DOI:
10.1039/C9RA01509D
(Paper)
RSC Adv., 2019,
9, 15061-15072
Preparation, characterization, and use of novel Cu@Fe3O4 MNPs in the synthesis of tetrahydrobenzimidazo[2,1-b]quinazolin-1(2H)-ones and 2H-indazolo[2,1-b]phthalazine-triones under solvent-free conditions†
Received
27th February 2019
, Accepted 7th May 2019
First published on 14th May 2019
Abstract
Cu@Fe3O4 MNPs as novel nanomagnetic reagents were prepared to investigate their catalytic behavior in the preparation of tetrahydrobenzimidazo[2,1-b]quinazolin-1(2H)-ones, as important biologically active compounds. Then, characterization of the synthesized nanoparticles was performed using different methods including Fourier transform infrared spectroscopy (FT-IR), X-ray diffraction (XRD) analysis, thermogravimetric analysis (TGA), vibrating sample magnetometer (VSM), energy dispersive X-ray (EDX), and scanning electron microscopy (SEM). All reactions were performed with small amounts of the Cu@Fe3O4 MNPs under solvent-free conditions. After completion of the reaction, because of the magnetic nature of the nanocatalyst, they could be simply separated with an external magnet and easily reused with no considerable decrease in the catalytic behavior even after seven runs.
Introduction
The use of eco-friendly, efficient, and maintainable reusable heterogeneous magnetic nanocatalysts involves both economic and ecological advantages.1 Coating of iron oxides with silica to form core–shell structures is considered a good semi-heterogeneous catalyst owing to the existence of surface FeOH groups. These groups in magnetic nanoparticles, as beneficial groups for the immobilization of homogeneous catalysts, are among the main issues in organic transformations. Among the noticeable characteristics of these nanoparticles are their high specific surface area and easy separation from the solution using an appropriate permanent magnet compared with centrifugation or filtration, which are not convenient for small magnetic nanoparticles.2–8
Heterocyclic compounds have attracted extensive attention in the field of chemical research because of their striking structural features, clinical applications, and pharmacological properties. Recently, the preparation of quinazolinone derivatives has been of extensive interest because of their biological and pharmaceutical activities such as antihypertensive, antihistaminic, analgesic and anti-inflammatory, anticancer, and anti-HIV.9–13 In chemistry, compounds with the quinazolinone skeleton such as tetrahydrobenzimidazo[2,1-b]quinazolin-1(2H)-ones have been widely utilized as building blocks in plenty of natural and synthetic products. In order to synthesize tetrahydrobenzimidazo[2,1-b]quinazolin-1(2H)-ones, a variety of strategies have been developed in the recent studies. However, these techniques suffer from some drawbacks such as long reaction times, requiring a large amount of the catalyst, and difficulty in catalyst isolation from the solution.14–18 Therefore, the search for improving reaction conditions for the preparation of these kinds of heterocyclic molecules via the three-component reaction between 2-aminobenzimidazole, aldehyde, and 5,5-dimethyl-1,3-cyclohexanedione using effective and recoverable catalysts under green conditions is a prime and real challenge for synthetic chemists.
The molecules containing phthalhydrazide moiety such as 2H-indazolo[2,1-b]phthalazine-triones has wide pharmaceutical and biological activities, such as anticonvulsant, cardiotonic, antifungal, anticancer, and vasorelaxant properties.19–23 Diverse types of method and catalyst have been developed for the acceleration of the production of phthalazine derivatives; however, several of these procedures suffer from some limitations.24–30 Hence, it is essential to expand an improved path for the effectual synthesis of these compounds under mild reaction conditions.
Experimental
General
All the pure substances were prepared from Merck, Aldrich, and Fluka chemical companies. Melting points of the substrate were determined using an Electrothermal-9100 apparatus with no correction. FT-IR spectroscopy was performed using a PerkinElmer PXI spectrometer in KBr wafers. TGA spectra were obtained using a TGA thermoanalyzer (PerkinElmer) instrument. The chemical composition was determined by means of energy dispersive X-ray spectroscopy (EDX) (ESEM, Philips, and XL30). The X-ray diffraction (XRD) measurements of catalyst were carried out with a Siemens D-500 X-ray diffractometer (Munich, Germany). Scanning electron microscopy was performed using an SEM-LEO 1430VP analyzer. Magnetic susceptibility measurements were accomplished using vibrating sample magnetometry (VSM; Lake Shore 7200 at 300 K VSM).
Catalyst synthesis
Preparation of Fe3O4. Magnetic nanoparticles Fe3O4 were synthesized in accordance with the reported chemical co-precipitating Fe2+ and Fe3+ ions in the existence of ammonia solution. For this purpose, 2 g of FeCl2·4H2O and 5.4 g of FeCl3·6H2O were dissolved in 40 mL deoxygenated water containing 0.9 mL of concentrated HCl under a continuous flow of nitrogen gas. Black sediment was achieved by addition of 250 mL of NaOH solution into Fe2+ and Fe3+ solution at ambient temperature under agitation. After this time, the precipitate was magnetically isolated and washed several times with water and ethanol. Finally, the precipitate was dried at 40 °C for 24 h and stored in glass vials.
Preparation of Fe3O4@SiO2. About 1 g of the Fe3O4 was dispersed in a mixture of EtOH (40 mL) and deionized water (5 mL) by ultrasonication for 20 min, followed by the addition of 3 mL of aqueous ammonia (28 wt%) and 0.5 mL of triethoxysilane (TEOS). The obtained mixture was mechanically stirred at 400 rpm for 24 h at 25 °C. After completion of the reaction, the precipitates of core–shell Fe3O4@SiO2 nanoparticles were collected by magnetic separation, washed several times with diluted hydrochloric acid, and deionized water, and dried under vacuum oven at 70 °C for 5 h.
