DOI:
10.1039/C9RA01584A
(Paper)
RSC Adv., 2019,
9, 16130-16135
High proton conductivity behavior in a 2D metal sulfite constructed from a histidine ligand†
Received
2nd March 2019
, Accepted 22nd April 2019
First published on 22nd May 2019
Abstract
In the presence of the amino acid histidine, an inorganic–organic hybrid metal sulfite, Zn2(SO3)2(C6N3O2H9)2·H2O (1), has been prepared under hydrothermal conditions. Single-crystal X-ray diffraction analysis reveals that 1 shows a 2D layer framework built up from a classical second building unit (S4R), and bridged histidine molecule. Notably, it is the first report of a metal sulfite in the presence of an amino acid molecule. A 1D H-bonding array can be constructed by the H-bonding interaction between histidine molecules and sulfite groups. Moreover, a new function of metal sulfite for proton conduction was investigated by alternating-current impedance analysis. The results demonstrate that compound 1 shows a high proton conductivity of approximately 10−3 S cm−1 at 348 K and 98% relative humidity.
Introduction
Proton-conducting materials have received extensive attention because of their applications in a variety of electrical and electrochemical devices, especially in fuel cells.1–4 Nafion is one of the most important popular materials that is being used as an electrolyte membrane with a high proton conductivity of 10−2 S cm−1 at 60–80 °C under 98% relative humidity (RH).5 However, this material suffers from several limitations, such as high cost, limited operating temperature and the difficulty of optimizing the proton transport pathway.6 Therefore, it is necessary to design and synthesize new materials which maintain high proton conductivity with low cost and a wide operating temperature.
Crystalline porous materials, with framework diversity, tunability and porosity, have become ideal candidates for proton-conducting applications. In addition, they provide theoretical guidance for the synthesis of new proton-conducting materials because the crystalline nature of crystalline porous materials provides an opportunity to study the proton-conduction pathway and mechanism.7–11 Inorganic open-framework materials based on oxyanions, such as phosphates,12,13 phosphites,14–16 and sulfates,17,18 exhibit well-defined pore architectures and variable framework compositions. On the one hand, proton carriers (e.g., H3O+, NH4+, imidazole, H2O and –COOH) can be easily introduced into their free voids or in their frameworks, which is an effective approach to prepare high performance proton-conducting materials.19–22 On the other hand, the hydrogen bond networks between host–guest or guest–guest can provide proton-conducting pathways.23–27 In addition, high hydrothermal stability which is very important for proton-conducting materials in fuel cells is usually another significant feature of these materials.24,28 Current endeavors on the study of proton-conducting materials have demonstrated that metal phosphates, phosphites and sulfates show proton conduction.29–35 For example, [{In2(μ-OH)2(SO4)4}·{(LH)4}·nH2O]n (LH = protonated melamine) carries a continuous water array functioning as a solid-state proton conductor (σ = 4.4 × 10−5 S cm−1, 98% RH, and T = 303.15 K), a three-dimensional beryllium phosphate with intersecting 24-membered ring channels displays exceptional hydrothermal stability and shows a high proton conductivity on the order of 10−3 S cm−1 at 25 °C under high humidity conditions.34,35
We are particularly interested in the use of 3-connected centers as basic structural units for the construction of inorganic open-framework materials, such as a chiral indium phosphite with intertwined host and guest helices and a layered cobalt phosphite-oxalate with proton conductivity.36,37 In order to expand the field of research concerning inorganic open-framework materials with 3-connected centers as basic structural units, we try to introduce sulfite into the inorganic framework. However, because of the instability of S in its +4 oxidation state, sulfates are usually obtained as products in most of the reactions even when SO32− is used as the S source. Probably due to this difficulty, there are few open-framework metal sulfites known.38–40 Herein, we use histidine as the organic template to synthesize a metal sulfite. Histidine molecules have a variety of coordination modes, and each functional group can be bonded to a metal center, wherein carboxylic acid is often introduced as a functional group capable of improving proton conduction properties in a proton conductive material, and a side chain imidazole is a common ligand in metal inorganic–organic hybrid materials, these functional groups can enhance proton conduction efficiency in theory.8–11
In this paper, a 2D layered zinc sulfite Zn2(SO3)2(C6N3O2H9)2·H2O with a histidine molecule as the ligand has been hydrothermally synthesized, which is the first report of a metal sulfite in the presence of an amino acid molecule. Notably, this compound shows a high proton conductivity in the order of 10−3 S cm−1 at 348 K and 98% RH.
