DOI:
10.1039/C9RA01551E
(Paper)
RSC Adv., 2019,
9, 14580-14585
Ammonia borane dehydrogenation and selective hydrogenation of functionalized nitroarene over a porous nickel–cobalt bimetallic catalyst†
Received
1st March 2019
, Accepted 18th April 2019
First published on 9th May 2019
Abstract
The hydrolysis of ammonia borane is a promising strategy for hydrogen energy exploration and exploitation. The in situ produced hydrogen could be directly utilized in hydrogenation reactions. In this work, a bimetallic nickel–cobalt material with porous structure was developed through the pyrolysis of ZIF-67 incorporated with Ni ions. Through the introduction of Ni(NO3)2 as an etching agent, the ZIF-67 polyhedrons were transformed into hollow nanospheres, and further evolved into irregular nanosheets. The bimetallic NiCo phase was formed after pyrolysis in a nitrogen atmosphere at high temperature, with the decomposition and release of organic ligands as gaseous molecules under flowing nitrogen. The obtained bimetallic NiCo porous materials show superior catalytic performance towards hydrolytic dehydrogenation of ammonia borane, thereby nitrobenzene with reducible functional groups can be reduced with high selectivity to the corresponding aniline.
1. Introduction
Metal–organic frameworks (MOFs) are crystalline porous materials prepared by the self-assembly of metal ions and organic linkers, which have been used as templates or precursors for nanostructured porous materials.1–4 The morphology of MOF materials shows diversity because of the flexible and controllable composition, structure and pore size.5 They are attractive as ideal precursors for the preparation of porous nano-materials with various morphologies or structures.6 MOF-derived metal composites often show large surface area and hierarchical pore structures. Metal nanocrystals,7 oxides,8 sulfides,9 nitrides10 and phosphides11 have been successfully prepared through the evolution of MOFs, and have shown extraordinary performance in catalysis, sensing, energy storage and conversion, separation and other fields.12 ZIF-67 is a kind of zeolite imidazole skeleton material with Co2+ ions as metal sites and 2-methylimidazole as organic ligands.13 Through pyrolysis at high temperature, ZIF-67 converted into nitrogen doped carbon encapsulated Co/CoOx nanocrystals (denoted as Co–N–C). The Co–N–C species has shown superior catalytic performance in both oxidation and reduction reactions.14–16 Through further phosphorization or sulfuration, the corresponding CoP–N–C or CoS–N–C can be used as an electrocatalytic material for the oxygen reduction reaction (ORR) or water splitting.9,17 The Co-based materials derived from might be promising alternative non-noble metal catalyst in heterogeneous catalysis.
Functional anilines are widely existed as key intermediates in dyes, pigments, herbicides and pharmaceuticals.18 The catalytic hydrogenation of nitroarenes using hydrogen as a reduce agent is the main protocol for the high efficiency and atom economic.19 Noble metal catalysts such as Pd and Pt have shown excellent catalytic activity in nitrobenzene hydrogenation.20–23 However, precious metals are rare, expensive and difficult to control the selectivity of hydrogenation. It is imperative to find non-precious metal catalysts that can replace precious metals. It has been reported that non-noble metal catalysts such as Fe and Co have been used in nitrobenzene hydrogenation.24–26 Actually, the direct use of hydrogen is uneconomical and increases the risk of reaction. Catalytic hydrogenation of non-noble metals generally requires higher reaction temperature and pressure.27–30 It is highly desirable to realize selective hydrogenation of nitrobenzene over non-noble metal catalysts under mild conditions.