Preparation of Fe3O4 bonded propyl chloride (Fe3O4@PC). About, 1 g of Fe3O4@SiO2 nanoparticles were suspended in 20 mL dry toluene and sonicated for 20 min in an ultrasonic bath. Then, 2 mL of 3-chloropropyltriethoxysilane (CPTCSi) was added into the flask and the reaction mixture was refluxed and mechanically agitated for 24 h under nitrogen atmosphere. At the end of the reaction, the suspension was collected using a powerful magnet and washed several times with ethanol and distilled water to remove any unreacted chemicals, and dried under vacuum oven.
Preparation of MNPs bonded dicyandiamide (Fe3O4@Gu). The MNPs@PC (2 g) was dispersed in 50 mL deionized water using ultrasonication for half an hour. Then, dicyandiamide (2 mmol) and triethylamine (1 mL) was poured in to the reaction vessel and refluxed for 2 h under a nitrogen atmosphere. After this step, the resultant solid (MNPs@Gu) was isolated by a permanent magnet and rinsed with water and ethanol, and then dried under a vacuum oven.
Preparation of Fe3O4@Gu@TSH. First, 2 g of prepared MNPs@Gu was dissolved in 50 mL deionized water followed by a 30 min sonication. Then, 50 mL of thiosalicylhydrazide (0.05 M) along with 50 mL hydrochloric acid (0.15 M) was poured in to the reaction vessel and sonicated for 1 h. In the following reaction, the product was stirred with sodium hydroxide (10%) to neutralize the excess hydrochloric acid present in the reaction vessel. Lastly, the synthesized Fe3O4@Gu@TSH was magnetically recycled and rinsed with ethanol several times and then dried in a vacuum oven.
Coordination of Cu(II) with Fe3O4@Gu@TSH. The Fe3O4@Gu@TSH (1 g) was distributed in 20 mL of ethanol by the ultrasonic bath for half an hour. Subsequently, CuCl2 (2 g) was poured in to the dispersion of Fe3O4@Gu@TSH and the mixture was stirred for 24 hours at ambient temperature. Upon completing the reaction, the resultant precipitate Cu@Fe3O4 MNPs formed was separated by magnetic decantation and washed twice with ethanol (15 mL) to remove unattached metal precursors and dried under vacuum oven to afford the pure product. All stages of the Cu@Fe3O4 MNPs synthesis are presented in Scheme 1.
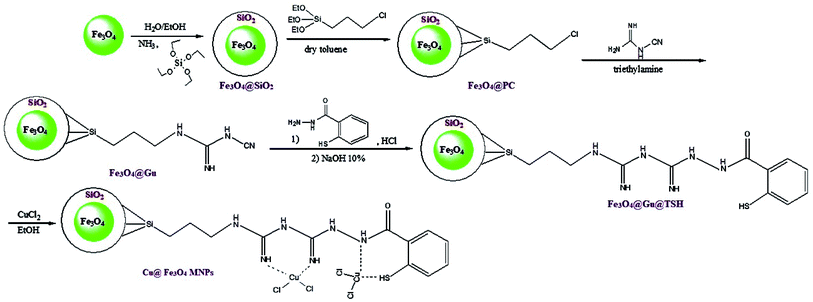 |
| Scheme 1 All stages of the Cu@Fe3O4 MNPs synthesis. | |
General process for the synthesis of tetrahydrobenzimidazo[2,1-b]quinazolin-1(2H)-ones (4)
A mixture of 2-aminobenzimidazole (1 mmol), aldehyde (1 mmol), dimedone (1 mmol), and Cu@Fe3O4 MNPs (0.38 mol%) was combined with each other at 100 °C in an oil bath under solvent-free conditions. The development of the reaction was checked using TLC [n-hexane
:
ethyl acetate (7
:
4 ratio)] analyses. At the end of the reaction, the mixture was dissolved in a hot mixture of ethyl acetate and ethanol (4
:
10 ratio) and then the catalyst was removed using an appropriate magnet. The remaining mixture was cooled down to ambient temperature and the pure product was separated by filtration. Finally, the Cu@Fe3O4 MNPs were washed with chloroform, dried, and used directly with an insignificant decrease in its activity for at least five runs.
General process for the synthesis of 2H-indazolo[2,1-b]phthalazine-triones (6)
A mixture of phthalhydrazide (1 mmol), aldehyde (1 mmol), dimedone (1 mmol), and Cu@Fe3O4 MNPs (0.38 mol%) was combined with each other at 100 °C in an oil bath under solvent-free conditions. The progress of the reaction was monitored by TLC [n-hexane
:
ethyl acetate (7
:
4 ratio)] analyses. After completion of the reaction, the mixture was dissolved in a hot mixture of ethyl acetate and ethanol (4
:
10 ratio) and then the catalyst was removed by an external magnet. The remaining mixture was cooled to room temperature and the pure product was separated by filtration. Finally, the Cu@Fe3O4 MNPs were washed with chloroform, dried, and used directly with a negligible reduction of its activity for at least five runs.
Results and discussion
Catalyst characterization
FTIR analysis of Cu@MNPs. The FT-IR spectra corresponding to the Fe3O4, Fe3O4@SiO2, Fe3O4@PC, Fe3O4@Gu@TSH, Cu@Fe3O4 MNPs and recovered Cu@Fe3O4 MNPs are showed in Fig. 1. The FT-IR spectrum of the Fe3O4 showed characteristic absorption at 579 and 3395 cm−1 due to Fe–O–Fe and O–H stretching vibrations, respectively. In the spectrum of Fe3O4@SiO2, the associated absorption bands of the Si–O–Si and Si–OH stretching vibrations were observed at 957 cm−1 and 1042 cm−1, respectively. The FT-IR spectrum of the Fe3O4@PC exhibits a peak at 2982 cm−1 that is indexed to the C–H stretching vibration mode. In Fe3O4@Gu@TSH and Cu@Fe3O4 MNPs, the absorption peaks at 2573 cm−1 and 3315 cm−1 are indexed to the S–H and N–H stretching vibrations, respectively. Also, the peak at 1638 cm−1 is related with the
NH and –NH stretching vibrations of the functional groups on the surface of the Fe3O4. It is necessary to mention that the spectrum of recovered Cu@Fe3O4 MNPs after the first recovery and reuse do not show any difference.