Experimental section
Materials and instrumentation
All chemical samples were obtained from commercial sources and used without further purification. The X-ray diffraction (XRD) pattern was obtained with a Bruker D8 Advance diffractometer with Cu-Kα (λ = 1.5418 Å, 40 kV, 40 mA) radiation in the scan range of 4–40° with a step size of 0.02°. The elemental analysis was conducted on a Vario EL cube elemental analyzer. ICP-AES (inductively coupled plasma-atomic emission spectroscope) analysis was performed on a PerkinElmer Optima 3300DV ICP instrument. FT-IR spectra was recorded on a Nicolet Impact 410 spectrometer between 400 and 4000 cm−1 using the KBr pellet method. Thermogravimetric analysis (TGA) was conducted on a PerkinElmer TGA 7 thermogravimetric analyzer at a ramp rate of 10 °C min−1 under a flow of air gas from room temperature to 800 °C. Photoluminescence analyses were performed on an Edinburgh Instrument FLS920 luminescence spectrometer.
Synthesis
The zinc sulfite compound, Zn2(SO3)2(C6N3O2H9)2·H2O, was synthesized from Zn(Ac)2·4H2O, NaHSO3, and L-histidine in a H2O solution. A mixture of 0.1 g Zn(Ac)2·4H2O, 0.4 g sodium hydrogen sulfite (NaHSO3), and 0.2 g L-histidine was dissolved in 2.0 mL of water. The mixture was further stirred for 30 minutes at room temperature and heated at 115 °C for 5 days in a 23 ml Teflon-lined stainless steel autoclave (filled up to 27% volume capacity), followed by slow cooling down to the ambient temperature. Colorless crystals could be achieved, washed with water, then filtered, and dried in air. The yield of the product was 56% in weight based on zinc. The experimental XRD pattern are in good agreement with the simulated ones from the single-crystal X-ray diffraction (Fig. S1†). Analysis found (wt%): Zn, 21.07; S, 10.25; C, 23.16; H, 3.18; N, 13.65; calcd (wt%): Zn, 21.12; S, 10.36; C, 23.27; H, 3.26; N, 13.57. IR data (KBr, cm−1): 3133 m, 3037 m, 2910 m, 1644 s, 1585 s, 1409 m, 1367 w, 1292 w, 1267 w, 1230 w, 1145 w, 1101 w, 1037 w, 937 s, 900 m, 838 w, 817 w, 784 w, 721 w, 692 m, 632 m, 547 w, 507 m, 440 w.
Determination of crystal structure
A suitable single crystal with dimensions of 0.20 × 0.21 × 0.22 mm3 of compound 1 was carefully selected under an optical microscope for single crystal XRD analysis. Single-crystal structure determination by X-ray diffraction was performed on a Bruker D8 Quest diffractometer with graphite-monochromated Mo-Kα (λ = 0.71073 Å) radiation at a temperature of 296 K. Data processing was accomplished with the saint-processing program. The structure was solved by a direct method using the SHELXTL-2014 crystallographic software package.41,42 The zinc and sulphur atoms were first located, whereas the carbon, nitrogen and oxygen atoms were found in the different Fourier maps. All the hydrogen atoms were placed geometrically and refined in a riding model. All of the non-hydrogen atoms were refined anisotropically.
The detailed crystallographic data and selected bond lengths as well as the bond angles for compound 1 are listed in Table 1 and S1.† CCDC-1892957 contains the supplementary crystallographic data for this paper.