Ammonia borane (NH3BH3, AB) is non-toxic, safe, stable and environmentally friendly compounds with high quality hydrogen storage (mass fraction of 19.6%).31,32 Based on these characteristics, it is the most potential new type of hydrogen storage.33–35 To combine the dehydrogenation of ammonia borane and hydrogenation of nitrobenzene would be an ideal strategy to realize selective hydrogenation of nitrobenzene with reducible functional groups under mild condition.36 To find cheap and efficient catalysts to reduce the cost of hydrogen production would be highly desirable but challenging. The non-precious metal catalysts, nickel and cobalt based nanoparticles or alloy catalysts have shown superior catalytic effect in hydrogen production by ammonia borane hydrolysis.37,38
The convenient and effective preparation strategy of non-noble catalysts is still a challenge due to the lower electronegativity.39 Thermal annealing with high temperature is always need, which often results in serious agglomeration and losing of surface area or active sites. To construct porous structure materials is propitious to expose more active site and mass transfer of substances in catalysis.40 The developed porous catalysts such as Ni, Au, Cu, etc. have shown improved or enhanced performance in many catalytic processes.41–43 In general, the synthetic methods of porous catalysts are based on template, corrosion or assembling of smaller particles.44,45 To develop novel, more convenient and effective synthetic method is crucial to higher requirements in catalysis. Here, we present a convenient and effective method to synthesize porous NiCo bimetallic catalyst with controllable composition through pyrolysis of Ni-ZIF-67. The obtained NiCo bimetallic catalysts show excellent activity towards hydrogen production by hydrolysis of ammonia borane.
2. Experimental
2.1 Materials
Co(NO3)2·6H2O (99%) and Ni(NO3)2·6H2O (99%) were purchased from Aladdin. Ammonia borane (90%) was purchased from Aldrich. Methanol and 2-methylimidazole were of analytical grade from the Sinopharm Chemical Reagent. All kinds of nitrobenzenes were of analytical grade from the Energy Chemical. All the reagents in this work were used without further purification.
2.2 Synthesis of ZIF-67
In a typical synthesis, 0.5 g Co(NO3)2 was dissolved in 15 mL of methanol; then 0.5 g 2-methylimidazole was dissolved in 15 mL of methanol. Then both solutions were mixed and stirred for 24 h at room temperature, then the resulting ZIF-67 solids were separated by centrifuging and washed with methanol for 3 times, and finally dried at 50 °C at vacuum for 24 h.
2.3 Synthesis of Ni-ZIF-67
The obtained ZIF-67 (0.1 g) was dispersed in 30 mL methanol and 0.1 g Ni(NO3)2 with 10 mL methanol was introduced, then stirred for 24 h at room temperature. The Ni-ZIF-67 was obtained after centrifuging and washing with methanol for 3 times. The resulting Ni-ZIF-67 solids were finally dried at 50 °C at vacuum for 24 h.
2.4 Synthesis of bimetallic NiCo porous materials
100 mg of Ni-ZIF-67 solid was placed into a quartz boat, which was charged into the middle of a quartz tube in a furnace with a continuous nitrogen flow of 50 mL min−1. The furnace was heated from room temperature to the targeted temperature (600–900 °C) with a programmed heating rate of 2.5 °C min−1. After the pyrolysis for 2 hours, the sample was naturally cooled down to room temperature. The resultant samples were denoted as NiCo-T (T denotes as pyrolysis temperature).
2.5 Characterization
X-ray diffraction (XRD) was performed on a Philips X'pert diffractometer equipped with a Ni-filtered Cu Kα radiation source. The particle size and morphology of as-synthesized samples were determined by using Hitachi model H-800 transmission electron microscope. High-resolution transmission electron microscopy (HRTEM) images were taken using a JEM-2100 electron microscope. X-ray photoelectron spectroscopy (XPS) experiments were performed on a ULVAC PHI Quantera microprobe. Binding energies (BE) were calibrated by setting the measured binding energies of C 1s to 284.6 eV.
2.6 Dehydrogenation of ammonia borane
The catalysts were suspended in water (5.0 mL) of a two-necked round-bottomed flask. A gas cylinder filled with water was connected to one neck of the reaction flask (the other neck was sealed) to measure the volume of hydrogen. The reaction was initiated in a water bath at 25 °C under ambient atmosphere. 1 mmol of ammonia borane was added into the reaction flask with stirring. By measuring the displaced water, the produced volume of hydrogen could be recorded.