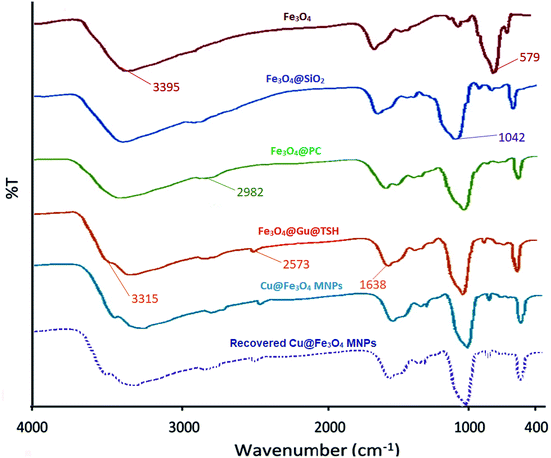 |
| Fig. 1 FTIR spectra corresponding to Fe3O4, Fe3O4@SiO2, Fe3O4@PC, Fe3O4@Gu@TSH, and Cu@Fe3O4 MNPs. | |
Thermal analysis of Cu@MNPs. Thermogravimetric analysis (TGA) spectrum of Fe3O4, Fe3O4@PC, and Cu@Fe3O4 MNPs is presented in Fig. 2. TGA data for Fe3O4 and Fe3O4@PC samples show that approximately 2% and 3.5% mass loss at a temperature up to 600 °C is because of losing water molecules. The TGA curve of Cu@Fe3O4 MNPs shows two distinct steps of mass loss. The early mass loss of the Cu@Fe3O4 MNPs was less than about 200 °C, which is due to evaporation of adsorbed water. The second significant mass loss is at temperatures within the range of 370–420 °C, which can be related to the organic parts decomposition.
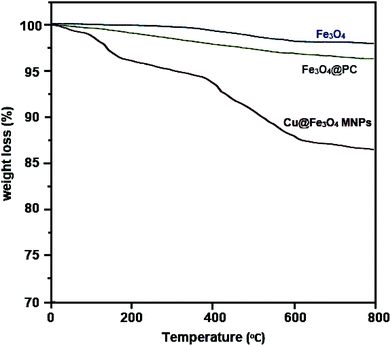 |
| Fig. 2 TGA curves of Fe3O4, Fe3O4@PC, and Cu@Fe3O4 MNPs. | |
VSM analysis of Cu@MNPs. To describe the magnetic feature of the catalyst, magnetic measurements of the Fe3O4 and Cu@Fe3O4 MNPs were surveyed with a vibrating sample magnetometer (VSM) at ambient temperature with the field sweeping from −8500 to +8500 oersted (Fig. 3). The saturation magnetization (Ms) value of Fe3O4 was about 60.39 emu g−1, which decreased to 33.85 emu g−1 because of the creation of a nonmagnetic silica shell and organic groups around the Fe3O4 core.
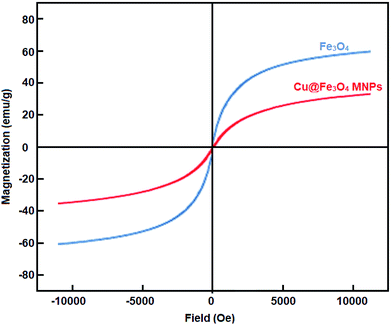 |
| Fig. 3 VSM magnetization curves corresponding to the bare Fe3O4 and Cu@Fe3O4 MNPs. | |
SEM analysis of Cu@MNPs. A morphological property of Cu@Fe3O4 MNPs was evaluated using SEM as shown in Fig. 4. The size of magnetite nanocatalyst was in the range 50–65 nm based on SEM measurements and has more porous surface. It illustrates that the incorporation of magnetic nanoparticles with organic groups increases surface porosity.
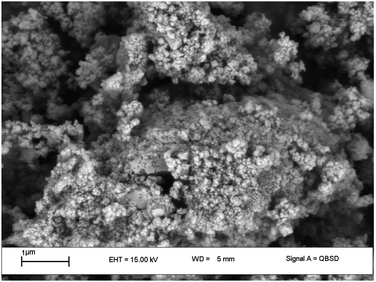 |
| Fig. 4 SEM spectra of Cu@Fe3O4 MNPs. | |
EDX analysis of Cu@MNPs. The energy dispersive X-ray analysis (EDX) spectrum of Cu@Fe3O4 MNPs illustrates the presence of all of the expected elements (Fe, O, Si, C, N, and Cu) and confirms immobilization of copper(II) complex on the Fe3O4 surface (Fig. 5).
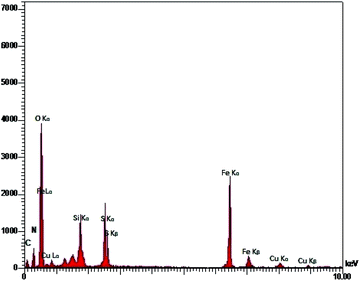 |
| Fig. 5 EDX spectra of Cu@Fe3O4 MNPs. | |
XRD analysis of Cu@MNPs. The energy dispersive X-ray analysis (EDX) spectrum of the Fe3O4, Fe3O4@Gu@TSH and Cu@Fe3O4 MNPs (Fig. 6) had six characteristic peaks at 2θ = 30.29, 35.34, 43.73, 54.45, 57.51, 63.19, and 74.58 were indexed to the (220), (311), (400), (422), (511), and (440) planes, respectively, which have a good accordance with the spinel phase of magnetic iron oxide nanoparticles. These indicated that the surface modification of the Fe3O4 magnetic nanoparticles with functional groups did lead to retention of the crystalline structure.