Table 1 Crystal data and structure refinement for compound 1
Empirical formula |
C12H20N6O11S2Zn2 |
Formula weight |
619.26 |
Temperature/K |
296(2) |
Crystal system |
Monoclinic |
Space group |
C2/c |
a/Å |
14.6764(7) |
b/Å |
8.3407(4) |
c/Å |
16.8021(8) |
β/° |
101.397(2) |
V/Å3 |
2016.21(17) |
Z |
4 |
Calculated density/g cm−3 |
2.040 |
Absorption coefficient/mm−1 |
2.660 |
F(000) |
1256 |
Theta range for data collection/° |
2.5 to 25.1 |
Refinement method/unique |
8485/1782 [R(int) = 0.034] |
Refinement method |
Full-matrix least-squares on F2 |
Data/restraints/parameters |
1782/0/151 |
Goodness-of-fit on F2 |
1.05 |
Final R indices [I > 2 sigma(I)] |
R1 = 0.0258, wR2 = 0.0574 |
R indices (all data) |
R1 = 0.0350, wR2 = 0.0608 |
Largest diff. peak and hole/(e Å−3) |
0.32 and −0.28 |
Proton conductivity measurement
The hydrated proton conductivities of the compound pellets were measured using a CHI660E electrochemical work station. The alternating current frequencies ranged from 1 Hz to 106 Hz. Variable impedance spectra were collected at different humidity and temperatures. The humidity was controlled by different saturated aqueous salt solutions, which were salt solutions of Na2HPO4 (98% RH), NaCl (76% RH), NaBr (58% RH) and K2CO3 (44% RH). The conductivity measurement was performed after setting the sample at different humidities for approximately 48 hours. The proton conductivity (S cm−1) was calculated using the formula σ = L/AR, where L is the pellet thickness, A is the pellet area (7 mm in diameter and 0.8 mm in thickness), and R is the compound impedance obtained from the Nyquist plot. The Ea value was derived from the Arrhenius equation: Tσ = σ0
exp(−Ea/kT), where σ0 is a pre-exponential factor, Ea is the activation energy, and k is the Boltzmann constant.
Results and discussion
Structural description
The single crystal X-ray analysis reveals that 1 crystallizes in the monoclinic crystal system with the C2/c space group. The asymmetric unit contains one Zn atom, one S atom, one L-histidine cation and one free lattice water molecule (Fig. S3†). The crystallographically independent Zn atom is tetrahedrally coordinated to two O atoms bridging to the neighboring S atoms, one carboxyl O atom and one N atom from the imidazole of the L-histidine cations. The Zn–O bond lengths are in the range of 1.9247(18)–1.9853(19) Å and the O–Zn–O bond angles vary in the range of 102.49(8)–112.68(8)°. The Zn–N bond length is 1.989(2) Å. The crystallographically independent S atom is three-coordinated to oxygen atoms and shares two oxygen atoms with adjacent Zn atoms, with the third trigonal pyramidal vertex occupied by an terminal oxygen atom [d (S
O) = 1.5167(18) Å]. The S–O bridging bond lengths are in the range of 1.5167(18)–1.5362(19) Å and the average O–S–O bond angle is 104.83°.
As shown in Fig. 1a, the framework of Zn2(SO3)2(C6N3O2H9)2·H2O shows a 2D layered structure, which is built up from a second building unit, namely a single four-membered ring (S4R) and a histidine molecule. The S4R, (ZnSO3)2, is formed from two tetrahedral Zn2+ cations and two SO32− sulfite anions. The histidine molecule is neutral zwitterionic and serves as a bidentate ligand connecting with the adjacent two S4Rs to form a 2D inorganic–organic hybrid layer. In the layer, six Zn(II) ions, two sulfites and four histidine molecules get together and form a 12-membered ring window.
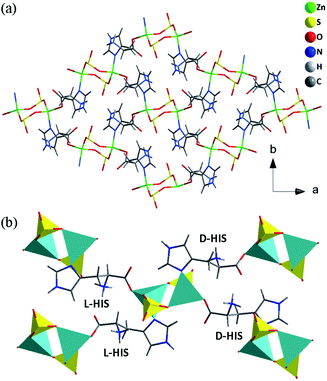 |
| Fig. 1 The structure of compound 1: (a) the 2D layer with the 12-membered ring window. (b) The connection between histidine molecules and S4Rs (HIS: histidine). | |
As shown in Fig. 1b, each S4R is surrounded by two pairs of histidine molecules while each histidine molecule is connected with the adjacent two S4Rs. It is noteworthy that the histidine molecules surrounding the S4R show two different absolute configurations. A pair of histidine molecules on one side of the S4R has the R absolute configuration while the other pair of those on the other side of the S4R has the S absolute configuration. As a result, compound 1 is achiral, although the initially added histidine is homochiral. It is demonstrated that the racemization happened in the hydrothermal synthetic system. A similar phenomenon was observed in the synthesis of Zn(HPO4)(C6H9N3O2), Zn(HPO3)(DL-C6H9N3O2)(H2O)0.5 and ZnPO-CJ36.43–45 In order to contrast experiment results, we used D,L-histidine and D-histidine to replace the L-histidine in the system of reaction, but the final structure is not charged and it is still compound 1. The result further demonstrated that a single chiral histidine does not easily exist in the final structure under hydrothermal synthesis.