2.7 Hydrogenation of nitrobenzene
In a typical reaction, nitrobenzene (0.5 mmol) was charged into a round bottom flask with ethanol (0.5 mL) and deionized water (5 mL). Then porous NiCo bimetallic catalyst (0.02 mmol) was introduced. After ammonia borane (1 mmol) was added, the round bottom flask was sealed immediately and the reaction mixture was stirred at 25 °C. The conversion and selectivity were determined by GC using n-hexadecane (100 μL) internal standard.
3. Results and discussion
3.1 Characterization of bimetallic NiCo porous materials
The synthetic route of NiCo bimetallic catalyst is schematically shown in Scheme 1. The corresponding structure model for the ZIF-67-Co rhombic dodecahedron is schematically illustrated. ZIF-67 was chosen for its high content of cobalt, which could be promising precursor for the synthesis of Co nanoparticles or metal oxide. Ni(NO3)2 was introduced as etching agents to transform the ZIF-67 polyhedrons into hollow nanospheres, and irregular nanosheets were subsequently generated with further increase the Ni salt. After pyrolysis in nitrogen atmosphere at high temperature, the Ni2+ and Co2+ were reduced in situ into bimetallic NiCo phase. Meanwhile, the organic ligand decomposed into gaseous molecule and released at high temperature under flowing nitrogen.
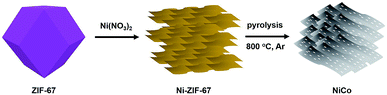 |
| Scheme 1 Schematic illustration of the synthetic route to porous NiCo bimetallic catalyst. | |
Through the characterization by Transmission Electron Microscopy (TEM), ZIF-67 with polyhedrons shape and smooth surface which have a diameter of 700 nm and an edge length of about 500 mm could be obtained according to reported methods (Fig. 1a).15 Fig. 1a shows that the architectures are constructed from many wrinkled nanosheets. Based on the incremental amount of Ni salt, ZIF-67 polyhedrons were firstly etched into hollow nanospheres and then destroyed by more Ni salt into nanosheets (Fig. S1†). After pyrolysis of the eventually obtained Ni-ZIF-67 at 800 °C, the Ni2+ and Co2+ were simultaneously reduced into NiCo crystalline, which exhibit the synthesized architectures are composed of sheet-like nanostructures. Interestingly, different from the reported work about the organic ligand of ZIF-67 convert into nitrogen doped carbon materials, we found that no carbon was formed and only metallic NiCo was produced. The magnified TEM image (Fig. 1b) confirms the nanoporous structures of these nanosheets. The NiCo bimetallic material displays the honeycomb-like microstructures with pores, in which small NiCo nanoparticles interconnect with each other, forming a large number of pores, and interstitial structures on their exterior surfaces. Based on the characterization results, it is reasonably supported that the pyrolysis of the Ni-ZIF-67 would induce thermal decomposition of organic ligands and the release of a mass of gaseous molecule from the interior of the nanosheets, thus leading to the formation of porosity left inside the particles. The High Resolution Transmission Electron Microscopy (HRTEM) image (Fig. 1f) shows the lattice fringes with interfringe distances of 0.259 nm and 0.224 nm, which is between the characteristics of Ni and Co crystal phases in the (200) plane and (220) plane respectively. The energy-dispersive X-ray spectroscopy (EDS) mapping profile provides evidence of a homogeneous distribution of nickel and cobalt (Fig. 1g) in bimetallic NiCo porous material. Almost no carbon elements and nitrogen elements can be detected, which is consistence with the previous inference about the disappearance of organic moiety. The above characterization on the NiCo architecture reveals that pores in the architecture are actually constructed by the highly packed NiCo nanoparticles.