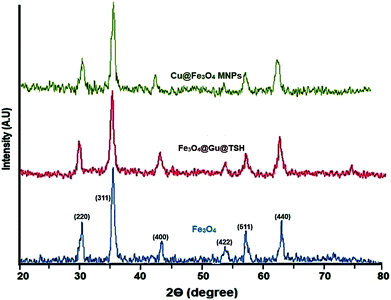 |
| Fig. 6 The XRD patterns of Fe3O4, Fe3O4@Gu@TSH and Cu@Fe3O4 MNPs. | |
Herein, we reported our outcomes for the effective and rapid preparation of tetrahydrobenzimidazo[2,1-b]quinazolin-1(2H)-ones and 2H-indazolo[2,1-b]-phthalazine-triones using an effective and reusable heterogeneous nanomagnetic catalyst, Cu@Fe3O4 MNPs, under solvent-free conditions (Scheme 2).
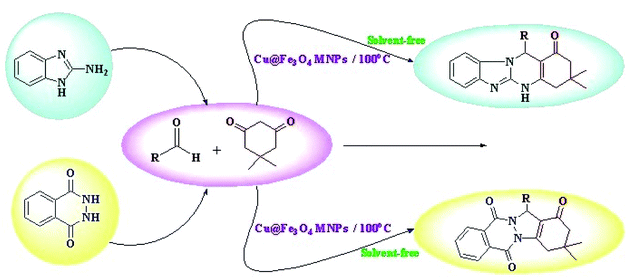 |
| Scheme 2 Preparation of tetrahydrobenzimidazo[2,1-b]quinazolin-1(2H)-ones and 2H-indazolo[2,1-b]-phthalazine-triones using Cu@Fe3O4 MNPs. | |
After synthesizing and identifying the Cu@Fe3O4 MNPs, in order to screen the reaction conditions for synthesizing tetrahydrobenzimidazo[2,1-b]quinazolin-1(2H)-ones, the impact of the solvents, the reaction temperature, and the concentrations of catalyst were explored using the reaction of 2-aminobenzimidazole (1 mmol), 4-chlorobenzaldehyde (1 mmol) and dimedone (1 mmol) (molar ratio: 1
:
1
:
1) as a model reaction. The outcomes are listed in Table 1. To attain the optimal reaction solvent, different solvents such as CH3CN, n-hexane, H2O, and EtOH in the existence of a certain concentrations of catalyst were examined (Table 1, entries 1–4) and most favorable conditions in terms of rate and yield were found under solvent-free conditions for the reaction (Table 1, entry 8). To explore the impact of reaction temperature, different temperatures (25, 80, 90, 100, and 110 °C) were used for comparing the reaction efficiency (Table 1, entries 5–9). In the absence of temperature, the reaction speed was very slow and the yield was negligible (Table 1, entry 5). The product yield was increased at higher temperatures (Table 1, entries 6–9). At 100 °C, under solvent-free conditions, the reaction rate was the maximum (Table 1, entry 8); however, a further increase in temperature did not indicate any sign of the enhancement (Table 1, entry 9). In the following phase of the study, the impact of catalyst loading on the completion of the reaction was investigated (Table 1, entries 8 and 10–11). The outcomes indicated that the reaction using 0.38 mol% of the Cu@Fe3O4 MNPs as the catalyst at 100 °C under solvent-free conditions proceeded with the highest yield at the short reaction time (Table 1, entry 8). Finally, when the model reaction was performed in the presence of 0.38 mol% of Fe3O4, Fe3O4@SiO2, Fe3O4@PC, Fe3O4@Gu, and Fe3O4@Gu@TSH, under the optimized conditions, the yield of the product were 43, 51, 59, 71 and 83%, respectively (Table 1, entries 12–16). The favourable comparison of the product yields for inputs 8 and 12–16 accurately exhibits that the catalyst activity increases when Fe3O4@Gu@TSH is coordinate to the CuCl2 through the nitrogen lone pair.
Table 1 Optimization of the three-component reaction of 2-aminobenzimidazole (1), 4-chlorobenzaldehyde (2f), and dimedone (3) under various conditionsa
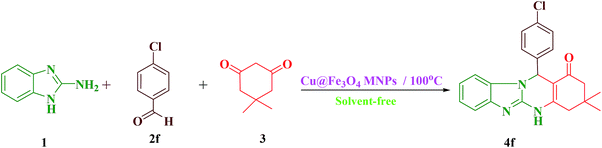
|
Entry |
Solvent |
Catalyst (mol%) |
Temp. |
Time (min) |
Yieldb (%) |
Reaction conditions: 2-aminobenzimidazole (1 mmol), 4-chlorobenzaldehyde (1 mmol), dimedone (1 mmol), and required amount of the catalysts. The yields refer to the isolated product. |
1 |
CH3CN |
Cu@Fe3O4 MNPs/0.38 |
Reflux |
70 |
61 |
2 |
n-Hexane |
Cu@Fe3O4 MNPs/0.38 |
Reflux |
120 |
Trace |
3 |
H2O |
Cu@Fe3O4 MNPs/0.38 |
Reflux |
70 |
47 |
4 |
EtOH |
Cu@Fe3O4 MNPs/0.38 |
Reflux |
60 |
64 |
5 |
Solvent-free |
Cu@Fe3O4 MNPs/0.38 |
25 °C |
12 |
Trace |
6 |
Solvent-free |
Cu@Fe3O4 MNPs/0.38 |
80 °C |
12 |
83 |
7 |
Solvent-free |
Cu@Fe3O4 MNPs/0.38 |
90 °C |
12 |
91 |
8 |
Solvent-free |
Cu@Fe3O4 MNPs/0.38 |
100 °C |
12 |
94 |
9 |
Solvent-free |
Cu@Fe3O4 MNPs/0.38 |
110 °C |
12 |
92 |
10 |
Solvent-free |
Cu@Fe3O4 MNPs/0.19 |
100 °C |
12 |
74 |
11 |
Solvent-free |
Cu@Fe3O4 MNPs/0.57 |
100 °C |
12 |
93 |
12 |
Solvent-free |
Fe3O4/0.38 |
100 °C |
60 |
43 |
13 |
Solvent-free |
Fe3O4@SiO2/0.38 |
100 °C |
55 |
51 |
14 |
Solvent-free |
Fe3O4@PC/0.38 |
100 °C |
50 |
59 |
15 |
Solvent-free |
Fe3O4@Gu/0.38 |
100 °C |
40 |
71 |
16 |
Solvent-free |
Fe3O4@Gu@TSH/0.38 |
100 °C |
25 |
83 |
Encouraged by these results, the scope and generality of the developed protocol regarding diverse aromatic and heterocyclic aldehydes were surveyed in the existence of 0.38 mol% of Cu@Fe3O4 MNPs at 100 °C under solvent-free conditions. The outcomes are presented in Table 2.