As shown in Fig. 2, the 2D layers are stacked in an ABAB sequence along the a axis. The lattice water molecules are situated in between the layers, which are held via extensive O(W)–H⋯O hydrogen bonding interactions between guest molecules and the host framework. The O⋯O distance is 2.83 Å. The H bond provides extra stability to the lattice water molecules, which has been confirmed by the TGA of the powder sample of compound 1 showing a continuous initial loss.34 Extensive hydrogen bonds are also formed between the histidine molecules and sulfite units. The N(2)H3− group forms three hydrogen bonds to one terminal C
O group (dN···O = 2.848(3) Å), two terminal oxygen atoms from sulfite units with an average N⋯O distance of 2.82 Å. The N(3)H− groups of the imidazole moiety form one hydrogen bond with the terminal O(3) atom from the sulfite unit with a distance of 2.791(3) Å. In addition, there are three types of weak C–H⋯O hydrogen-bonding interactions: the interaction between the chiral tertiary carbon C(2) atom and the μ2-O(4) of the carboxyl oxygen atoms, the interaction between the C(5) atom from imidazole and the terminal O(5) of the carboxyl oxygen atoms, and the interaction between the C(6) atom from imidazole and μ2-O(2) of the sulfite group. Because of the H-bond interactions between the histidine molecules and sulfite groups, a 1D H-bonding array can be constructed (Fig. S4†). Finally, the hydrogen bonding interactions among water molecules, histidine molecules and sulfite units construct a pseudo-3D supramolecular structure (Fig. 3). The detailed H-bond data is listed in Table S2.†
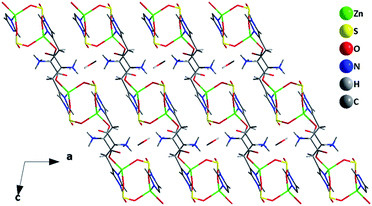 |
| Fig. 2 The 2D layers are stacked in an ABAB sequence along the a axis. | |
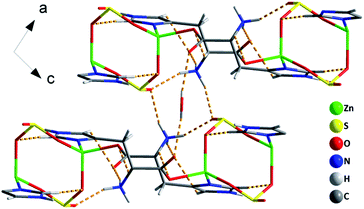 |
| Fig. 3 View of H-bonding interactions existing in the structure of 1. | |
As far as we know, compound 1 is the first zinc sulfite to be prepared in the presence of a histidine molecule. The histidine acts as a bridge between inorganic secondary building blocks which directly cross-link to the framework. In the past, although some zinc sulfite frameworks are already reported such as (NH3CH2CH2NH3)[Zn3(SO3)4],40 the structure of cross-linking organic metal-sulfites has been rarely reported.
Proton conductivity
In the structure of Zn2(SO3)2(C6N3O2H9)·H2O, there are water molecules and a 1D H-bonding array. Therefore, compound 1 is expected to conduct protons and proton conduction behavior was investigated by AC impedance measurement both as a function of temperature at constant RH and as a function of RH at constant temperature. The proton conductivity was estimated from the Nyquist plots shown in Fig. 4, 5 and S5–S7.† The proton conductivity of different relative humidity (RH) at 303 K was measured. It was demonstrated that the proton conductivity increases with the increase of humidity (Fig. 4). This phenomenon is well proven at low temperatures, the proton conductivity is 2.05 × 10−9 S cm−1 under 44% RH and reaches 1.01 × 10−5 S cm−1 under 98% RH. Even though the proton conductivity of 1 was not remarkable at room temperature and 98% RH, it is comparable to several materials such as [Zn(D-LCl)(Cl)](H2O)2 (4.42 × 10−5 S cm−1 at 304 K, 98% RH), [H3O][Ni(SO4)F] (7.9 × 10−6 S cm−1 at 298 K, 98% RH) and [{In2(OH)2(SO4)4}·{(LH)4}·nH2O]n (4.4 × 10−5 S cm−1 at 303 K, 98% RH).34,46 As shown in Fig. S5–S7,† we found that at high temperature under humid conditions, the semicircle of the Nyquist plot basically disappears, which is similar to the phenomenon reported previously in the literature.47–49
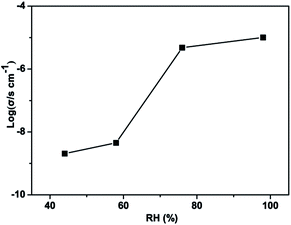 |
| Fig. 4 Humidity dependence of the proton conductivity at 303 K. | |