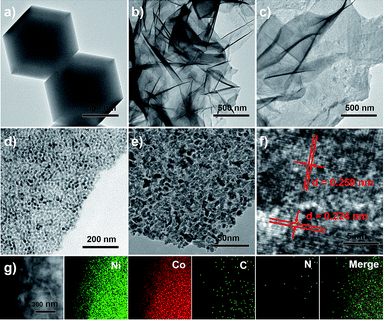 |
| Fig. 1 (a) TEM image of representative ZIF-67 nanocrystals; (b) TEM image of Ni-ZIF-67 with Ni/Co ratio 1 : 1; (c) TEM image of NiCo bimetallic materials obtained from pyrolysis of Ni-ZIF-67 at 800 °C; (d and e) the magnified TEM images of NiCo bimetallic materials; (f) HRTEM image of NiCo bimetallic materials; (g) EDS-mapping of NiCo bimetallic materials. | |
To further study the conversion of ZIF-67 to Ni-ZIF-67 after adding Ni(NO3)2, the phase structure of the samples was analyzed by X-ray diffraction (XRD). The X-ray diffraction (XRD) patterns confirmed high crystallinity and zeolite type structure of ZIF-67 (Fig. S1†), and the diffraction peaks are identical to its corresponding simulated pattern. After the introduction of Ni salt, the XRD pattern of product changed notably with gradually increasing the amount (Fig. S2†). The characteristic peaks of ZIF-67 disappears, and the appearance of a new narrow diffraction peaks at 10° was found. After calcined at 800 °C, all of the detectable diffraction peaks agree well with standard data of cubic phase NiCo, and no other phases can be detected, indicating that the as-obtained calcined product is pure crystalline (Fig. 2a). Moreover, with increasing calcination temperature, the shape of these diffraction peaks become sharper and narrower. It is confirmed that the successful conversion of Ni-ZIF-67 to cubic phase NiCo after thermal treatment based on above HRTEM and XRD results. To evaluate the specific surface areas and porosity of the obtained porous NiCo bimetallic catalysts, the N2 adsorption–desorption isotherms and pore sizes were shown in Fig. 2b. The BET surface areas is 184.2 m2 g−1, and micropore size centered at 1.2 nm.
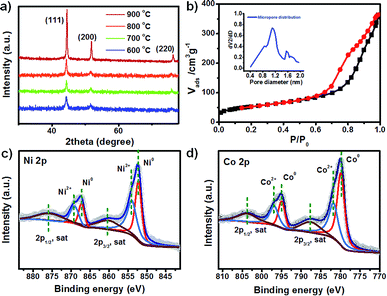 |
| Fig. 2 (a) The XRD patterns of the samples resulting from annealing Ni-ZIF-67 at various temperatures; (b) N2 adsorption/desorption isotherms and pore distribution of porous NiCo bimetallic catalyst obtained at 800 °C; XPS spectra of Ni 2p peaks (c) and Co 2p peaks (d) from Ni1Co1 bimetallic porous materials annealing at 800 °C. | |
The chemical and electronic states of the NiCo architecture were further determined by X-ray photoelectron spectroscopy (XPS). All of the binding energies in the XPS analysis were corrected for specimen charging by referencing them to the C 1s peak (284.6 eV). Fig. 2c shows the high-resolution Ni 2p spectrum. It presents two prominent peaks at 852.4 and 867.5 eV, assigned to the Ni 2p3/2 and Ni 2p1/2 peaks respectively. Further deconvolution of the Ni 2p spectra yields two fitting peaks at binding energies of 852.2 and 867.2 eV are ascribed to Ni0, while another two fitting peaks at 854.0 and 869.2 eV are attributed to Ni2+. Fig. 2d shows the deconvolution of high-resolution Co 2p spectrum, and the Co 2p spectra yields two fitting peaks at binding energies of 779.9 and 794.9 eV are ascribed to Co0, while another two fitting peaks at 781.9 and 796.9 eV are attributed to Co2+. Therefore, the main valence state of NiCo bimetallic porous materials is zero with partial oxidation on the surface when exposing to air.