Table 2 Cu@Fe3O4 MNPs-catalyzed synthesis of tetrahydrobenzimidazo[2,1-b]quinazolin-1(2H)-one (4) derivativesa
Entry |
RCHO (2) |
Product |
Time (min) |
Yield (%) |
TONb |
TOFc (h−1) |
Mp (obsd) (°C) |
Mp (lit) (°C) |
Reaction conditions: 2-aminobenzimidazole (1 mmol), aldehyde (1 mmol), dimedone (1 mmol), Cu@Fe3O4 MNPs (0.38 mol%). Number of moles of product produced from 1 mole of catalyst. TON per unit of time. |
1 |
 |
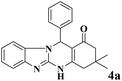 |
10 |
94 |
247 |
1482 |
>350 |
>350 (31) |
2 |
 |
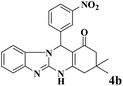 |
18 |
93 |
244 |
813 |
>300 |
>300 (32) |
3 |
 |
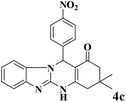 |
18 |
92 |
242 |
807 |
>350 |
>300 (15) |
4 |
 |
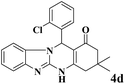 |
15 |
95 |
250 |
1000 |
>300 |
>300 (32) |
5 |
 |
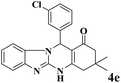 |
15 |
94 |
247 |
988 |
>300 |
>300 (32) |
6 |
 |
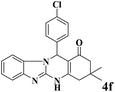 |
12 |
94 |
247 |
1235 |
>300 |
>300 (33) |
7 |
 |
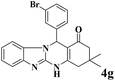 |
12 |
93 |
244 |
1220 |
>300 |
>300 (32) |
8 |
 |
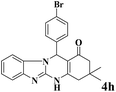 |
12 |
95 |
250 |
1250 |
346–349 |
348–350 (34) |
9 |
 |
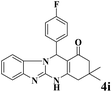 |
15 |
90 |
236 |
944 |
>300 |
>300 (33) |
10 |
 |
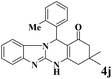 |
12 |
95 |
250 |
1250 |
233–235 |
236–238 (32) |
11 |
 |
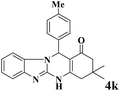 |
12 |
92 |
242 |
1210 |
240–243 |
242–244 (31) |
12 |
 |
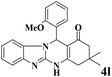 |
15 |
90 |
236 |
944 |
318–322 |
320–323 (34) |
13 |
 |
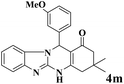 |
20 |
92 |
242 |
726 |
318–320 |
320–322 (31) |
14 |
 |
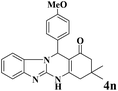 |
20 |
90 |
236 |
708 |
339–342 |
341–343 (34) |
15 |
 |
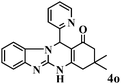 |
15 |
92 |
242 |
968 |
338–341 |
337–339 (41) |
We also report a rapid and efficient one-pot three-component preparation of some 2H-indazolo[2,1-b]phthalazine-triones via the reaction of phthalhydrazide, aromatic aldehydes, and dimedone in the existence of Cu@Fe3O4 MNPs (Table 3). To identify the best conditions, we carried out the reaction between 4-chlorobenzaldehyde (1 mmol), dimedone (1 mmol) and phthalhydrazide (1 mmol) (molar ratio: 1
:
1
:
1) in the existence of 0.38 mol% of Cu@Fe3O4 MNPs at 100 °C under solvent-free conditions. We found that the desired product with a very high yield (96%) within 15 min (Table 3, entry 8).
Table 3 Optimization of the three-component reaction of phthalhydrazide (5), 4-chlorobenzaldehyde (2g), and dimedone (3) under various conditionsa
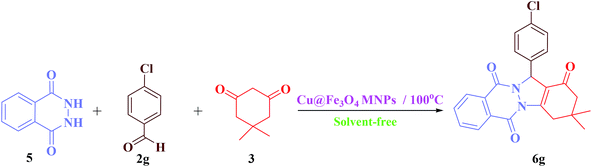
|
Entry |
Solvent |
Catalyst (mol%) |
Temp. |
Time (min) |
Yieldb (%) |
Reaction conditions: phthalhydrazide (1 mmol), 4-chlorobenzaldehyde (1 mmol), dimedone (1 mmol), and the required level of the catalysts. The yields refer to the isolated product. |
1 |
CH3CN |
Cu@Fe3O4 MNPs/0.38 |
Reflux |
70 |
63 |
2 |
n-Hexane |
Cu@Fe3O4 MNPs/0.38 |
Reflux |
120 |
Trace |
3 |
H2O |
Cu@Fe3O4 MNPs/0.38 |
Reflux |
60 |
33 |
4 |
EtOH |
Cu@Fe3O4 MNPs/0.38 |
Reflux |
60 |
64 |
5 |
Solvent-free |
Cu@Fe3O4 MNPs/0.38 |
25 °C |
12 |
Trace |
6 |
Solvent-free |
Cu@Fe3O4 MNPs/0.38 |
80 °C |
12 |
79 |
7 |
Solvent-free |
Cu@Fe3O4 MNPs/0.38 |
90 °C |
12 |
90 |
8 |
Solvent-free |
Cu@Fe3O4 MNPs/0.38 |
100 °C |
12 |
96 |
9 |
Solvent-free |
Cu@Fe3O4 MNPs/0.38 |
110 °C |
12 |
93 |
10 |
Solvent-free |
Cu@Fe3O4 MNPs/0.19 |
100 °C |
12 |
71 |
11 |
Solvent-free |
Cu@Fe3O4 MNPs/0.57 |
100 °C |
12 |
94 |
12 |
Solvent-free |
Fe3O4/0.38 |
100 °C |
80 |
26 |
13 |
Solvent-free |
Fe3O4@SiO2/0.38 |
100 °C |
65 |
37 |
14 |
Solvent-free |
Fe3O4@PC/0.38 |
100 °C |
60 |
45 |
15 |
Solvent-free |
Fe3O4@Gu/0.38 |
100 °C |
45 |
69 |
16 |
Solvent-free |
Fe3O4@Gu@TSH/0.38 |
100 °C |
25 |
85 |
After optimization of the conditions for the model reaction, a range of different tetrahydrobenzimidazo[2,1-b]quinazolin-1(2H)-ones was synthesized with array of arylaldehydes bearing either electron-withdrawing or electron-donating substituents and aliphatic aldehyde by this protocol (Table 4).