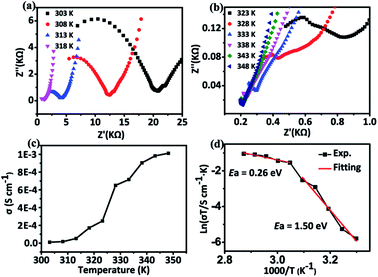 |
| Fig. 5 (a) and (b) Nyquist plots of 1 at various temperatures under 98% RH. (c) Temperature dependence of the proton conductivity of 1. (d) Arrhenius plot of 1. | |
To gain insight into the proton-conduction mechanism, we calculated the activation energy at different temperatures under 98% RH (Fig. 5). It is obvious that the resistance of the compound decreases with increasing temperature, indicating a sustained increase in proton conductivity. The proton conductivity increased from 1.01 × 10−5 S cm−1 at 303 K to 2.49 × 10−4 S cm−1 at 323 K. It is worth noting that the conductivity has a jump in growth around 323 K, and a maximum value is reached 1.01 × 10−3 S cm−1 at 348 K. This value was comparable to some MOFs such as [LnL(H2O)3]·2H2O (L = N-phenyl-N′-phenylbicyclo-[2,2,2]-oct-7-ene-2,3,5,6-tetra-carboxdiimide tetracarboxylic acid, Ln = Eu, Dy) (10−5 S cm−1, 343 K, 97% RH), POMs such as NENU-530 (10−3 S cm−1, 343 K, 98% RH), metal phosphates such as (NH4)2Al4(PO4)4(HPO4)·H2O (10−4 S cm−1, 343 K, 99% RH) and metal phosphites, like (NH4)0.59(H3O)1.41Mn5(HPO3)6 (10−4 S cm−1, 328 K, 98% RH).47–55 By linearly fitting the relationship between ln(σT) and 1000/T, the activation energy of proton transport is estimated to be 1.50 eV at 98% RH between 303 K and 323 K while the Ea is 0.26 eV between 328 K to 348 K (Fig. 5d). Therefore, the above results suggest that the vehicle mechanism and the Grotthuss mechanism should exist in the temperature range of 303–348 K according to the value of Ea (vehicle mechanism: Ea > 0.4 eV; Grotthuss mechanism: Ea < 0.4 eV).2,56 The proton conduction pathway could be further understood by analyzing the structure of the compound and the Ea value. As shown in Fig. S4,† a 1D H-bonding array can be constructed because of the H-bond interactions between histidine molecules and sulfite groups, which should be active in promoting a Grotthuss type conduction. In the temperature range of 328–348 K, the Ea was measured to be 0.26 eV, which is quite a low value suggesting probably the Grotthuss mechanism. However, the activation energy of compound 1 is higher at low temperatures (303–323 K), the Ea is estimated to be 1.50 eV, showing that the proton transport process follows the vehicle mechanism, proton conduction may be due to the diffusion or transfer of crystalline water molecules between layers, which requires a higher activation energy.2 A similar phenomenon has been reported in a lanthanide carbonate cluster based MOF with the formula {[Gd2(CO3)(ox)2(H2O)2]·3H2O}n.57
To further investigate the humidity and temperature stability of compound 1, we performed a PXRD test on samples after the electrochemical test under different humidity and temperature conditions. As shown in Fig. 6, the structure of compound 1 did not change even after electrochemical testing under conditions of 348 K and 98% RH, indicating that compound 1 has good structural stability, and this property is valuable in practical applications.
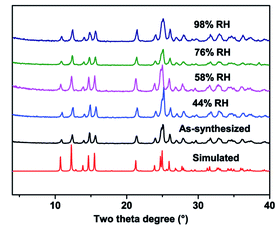 |
| Fig. 6 PXRD patterns of simulated, as-synthesized, and after impedance test under different humidity phases of compound 1. | |
TGA analysis
Thermogravimetric analysis carried out in the air at a heating rate of 10 °C min−1 up to temperature of 800 °C. The continuous two-step weight loss was observed in the range of 220–673 °C. The total observed weight loss of 71.94 wt% is attributed to the removal of water molecules (calcd 2.91 wt%) and the decomposition of L-histidine (calcd 50.11 wt%) and the sulfur dioxide molecule (calcd 20.67 wt%) in the framework (Fig. S8†).