3.2 Catalytic activity in dehydrogenation of ammonia borane
The as-prepared porous NiCo bimetallic catalysts were tested for the hydrolytic dehydrogenation of AB. For the Ni1Co4 catalysts, a rapid (20 minutes) and nearly linear hydrogen evolution curves was obtained without observable induction period (Fig. 3a). As the catalyst obtained from pyrolysis of pure ZIF-67 (denoted as Co–N–C, Fig. S3 and S4†) was employed in the hydrogen generation of aqueous AB, moderate activities for the hydrolysis was found (60 minutes). The precursors were also studied as catalyst for the hydrolytic dehydrogenation of AB. The pure Ni(NO3)2 and ZIF-67 show little activity and negligible activities respectively. The difference in the activity may be ascribed to the introduction of Ni in Co–N–C structure and the electronic effects between Ni and Co. Further reasonable modification of the metal ratios showed that the reaction time decreased with the increasing of Ni amount, and the Ni1Co1 could complete the reaction during just 7 minutes (Fig. 3b). The temperature dependence of the catalytic reaction was studied in the range 25–35 °C, with initial AB and Ni1Co1 concentrations of 1 and 0.05 mmol, respectively; the results are shown in Fig. 3c. When the hydrogen generation of aqueous AB was initiated at 30 °C and 35 °C, the reaction could be completed at three and two minutes.
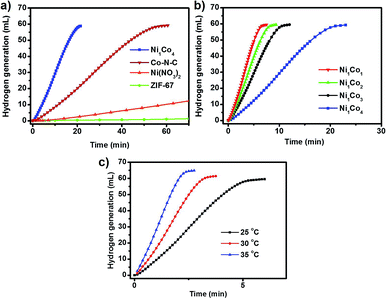 |
| Fig. 3 (a) Volume of hydrogen evolved versus reaction time for AB dehydrogenation reaction (1 mmol) catalyzed by the different catalysts; (b) volume of hydrogen evolved versus reaction time for AB dehydrogenation reaction catalyzed by the as-prepared bimetallic NiCo porous catalysts with different Ni/Co ratio; (c) volume of hydrogen evolved versus reaction time for AB dehydrogenation reaction catalyzed by the as-prepared bimetallic Ni1Co1 porous catalysts with different temperatures. | |
3.3 Catalytic activity in hydrogenation of nitrobenzene
To demonstrate the general applicability of nitroarenes reduction of the porous NiCo bimetallic catalyst, we explored the hydrogenation of various nitroarenes with reducible functional groups through AB dehydrogenation (Table 1). Cyano group substituted nitrobenzene at para-position converted into aniline by nearly 100% conversion and selectivity (entry 1). The reduction of ester or amide substituted nitrobenzene produced corresponding anilines with 88% or 85% conversion (entries 2 and 3). Particularly, the most-challenging nitrobenzene with ethynyl or vinyl substituted could also be reduced into ethynyl or vinyl aniline with 97% or 99% conversion and near 100% selectivity (entries 4 and 5). Halogenated nitrobenzenes, such as chloro-, bromo-substituted, were converted into halogenated anilines in excellent conversion (89–95%) and selectivity (95–98%) (entries 6 and 7). Moreover, 3-nitropyridine compound could also be reduced into the 3-aminopyridine which is an important building blocks in pharmaceuticals and agrochemicals with 98% conversion and 100% selectivity (entry 8). The above results show that the porous NiCo bimetallic catalyst is highly effective in AB dehydrogenation and chemo-selective hydrogenation of functionalized nitrobenzenes. Compared with the reported catalysts (Table S1†), our catalysts can achieve the mildest reaction conditions for nitrobenzene hydrogenation, and comparable catalytic activity towards combination of ammonia borane dehydrogenation and nitrobenzene hydrogenation.