Table 4 Cu@Fe3O4 MNPs-catalyzed synthesis of 2H-indazolo[2,1-b]phthalazine-trione (6) derivativesa
Entry |
RCHO (2) |
Product |
Time (min) |
Yield (%) |
TONb |
TOFc (h−1) |
Mp (obsd) (°C) |
Mp (lit) (°C) |
Reaction conditions: phthalhydrazide (1 mmol), aldehyde (1 mmol), dimedone (1 mmol), Cu@Fe3O4 MNPs (0.38 mol%). Number of moles of product produced from 1 mole of catalyst. TON per unit of time. |
1 |
 |
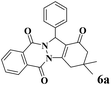 |
12 |
95 |
250 |
1250 |
232–234 |
234–235 (36) |
2 |
 |
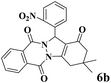 |
18 |
92 |
242 |
807 |
216–219 |
214–216 (35) |
3 |
 |
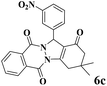 |
15 |
93 |
245 |
980 |
216–219 |
214–216 (37) |
4 |
 |
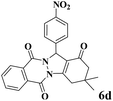 |
15 |
91 |
239 |
956 |
180–182 |
179–180 (37) |
5 |
 |
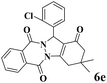 |
15 |
91 |
239 |
956 |
207–210 |
198–200 (37) |
6 |
 |
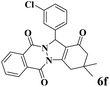 |
15 |
93 |
245 |
980 |
212–214 |
215–217 (15) |
7 |
 |
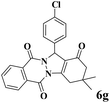 |
12 |
96 |
253 |
1265 |
212–214 |
215–217 (36) |
8 |
 |
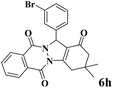 |
12 |
93 |
245 |
1225 |
208–211 |
207–209 (35) |
9 |
 |
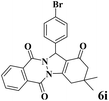 |
12 |
95 |
250 |
1250 |
208–211 |
207–209 (35) |
10 |
 |
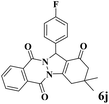 |
12 |
91 |
239 |
1195 |
208–211 |
207–209 (36) |
11 |
 |
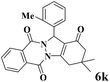 |
15 |
91 |
239 |
956 |
225–229 |
227–230 (37) |
12 |
 |
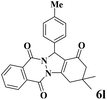 |
15 |
93 |
245 |
980 |
217–220 |
220–222 (36) |
13 |
 |
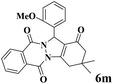 |
18 |
91 |
239 |
797 |
203–205 |
201–202 (35) |
14 |
 |
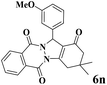 |
18 |
95 |
250 |
833 |
223–225 |
224–226 (37) |
15 |
 |
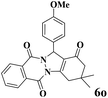 |
20 |
93 |
245 |
735 |
237–239 |
238–240 (36) |
16 |
 |
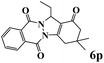 |
35 |
81 |
213 |
365 |
146–149 |
145–147 (42) |
A possible mechanism for the creation of tetrahydrobenzimidazo[2,1-b]quinazolin-1(2H)-ones and 2H-indazolo[2,1-b]phthalazine-triones is proposed in Scheme 3. The reaction occurs via initial formation of the heterodyne 7 by nucleophilic addition of dimedone 3 to aldehyde 2 followed by dehydration. The second step involves initial formation of intermediates 8 and 9 by Michael-type addition of the 2-aminobenzimidazole 1 and phthalhydrazide 5 with heterodyne 7, followed by cyclization of the corresponding products 4 and 6.
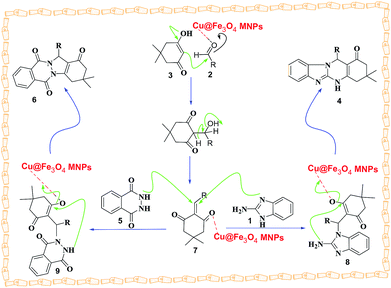 |
| Scheme 3 A plausible mechanism for the creation of tetrahydrobenzimidazo[2,1-b]quinazolin-1(2H)-ones and 2H-indazolo[2,1-b]phthalazine-triones in the existence of Cu@Fe3O4MNPs under solvent-free conditions. | |
To obtain the degree of leaching of the copper from the heterogeneous catalyst, in a typical experiment, phthalhydrazide (1 mmol), 4-chlorobenzaldehyde (1 mmol), dimedone (1 mmol), and Cu@Fe3O4 MNPs (0.38 mol%) and 3 mL of EtOH were placed in a round bottom flask and stirred at 100 °C for 30 min. Then, the catalyst was separated by a prominent magnetic field and allowed to the residue solution to be stirred at 100 °C for further 60 min. This experiment illustrated only a little progress in the yield of the product (GC) in the absence of Cu@Fe3O4 MNPs, which corroborates little leaching of copper and confirms responsibility of the heterogeneous Cu@Fe3O4 MNPs in catalyzing the desired reaction.