Photoluminescence spectrum
The photoluminescence spectra of 1 and the histidine molecules were measured in a solid state at room temperature (Fig. S9†). The emission spectrum of Zn2(SO3)2(C6N3O2H9) showed a peak at 465 nm and excited at a wavelength of 407 nm, red-shifted compared to pure L-histidine. The reason is that the coordination between histidine and zinc ions effectively increases the rigidity of the ligand and reduces the energy loss through non-radiative thermal vibration.58–61
Conclusions
In summary, a two-dimensional layered zinc sulfite coordinated by histidine is synthesized under hydrothermal conditions. Notably, an amino acid is firstly introduced into the family of metal sulfites. Proton conductivity investigations on the title compound have been carried out and the proton transmission rate was estimated to be 10−3 S cm−1 at 348 K and 98% humidity. Additionally, the vehicle and Grotthuss proton conduction mechanisms are realized at 303–323 K and 328–348 K, respectively, which can be attributed to the transfer of crystalline water molecules and the 1D H-bonding array in the framework. Our investigation further exemplifies the possibility of constructing a metal sulfite with biofunctional molecules and exploring a new proton-conducting material in metal sulfite systems. Further work on the syntheses of such metal sulfite analogues for proton conduction is in progress.
Conflicts of interest
There are no conflicts to declare.
Acknowledgements
We thank National Natural Science Foundation of China (No. 21201095, 51663020 and 21301170), Educational Bureau of Liaoning Province for the Fundamental Research of Key Lab (No. L2017LZD002) and the National Science Fund for Distinguished Young Scholars of Guangxi Province (No. 2017JJG150011) for financial support.
References
- J. A. Hurd, R. Vaidhyanathan, V. Thangadurai, C. I. Ratcliffe, I. L. Moudrakovski and G. K. Shimizu, Nat. Chem., 2009, 1, 705–710 CrossRef CAS PubMed.
- X. Meng, H.-N. Wang, S.-Y. Song and H.-J. Zhang, Chem. Soc. Rev., 2017, 46, 464–480 RSC.
- X. Guan, Y. Ma, H. Li, Y. Yusran, M. Xue, Q. Fang, Y. Yan, V. Valtchev and S. Qiu, J. Am. Chem. Soc., 2018, 140, 4494–4498 CrossRef CAS PubMed.
- M. Inukai, S. Horike, T. Itakura, R. Shinozaki, N. Ogiwara, D. Umeyama, S. S. Nagarkar, Y. Nishiyama, M. Malon, A. Hayashi, T. Ohhara, R. Kiyanagi and S. Kitagawa, J. Am. Chem. Soc., 2016, 138, 8505–8511 CrossRef CAS PubMed.
- K. M. Mauritz and R. B. Moore, Chem. Rev., 2004, 104, 4535–4585 CrossRef CAS PubMed.
- G. Alberti and M. Casciola, Solid State Ionics, 2001, 145, 3–16 CrossRef CAS.
- F. Yang, G. Xu, Y. Dou, B. Wang, H. Zhang, H. Wu, W. Zhou, J.-R. Li and B. Chen, Nat. Energy, 2017, 2, 877–883 CrossRef CAS.
- M. Sadakiyo, T. Yamada and H. Kitagawa, ChemPlusChem, 2016, 81, 691–701 CrossRef CAS.
- A. Shigematsu, T. Yamada and H. Kitagawa, J. Am. Chem. Soc., 2011, 133, 2034–2036 CrossRef CAS PubMed.
- Y. Ye, L. Zhang, Q. Peng, G. Wang, Y. Shen, Z. Li, L. Wang, X. Ma, Q.-H. Chen, Z. Zhang and S. Xiang, J. Am. Chem. Soc., 2015, 137, 913–918 CrossRef CAS PubMed.
- S. Bureekaew, S. Horike, M. Higuchi, M. Mizuno, T. Kawamura, D. Tanaka, N. Yanai and S. Kitagawa, Nat. Mater., 2009, 8, 831–836 CrossRef CAS PubMed.
- T. Su, H. Xing, J. Xu, J. Yu and R. Xu, Inorg. Chem., 2011, 50, 1073–1078 CrossRef CAS PubMed.
- G.-M. Wang, J.-H. Li, X. Zhang, J.-Q. Jiao, Z.-Z. Bao, X.-M. Zhao, W.-W. Jiang, Y.-X. Wang and J.-H. Lin, Inorg. Chem. Commun., 2014, 46, 295–300 CrossRef CAS.
- P. Ramaswamy, S. Mandal and S. Natarajan, Inorg. Chim. Acta, 2011, 372, 136–144 CrossRef CAS.
- K. Wang, Y. Bian, J. Li, D. Xu and Z. Lin, Inorg. Chem., 2016, 55, 3727–3729 CrossRef CAS PubMed.
- Z.-Z. Xue, J. Pan, J.-H. Li, Z.-H. Wang and G.-M. Wang, J. Mol. Struct., 2017, 1138, 1–5 CrossRef CAS.