Table 1 The hydrogenation of functionalized nitrobenzene through ammonia borane dehydrogenation with porous NiCo bimetallic catalyst
3.4 Recycle and stability of bimetallic NiCo porous materials
The bimetallic NiCo porous materials used in nitrobenzene hydrogenation could be easily recovered by an applied magnetic field after the reaction. The test of AB dehydrogenation over bimetallic NiCo porous materials was repeated five times to evaluate the catalyst stability. After each experiment, the catalyst was separated from the reaction products by an applied magnetic field, washed with water and reused in the next cycle. As shown in Fig. 4a, which indicates that the bimetallic NiCo porous materials remain stable under the reaction conditions. Bimetallic NiCo porous materials can remain effective activity and selectivity after five cycle reactions. Moreover, we also tested the cycle performance of bimetallic NiCo porous materials in the hydrogenation of nitrobenzene with AB dehydrogenation. The results showed that there was no significant decline in catalytic activity of bimetallic NiCo porous materials after five cycles (Fig. 4b). The spent catalysts were characterized after five cycles and found that NiCo still maintained the morphology as the initial catalyst (Fig. 4c), and the surface state of the catalysts did not change significantly (Fig. 4d). These results reveal that mild reaction conditions are not enough to change the overall structure of the catalyst, and the reductive reaction conditions avoid the oxidation of NiCo in air. The mild reaction condition, ultra-high catalytic efficiency and stability would be advantageous and promising in the practical application.
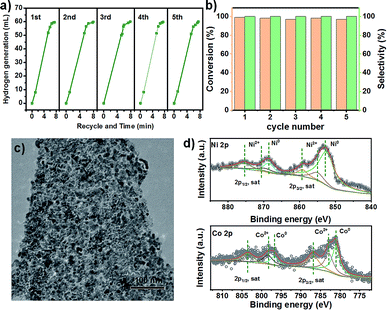 |
| Fig. 4 (a) The cycle experiments of ammonia borane dehydrogenation over porous NiCo bimetallic catalyst; (b) the cycle results of nitrobenzene hydrogenation over porous NiCo bimetallic catalyst (using 4-nitrobenzonitrile as substrate); (c) the TEM image of porous NiCo bimetallic catalyst after five cycles; (d) the XPS spectra of porous NiCo bimetallic catalyst after five cycles. | |
4. Conclusions
In conclusion, the convenient and effective preparation strategy for the bimetallic NiCo porous materials has been developed. Through the introduction of Ni(NO3)2 as etching agents, the ZIF-67 polyhedrons were transformed into hollow nanospheres, and irregular nanosheets with further increasing the amount of Ni salt. The bimetallic NiCo phase was formed after pyrolysis in nitrogen atmosphere at high temperature, with the decomposition and releasing of organic ligand into gaseous molecule under flowing nitrogen. The as-synthesized bimetallic NiCo porous materials show superior activity towards the hydrolytic dehydrogenation of AB. The application of AB dehydrogenation in hydrogenation reaction has perfectly realized the chemo-selective reduction of nitrobenzene with reducible functional groups. The etching through metal salt and subsequent pyrolysis at high temperature is a novel and promising strategy for the construction of porous metal materials in catalysis.
Conflicts of interest
There are no conflicts to declare.
Acknowledgements
This work was supported by the National Natural Science Foundation of China (21402029), Natural Science Foundation of Higher Education Institutions in Anhui Province (KJ2018A0332, KJ2018A0340), Natural Science Foundation of Anhui Province (1608085MB34), FYNC and Government Cooperation Foundation (XDHX2016013), and Students Research Training Program (201810371053).
Notes and references
- K. Shen, L. Chen, J. Long, W. Zhong and Y. Li, ACS Catal., 2015, 5, 5264–5271 CrossRef CAS.
- M. Huang, K. Mi, J. Zhang, H. Liu, T. Yu, A. Yuan, Q. Kong and S. Xiong, J. Mater. Chem. A, 2017, 5, 266–274 RSC.
- Y. Q. Yang, H. Dong, Y. Wang, C. He, Y. X. Wang and X. D. Zhang, J. Solid State Chem., 2018, 258, 582–587 CrossRef CAS.
- T. Zeng, M. Yu, H. Zhang, Z. He, J. Chen and S. Song, Catal. Sci. Technol., 2017, 7, 396–404 RSC.
- Q. Zhou and Y. Wu, Chin. Sci. Bull., 2018, 63, 2246–2267 CrossRef.
- B. Liu, H. Shioyama, T. Akita and Q. Xu, J. Am. Chem. Soc., 2008, 130, 5390–5391 CrossRef CAS PubMed.