To evaluate the recycled Cu@Fe3O4MNPs performance, this nanocatalyst was reused in the reaction of 2-aminobenzimidazole/phthalhydrazide with 4-hydroxycoumarin, 4-chlorobenzaldehyde, and dimedone for at least seven runs under the optimal reaction conditions (Fig. 7). To achieve this purpose, at the end of the reaction, the mixture was dissolved in a hot mixture of ethyl acetate and ethanol (4
:
10 ratio) and then the catalyst was removed using an appropriate magnet. The recovered Cu@Fe3O4 MNPs were washed with chloroform, dried, and reused with a negligible reduction of its activity.
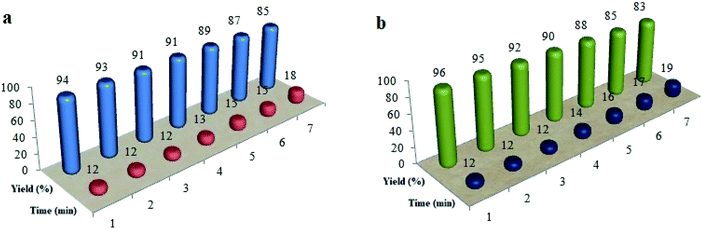 |
| Fig. 7 The recycling of Cu@Fe3O4 MNPs in the preparation of tetrahydrobenzimidazo[2,1-b]quinazolin-1(2H)-ones (a) and 2H-indazolo[2,1-b]phthalazine-triones (b). | |
Table 5 shows the efficiency of Cu@Fe3O4 MNPs as the catalyst in the preparation of tetrahydrobenzimidazo[2,1-b]quinazolin-1(2H)-ones and 2H-indazolo[2,1-b]phthalazine-triones compared with several of the previously mentioned homogeneous and heterogeneous catalysts. As clearly shown in Table 5, although all the reported catalysts are suitable for certain synthetic conditions, the catalytic behavior of the present catalytic system is remarkable in terms of low reaction times, easy work-up procedures, low catalyst loading, and simple recovery of the catalyst.
Table 5 Comparison of the current methods with other reported strategies for synthesizing tetrahydrobenzimidazo[2,1-b]quinazolin-1(2H)-ones and 2H-indazolo[2,1-b]phthalazine-triones
Entry |
Catalyst |
Catalyst loading |
Conditions |
Temp. (°C) |
Time (min) |
Yield (%) |
Ref. |
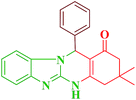 |
1 |
H6P2W18O62·18H2O |
0.01 equiv. |
CH3CN |
Reflux |
15 |
96 |
15 |
2 |
P-TsOH·H2O |
15 mol% |
CH3CN |
40–50 |
25 |
95 |
33 |
3 |
NH2SO3H |
0.05 mmol |
CH3CN |
Reflux |
15 |
96 |
38 |
4 |
Iodine |
10 mol% |
CH3CN |
Reflux |
10 |
84 |
14 |
5 |
Guanidinium chloride |
10 mol% |
Solvent-free |
110 |
30 |
91 |
39 |
6 |
Cu@Fe3O4 MNPs |
0.38 mol% |
Solvent-free |
100 |
12 |
94 |
This work |
![[thin space (1/6-em)]](https://www.rsc.org/images/entities/char_2009.gif) |
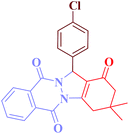 |
1 |
CAN |
5 mol% |
PEG 400 |
50 |
120 |
90 |
24 |
2 |
Iodine |
0.1 g |
Sonic bath |
25–30 |
10 |
92 |
27 |
3 |
[PVP–SO3H]Cl (HMT) |
8 mol% |
Solvent-free |
80 |
25 |
92 |
31 |
4 |
H2SO4 |
0.15 mmol |
[bmim]BF4 |
80 |
30 |
88 |
25 |
5 |
Mg (HSO4)2 |
10 mol% |
Solvent-free |
100 |
4 |
88 |
40 |
6 |
Cu@Fe3O4 MNPs |
0.38 mol% |
Solvent-free |
100 |
12 |
96 |
This work |
Conclusion
In summary, a novel, effective, and recyclable nanocatalyst, i.e., Cu@Fe3O4 MNPs, was prepared, characterized, and verified in terms of its catalytic activity. Cu@Fe3O4 MNPs was proven to be an eco-friendly environment for the preparation of tetrahydrobenzimidazo[2,1-b]quinazolin-1(2H)-ones and 2H-indazolo[2,1-b]phthalazine-triones via two one-pot three-component condensations under solvent-free conditions. This method provides several advantages including applying a green catalyst, lower loading of the catalyst, magnetically separable, omitting organic solvent, simple operation, and good to high yields.
Conflicts of interest
There are no conflicts to declare.
Acknowledgements
This investigation has been supported by the Guangxi Natural Science Foundation (No. 2018JJA120123).
References
- B. M. Trost, Science, 1991, 254, 1471 CrossRef CAS PubMed.
- M. Valden, X. Lai and D. W. Goodman, Science, 1998, 281, 1647 CrossRef CAS PubMed.
- G. Hutchings, Nanocatalysis: Synthesis and Applications, ed. V. Polshettiwar and T. Asefa, John Wiley & Sons, 2013 Search PubMed.
- D. J. Cole-Hamilton, Science, 2003, 299, 1702 CrossRef CAS PubMed.
- R. J. White, R. Luque, V. L. Budarin, J. H. Clark and D. J. Macquarrie, Chem. Soc. Rev., 2009, 38, 481 RSC.
- A. C. Templeton, M. J. Hostetler, E. K. Warmoth, S. Chen, C. M. Hartshorn, V. M. Krishnamurthy, M. D. Forbes and R. W. Murray, J. Am. Chem. Soc., 1998, 120, 4845 CrossRef CAS.
- J. A. Gladysz, Chem. Rev., 2002, 102, 3215 CrossRef CAS PubMed.