- S. R. Marri, S. Mahana, D. Topwal and J. N. Behera, Dalton Trans., 2017, 46, 1105–1111 RSC.
- K. Wang, D. Luo, D. Xu, F. Guo, L. Liu and Z. Lin, Dalton Trans., 2014, 43, 13476–13479 RSC.
- X. Zhao, C. Mao, X. Bu and P. Feng, Chem. Mater., 2014, 26, 2492–2495 CrossRef CAS.
- S. Horike, D. Umeyama, M. Inukai, T. Itakura and S. Kitagawa, J. Am. Chem. Soc., 2012, 134, 7612–7615 CrossRef CAS PubMed.
- M. Sadakiyo, T. Yamada, K. Honda, H. Matsui and H. Kitagawa, J. Am. Chem. Soc., 2014, 136, 7701–7707 CrossRef CAS PubMed.
- A. Mallick, T. Kundu and R. Banerjee, Chem. Commun., 2012, 48, 8829–8831 RSC.
- T. Kundu, S. C. Sahoo and R. Banerjee, Chem. Commun., 2012, 48, 4998–5000 RSC.
- M. Wei, X. Wang and X. Duan, Chem.–Eur. J., 2013, 19, 1607–1616 CrossRef CAS PubMed.
- S. S. Nagarkar, S. M. Unni, A. Sharma, S. Kurungot and S. K. Ghosh, Angew. Chem., Int. Ed., 2014, 53, 2638–2642 CrossRef CAS PubMed.
- G.-L. Zheng, G.-C. Yang, S.-Y. Song, X.-Z. Song and H.-J. Zhang, CrystEngComm, 2014, 16, 64–68 RSC.
- M. Bazaga-Garcia, R. M. P. Colodrero, M. Papadaki, P. Garczarek, J. Zon, P. Olivera-Pastor, E. R. Losilla, L. Leon-Reina, M. A. G. Aranda, D. Choquesillo-Lazarte, K. D. Demadis and A. Cabeza, J. Am. Chem. Soc., 2014, 136, 5731–5739 CrossRef CAS PubMed.
- G.-G. Cao, J.-D. Liu, T.-T. Zhuang, X.-H. Cai and S.-T. Zheng, Chem. Commun., 2015, 51, 2048–2051 RSC.
- M. Wang, H.-B. Luo, J. Zhang, S.-X. Liu, C. Xue, Y. Zou and X.-M. Ren, Dalton Trans., 2017, 46, 7904–7910 RSC.
- H.-R. Zhao, C. Xue, C.-P. Li, K. Zhang, H.-B. Luo, S.-X. Liu and X.-M. Ren, Inorg. Chem., 2016, 55, 8971–8975 CrossRef CAS PubMed.
- J. Shi, K. Wang, J. Li, H. Zeng, Q. Zhang and Z. Lin, Dalton Trans., 2018, 47, 654–658 RSC.
- C. Guo, C. Chen, K. Wang, H. Zeng and Z. Lin, Inorg. Chem. Commun., 2017, 85, 96–99 CrossRef CAS.
- Y. Yu, J. Zhu, J. Liu, Y. Yan and X. Song, Dalton Trans., 2017, 46, 9157–9162 RSC.
- B. Manna, B. Anothumakkool, A. V. Desai, P. Samanta, S. Kurungot and S. K. Ghosh, Inorg. Chem., 2015, 54, 5366–5371 CrossRef CAS PubMed.
- K. Wang, T. Li, H. Zeng, G. Zou, Q. Zhang and Z. Lin, Inorg. Chem., 2018, 57, 8726–8729 CrossRef CAS PubMed.
- L. Huang, T. Song, Y. Fan, L. Wang, C. Ji, L. Shan and L. Wang, Microporous Mesoporous Mater., 2012, 149, 95–100 CrossRef CAS.
- F. Gao, L. Huang, Z. Xiu, Y. Yin, Y. Ma, Y. Bi and Z. Zheng, CrystEngComm, 2018, 20, 5544–5550 RSC.
- G. I. Chilas, N. Lalioti and T. Vaimakis, Dalton Trans., 2010, 39, 8296–8305 RSC.
- R. K. Tiwari and J. N. Behera, Dalton Trans., 2017, 46, 5911–5917 RSC.
- R. K. Tiwari, J. Kumar and J. N. Behera, Chem. Commun., 2016, 52, 1282–1285 RSC.