- S. Hong, J. Yoo, N. Park, S. M. Lee, J.-G. Park, J. H. Park and S. U. Son, Chem. Commun., 2015, 51, 17724–17727 RSC.
- X. Wang, H. Xiao, A. Li, Z. Li, S. Liu, Q. Zhang, Y. Gong, L. Zheng, Y. Zhu, C. Chen, D. Wang, Q. Peng, L. Gu, X. Han, J. Li and Y. Li, J. Am. Chem. Soc., 2018, 140, 15336–15341 CrossRef CAS PubMed.
- H. Hu, L. Han, M. Yu, Z. Wang and X. W. Lou, Energy Environ. Sci., 2016, 9, 107–111 RSC.
- P. Yin, T. Yao, Y. Wu, L. Zheng, Y. Lin, W. Liu, H. Ju, J. Zhu, X. Hong, Z. Deng, G. Zhou, S. Wei and Y. Li, Angew. Chem., Int. Ed., 2016, 55, 10800–10805 CrossRef CAS.
- J. Yang, F. Zhang, X. Wang, D. He, G. Wu, Q. Yang, X. Hong, Y. Wu and Y. Li, Angew. Chem., Int. Ed., 2016, 55, 12854–12858 CrossRef CAS.
- S. Dang, Q.-L. Zhu and Q. Xu, Nat. Rev. Mater., 2017, 3, 17075–17088 CrossRef.
- G. Zhong, D. Liu and J. Zhang, J. Mater. Chem. A, 2018, 6, 1887–1899 RSC.
- H. Wu, X. Qian, H. Zhu, S. Ma, G. Zhu and Y. Long, RSC Adv., 2016, 6, 6915–6920 RSC.
- Y.-X. Zhou, Y.-Z. Chen, L. Cao, J. Lu and H.-L. Jiang, Chem. Commun., 2015, 51, 8292–8295 RSC.
- K. Shen, L. Chen, J. Long, W. Zhong and Y. Li, ACS Catal., 2015, 5, 5264–5271 CrossRef CAS.
- B. You, N. Jiang, M. Sheng, S. Gul, J. Yano and Y. Sun, Chem. Mater., 2015, 27, 7636–7642 CrossRef CAS.
- N. Ono, The Nitro Group in Organic Synthesis, Wiley-VCH, New York, 2011 Search PubMed.
- X. Wang, N. Perret, L. Delannoy, C. Louis and M. A. Keane, Catal. Sci. Technol., 2016, 6, 6932–6941 RSC.
- M. Zhao, K. Deng, L. He, Y. Liu, G. Li, H. Zhao and Z. Tang, J. Am. Chem. Soc., 2014, 136, 1738–1741 CrossRef CAS PubMed.
- C. Lian, H. Liu, C. Xiao, W. Yang, K. Zhang, Y. Liu and Y. Wang, Chem. Commun., 2012, 48, 3124–3126 RSC.
- X. Chen, K. Shen, D. Ding, J. Chen, T. Fan, R. Wu and Y. Li, ACS Catal., 2018, 8, 10641–10648 CrossRef CAS.
- Q. Wei, Q. Ma, P. Zuo, H. Fan, S. Qu and W. Shen, ChemCatChem, 2018, 10, 1019–1026 CrossRef CAS.
- F. A. Westerhaus, R. V. Jagadeesh, G. Wienhöfer, M.-M. Pohl, J. Radnik, A.-E. Surkus, J. Rabeah, K. Junge, H. Junge, M. Nielsen, A. Brückner and M. Beller, Nat. Chem., 2013, 5, 537 CrossRef CAS PubMed.
- R. V. Jagadeesh, A.-E. Surkus, H. Junge, M.-M. Pohl, J. Radnik, J. Rabeah, H. Huan, V. Schünemann, A. Brückner and M. Beller, Science, 2013, 342, 1073–1076 CrossRef CAS PubMed.