- A. Zecchina, S. Bordiga and E. Groppo, Selective Nanocatalysts and Nanoscience: Concepts for Heterogeneous and Homogeneous Catalysis, John Wiley & Sons, 2011 Search PubMed.
- V. Alagarsamy and U. S. Pathak, Bioorg. Med. Chem., 2007, 15, 3457 CrossRef CAS PubMed.
- V. Alagarsamy, Pharmazie, 2004, 59, 753 CAS.
- V. Alagarsamy, G. Murugananthan and R. Venkateshperumal, Biol. Pharm. Bull., 2003, 26, 1711 CrossRef CAS.
- M. J. Hour, L. J. Huang, S. C. Kuo, Y. Xia, K. Bastow, Y. Nakanishi, E. Hamel and K. H. Lee, J. Med. Chem., 2000, 43, 4479 CrossRef CAS PubMed.
- V. Alagarsamy, R. Revathi, S. Meena, K. V. Ramaseshu, S. Rajasekaran and E. De Clercq, Indian J. Pharm. Sci., 2004, 66, 459 CAS.
- R. G. Puligoundla, S. Karnakanti, R. Bantu, N. Kommu, S. B. Kondra and L. Nagarapu, Tetrahedron Lett., 2013, 54, 2480 CrossRef CAS.
- M. M. Heravi, L. Ranjbar, F. Derikvand, B. Alimadadi, H. A. Oskooie and F. F. Bamoharram, Mol. Diversity, 2008, 12, 181 CrossRef CAS PubMed.
- G. M. Ziarani, A. Badiei, Z. Aslani and N. Lashgari, Arabian J. Chem., 2015, 8, 54 CrossRef.
- G. Krishnamurthy and K. V. Jagannath, J. Chem. Sci., 2013, 125, 807 CrossRef CAS.
- A. E. Mourad, A. A. Aly, H. H. Farag and E. A. Beshr, Beilstein J. Org. Chem., 2007, 3, 1 CrossRef PubMed.
- J. Li, Y. F. Zhao, X. Y. Yuan and J. X. Xu, Molecules, 2006, 11, 574 CrossRef CAS PubMed.
- C. K. Ryu, R. E. Park, M. Y. Ma and J. H. Nho, Bioorg. Med. Chem. Lett., 2007, 17, 2577 CrossRef CAS PubMed.
- N. Watanabe, Y. Kabasawa, Y. Takase, M. Matsukura, K. Miyazaki, H. Ishihara, K. Kodama and H. Adachi, J. Med. Chem., 1998, 41, 3367 CrossRef CAS PubMed.
- S. Grasso, G. DeSarro, N. Micale, M. Zappala, G. Puia, M. Baraldi and C. Demicheli, J. Med. Chem., 2000, 43, 2851 CrossRef CAS PubMed.
- Y. Nomoto, H. Obase, H. Takai, M. Teranishi, J. Nakamura and K. Kubo, Chem. Pharm. Bull., 1990, 38, 2179 CrossRef CAS PubMed.
- K. Mazaahir, C. Ritika and J. Anwar, Chin. Sci. Bull., 2012, 57, 2273 CrossRef CAS.
- J. M. Khurana and D. Magoo, Tetrahedron Lett., 2009, 50, 7300 CrossRef CAS.
- B. Dam, M. Saha, R. Jamatia and A. K. Pal, RSC Adv., 2016, 6, 54768 RSC.
- A. Varghese, A. Nizam, R. Kulkarni and L. George, Eur. J. Chem., 2013, 4, 132 CrossRef CAS.
- X. N. Zhao, G. F. Hu, M. Tang, T. T. Shi, X. L. Guo, T. T. Li and Z. H. Zhang, RSC Adv., 2014, 4, 51089 RSC.
- H. R. Shaterian, M. Ghashang and M. Feyzi, Appl. Catal., A, 2008, 345, 128 CrossRef CAS.
- A. R. Kiasat, S. Noorizadeh, M. Ghahremani and S. J. Saghanejad, J. Mol. Struct., 2013, 1036, 216 CrossRef CAS.
- O. G. Jolodar, F. Shirini and M. Seddighi, RSC Adv., 2016, 6, 44794 RSC.
- A. Shaabani, E. Farhangi and A. Rahmati, Comb. Chem. High Throughput Screening, 2006, 9, 771 CrossRef CAS PubMed.
- M. R. Mousavi and M. T. Maghsoodlou, Monatsh. Chem., 2014, 145, 1967 CrossRef CAS.
- F. Shirini, M. Seddighi and O. G. Jolodar, J. Iran. Chem. Soc., 2016, 13, 1077 CrossRef.
- F. Shirini, M. S. N. Langarudi and O. G. Jolodar, Dyes Pigm., 2015, 123, 186 CrossRef CAS.
- B. Mombani Godajdar, A. R. Kiasat and M. M. Hashemi, Heterocycles, 2013, 87, 559 CrossRef.
- A. Rostami, B. Tahmasbi and A. Yari, Bull. Korean Chem. Soc., 2013, 34, 1521 CrossRef CAS.
- M. M. Heravi, F. Derikvand and L. Ranjbar, Synth. Commun., 2010, 40, 677 CrossRef CAS.
- R. Talaei and A. Olyaei, Iran. J. Catal., 2016, 6, 339 CAS.
- H. R. Shaterian, F. Khorami, A. Amirzadeh, R. Doostmohammadi and M. Ghashang, Journal of the Iranian Chemical Research, 2009, 2, 57 Search PubMed.
- M. Dehghan, A. Davoodnia, M. R. Bozorgmehr and F. F. Bamoharram, Org. Prep. Proced. Int., 2017, 49, 236 CrossRef CAS.
- R. G. Vaghei, R. Karimi-Nami, Z. Toghraei-Semiromi, M. Amiri and M. Ghavidel, Tetrahedron, 2011, 67, 1930 CrossRef.
Footnote |
† Electronic supplementary information (ESI) available. See DOI: 10.1039/c9ra01509d |
|
This journal is © The Royal Society of Chemistry 2019 |
Click here to see how this site uses Cookies. View our privacy policy here.