- G. M. Sheldrick, SHELXTL-NT, Version 5.1, Bruker AXS Inc., Madison, WI, 1997 Search PubMed.
- D. T. Cromer and J. T. Waber, International Tables for X-Ray Crystallography, Kynoch Press, Birmingham, AL, 1974, vol. 4, Table 2.2A Search PubMed.
- J. Fan, C. Slebodnick, R. Angel and B. E. Hanson, Inorg. Chem., 2005, 44, 552–558 CrossRef CAS PubMed.
- L. Chen and X. Bu, Chem. Mater., 2006, 18, 1857–1860 CrossRef CAS.
- L. Zhao, J. Li, P. Chen, Z. Dong, J. Yu and R. Xu, CrystEngComm, 2008, 10, 497–501 RSC.
- S. C. Sahoo, T. Kundu and R. Banerjee, J. Am. Chem. Soc., 2011, 133, 17950–17958 CrossRef CAS PubMed.
- X. Xie, S. Yu, C. Yang, J. Zhang, Z. Li and G. Li, New J. Chem., 2018, 42, 20197–20204 RSC.
- D. Umeyama, S. Horike, M. Inukai, Y. Hijikata and S. Kitagawa, Angew. Chem., Int. Ed., 2011, 50, 11706–11709 CrossRef CAS PubMed.
- W. Li, L. X. Xu, D. Luo, H. Wu, J. Tu and M. Yang, Eur. Polym. J., 2007, 43, 522–528 CrossRef CAS.
- M. Zhu, Z.-M. Hao, X.-Z. Song, X. Meng, S.-N. Zhao, S.-Y. Song and H.-J. Zhang, Chem. Commun., 2014, 50, 1912–1914 RSC.
- J. Li, X.-L. Cao, Y.-Y. Wang, S.-R. Zhang, D.-Y. Du, J.-S. Qin, S.-L. Li, Z.-M. Su and Y.-Q. Lan, Chem.–Eur. J., 2016, 22, 9299–9304 CrossRef CAS PubMed.
- C. Xue, Y. Zou, S.-X. Liu, X.-M. Ren and Z.-F. Tian, J. Solid State Chem., 2018, 258, 695–701 CrossRef CAS.
- S. D. Mikhailenko, S. Kaliaguine and E. Ghali, Microporous Mater., 1997, 11, 37–44 CrossRef CAS.
- S. Shalini, S. Aggarwal, S. K. Singh, M. Dutt, T. G. Ajithkumar and R. Vaidhyanathan, Eur. J. Inorg. Chem., 2016, 27, 4382–4386 CrossRef.
- S. Shalini, V. M. Dhavale, K. M. Eldho, S. Kurungot, T. G. Ajithkumar and R. Vaidhyanathan, Sci. Rep., 2016, 6, 32489 CrossRef CAS PubMed.
- P. Ramaswamy, N. E. Wong and G. K. H. Shimizu, Chem. Soc. Rev., 2014, 43, 5913–5932 RSC.
- Q. Tang, Y.-L. Yang, N. Zhang, S.-H. Zhang, F.-S. Tang, J.-Y. Hu, Y.-Z. Zheng and F.-P. Liang, Inorg. Chem., 2018, 57, 9020–9027 CrossRef CAS PubMed.
- S. Khatua, A. Santra, S. Padmakumar, K. Tomar and S. Konar, ChemistrySelect, 2018, 3, 785–793 CrossRef CAS.
- M. D. Allendorf, C. A. Bauer, R. K. Bhakta and R. J. Houk, Chem. Soc. Rev., 2009, 38, 1330–1352 RSC.
- L. Wen, Y. Li, Z. Lu, J. Lin, C. Duan and Q. Meng, Cryst. Growth Des., 2016, 6, 530–537 CrossRef.
- W. Feng, Y. Xu, G. Zhou, C. Zhang and X. Zheng, Inorg. Chem. Commun., 2007, 10, 49–52 CrossRef CAS.
Footnote |
† Electronic supplementary information (ESI) available: The crystallographic information files (CIF); bond lengths and angles, and hydrogen bonds. XRD, TGA, IR spectrum, photoluminescence spectrum, asymmetric unit structure, 1D H-bonding array, and Nyquist plots at various temperatures under 44% RH, 58% RH and 76% RH. CCDC 1892957. For ESI and crystallographic data in CIF or other electronic format see DOI: 10.1039/c9ra01584a |
|
This journal is © The Royal Society of Chemistry 2019 |
Click here to see how this site uses Cookies. View our privacy policy here.