- T. Schwob and R. Kempe, Angew. Chem., Int. Ed., 2016, 55, 15175–15179 CrossRef CAS PubMed.
- R. V. Jagadeesh, T. Stemmler, A.-E. Surkus, H. Junge, K. Junge and M. Beller, Nat. Protoc., 2015, 10, 548–557 CrossRef CAS PubMed.
- L. Liu, P. Concepción and A. Corma, J. Catal., 2016, 340, 1–9 CrossRef CAS.
- B. Chen, F. Li, Z. Huang and G. Yuan, ChemCatChem, 2016, 8, 1132–1138 CrossRef CAS.
- Z. Yu, Z. Chen, Y. Chen, Q. Peng, R. Lin, Y. Wang, R. Shen, X. Cao, Z. Zhuang and Y. Li, Nano Res., 2018, 11, 3730–3738 CrossRef CAS.
- H.-L. Jiang and Q. Xu, Catal. Today, 2011, 170, 56–63 CrossRef CAS.
- T. Umegaki, Q. Xu and Y. Kojima, Materials, 2015, 8, 4512–4534 CrossRef CAS PubMed.
- S. De, J. Zhang, R. Luque and N. Yan, Energy Environ. Sci., 2016, 9, 3314–3347 RSC.
- Q. Liu, S. Zhang, J. Liao, X. Huang, Y. Zheng and H. Li, Catal. Sci. Technol., 2017, 7, 3573–3579 RSC.
- A. M. Al-Enizil, R. M. Brooks, M. M. Ahmad, M. M. Ei-Halwany, M. H. El-Newehy and A. Yousef, J. Nanosci. Nanotechnol., 2018, 18, 4714–4719 CrossRef PubMed.
- X. Ma, Y.-X. Zhou, H. Liu, Y. Li and H.-L. Jiang, Chem. Commun., 2016, 52, 7719–7722 RSC.
- J. Liao, D. Lu, G. Diao, X. Zhang, M. Zhao and H. Li, ACS Sustainable Chem. Eng., 2018, 6, 5843–5851 CrossRef CAS.
- H. Wang, D. Gao, L. Wang, Y. Chi, M. Wang, Y. Gu, C. Wang and Z. Zhao, Catal. Lett., 2018, 148, 1739–1749 CrossRef CAS.
- Y. X. Liu, X. W. Liu, Q. C. Feng, D. S. He, L. B. Zhang, C. Lian, R. A. Shen, G. F. Zhao, Y. J. Ji, D. S. Wang, G. Zhou and Y. D. Li, Adv. Mater., 2016, 28, 4747–4754 CrossRef CAS PubMed.
- T. Umegaki, Q. Xu and Y. Kojima, Materials, 2015, 8, 4512–4534 CrossRef CAS.
- N. Asao, Y. Ishikawa, N. Hatakeyama, Menggenbateer, Y. Yamamoto, M. Chen, W. Zhang and A. Inoue, Angew. Chem., Int. Ed., 2010, 49, 10093–10095 CrossRef CAS PubMed.
- A.-Y. Yin, X.-Y. Guo, W.-L. Dai and K.-N. Fan, Green Chem., 2009, 11, 1514–1516 RSC.
- X. Kong, C. Ma, J. Zhang, J. Sun, J. Chen and K. Liu, Appl. Catal., A, 2016, 509, 153–160 CrossRef CAS.
- C. Chen, C. Y. Nan, D. S. Wang, Q. A. Su, H. H. Duan, X. W. Liu, L. S. Zhang, D. R. Chu, W. G. Song, Q. Peng and Y. D. Li, Angew. Chem., Int. Ed., 2011, 50, 3725–3729 CrossRef CAS PubMed.
- J. Ying, X.-Y. Yang, G. Tian, C. Janiak and B.-L. Su, Nanoscale, 2014, 6, 13370–13382 RSC.
Footnote |
† Electronic supplementary information (ESI) available. See DOI: 10.1039/c9ra01551e |
|
This journal is © The Royal Society of Chemistry 2019 |
Click here to see how this site uses Cookies. View our privacy policy here.