z. H. Tang, L. G. Li and S. W. Chen, MoS2 nanosheet-coated CoS2 nanowire arrays on carbon cloth as three-dimensional electrodes for efficient electrocatalytic hydrogen evolution, J. Mater. Chem. A, 2015, 3, 22886–22891 RSC.
- Y. G. Li, H. L. Wang, L. M. Xie, Y. Y. Liang, G. S. Hong and H. J. Dai, MoS2 Nanoparticles Grown on Graphene: An Advanced Catalyst for the Hydrogen Evolution Reaction, J. Am. Chem. Soc., 2011, 133, 7296–7299 CrossRef CAS PubMed.
- F. X. Ma, H. B. Wu, B. Y. Xia, C. Y. Xu and X. W. Lou, Hierarchical β-Mo2C Nanotubes Organized by Ultrathin Nanosheets as a Highly Efficient Electrocatalyst for Hydrogen Production, Angew. Chem., Int. Ed., 2015, 54, 15395–15399 CrossRef CAS PubMed.
- M. Saraf, R. A. Dar, K. Natarajan, A. K. Srivastava and S. M. Mobin, A Binder-Free hybrid of CuO-Microspheres and rGO Nanosheets as an Alternative Material for Nest Generation Energy Storage Application, ChemistrySelect, 2016, 1, 2826–2833 CrossRef CAS.
- R. Zhou, C. J. Han and X. M. Wang, Hierarchical MoS2-coated three-dimensional graphene network for enhanced supercapacitor performances, J. Power Sources, 2017, 352, 99–110 CrossRef CAS.
- Y. Yang, H. L. Fei, G. D. Ruan, C. S. Xiang and M. T. James, Edge-oriented MoS2 Nanoporous films as Flexible Electrodes for Hydrogen Evolution Reactions and Supercapacitor Devices, Adv. Mater., 2014, 26, 8163–8168 CrossRef CAS PubMed.
- M. S. Raghua, K. Y. Kumar, S. Rao, T. Aravinda, S. C. Sharma and M. K. Prashanth, Simple fabrication of reduced graphene oxide-few layer MoS2 nanocomposite for enhanced electrochemical performance in supercapacitors and water purification, Phys. B, 2018, 537, 336–345 CrossRef.
- G. Zhang, H. J. Liu, J. H. Qu and J. H. Li, Two-dimensional layered MoS2: rational design, properties and electrochemical applications, Energy Environ. Sci., 2016, 9, 1190–1209 RSC.
- S. Mohit, N. Kaushik and M. M. Shaikh, Emerging Robust Heterostructure of MoS2-rGO for High-Performance Supercapacitors, ACS Appl. Mater. Interfaces, 2018, 10, 16588–16595 CrossRef PubMed.
Footnote |
† Electronic supplementary information (ESI) available. See DOI: 10.1039/c9ra01111k |
|
This journal is © The Royal Society of Chemistry 2019 |
Click here to see how this site uses Cookies. View our privacy policy here.