DOI:
10.1039/C9RA02474C
(Paper)
RSC Adv., 2019,
9, 18429-18438
Effect of glucose oxidase and pentosanase on the prebiotic potentials of wheat arabinoxylans in an in vitro fermentation system
Received
2nd April 2019
, Accepted 3rd June 2019
First published on 11th June 2019
Abstract
Arabinoxylans (AXs) treated with enzymes, pentosanase (Pn) and glucose oxidase (GOX) not only offer a promising way to improve wheat product quality but also change their prebiotic potentials by modifying the structures of AXs. In the present study, the different crosslinking degrees of water-extracted arabinoxylans (WEAXs) treated with GOX alone or in combination with Pn + GOX were examined. The structural features and candidate prebiotic capabilities were investigated. It was demonstrated that WEAXs treated with 50 μg g−1 (w/w, enzyme/WEAX) GOX and 200 μg g−1 (w/w, enzyme/WEAX) Pn + 400 μg g−1 (w/w, enzyme/WEAX) GOX exhibited weak gel formation, while WEAXs treated with 400 μg g−1 (w/w, enzyme/WEAX) GOX and 25 μg g−1 (w/w, enzyme/WEAX) Pn + 400 μg g−1 (w/w, enzyme/WEAX) GOX formed strong gels. The ferulic acid content was significantly decreased due to the formation of ferulic acid crosslinking in the enzyme-treated WEAXs (p < 0.05). During in vitro fermentation, GOX and Pn + GOX treatments resulted in significantly (p < 0.05) increased amounts of bifidobacteria compared to WEAX alone. Pn + GOX-treated WEAXs had higher (p < 0.05) bifidobacteria populations than WEAXs treated with GOX alone. The bifidobacteria numbers and the SCFAs content of the weak gels were significantly higher than those in the strong gels under the same enzyme action (p < 0.05). These findings suggested that the increased bifidobacteria populations of GOX-treated WEAXs were due to the formation of ferulic acid crosslinking in contrast to a combination of ferulic acid crosslinking and degradation of the xylan backbone as seen in WEAXs treated with Pn + GOX. The reason the weak gels had better prebiotic potential than the corresponding strong gels was their high content of ferulic acid crosslinking.
Introduction
Several researchers have reported that sufficient daily intake of cereal fibers can effectively proliferate beneficial bacteria and improve the diversity of the intestinal flora. Arabinoxylan (AX), a major constituent of the nonstarch polysaccharides in cereal grains, is one of the most important cereal fibers and possesses health-promoting effects, such as decreasing the risk of type 2 diabetes mellitus, cardiovascular disease, and colon cancer.1,2 Several previous studies in our laboratory have shown that AXs significantly inhibit the growth of transplanted tumors and might have natural immunomodulatory activities.3,4 The health related properties of AX are presumably associated with its prebiotic potential.5,6
AX consists of a main (1→4)-linked β-D-xylopyranose backbone, which may be attached to α-L-arabinofuranose, its feruloylated derivatives, and α-D-glucuronic acid. Ferulic acid can be ester linked onto the C (O)-5 position of arabinofuranose, and it can form covalent linkages under oxidative conditions. In general, AX can be classified as either water-extractable arabinoxylan (WEAX) or water-unextractable arabinoxylan (WUAX), with the latter as the predominant form. AX plays a key role in cereal products. It has been widely accepted that WEAX has beneficial effects on bread volume and textural properties.7 However, WUAX often causes undesirable product quality because it competes for water and hinders gluten formation during the development of dough.8
To eliminate the negative effect of AX on product quality, enzymatic treatments are attractive alternatives to environmentally damaging chemical methods. The enzymatic degradation of AX with pentosanase (Pn) is a well-studied approach. There has been growing evidence that Pn can improve dough-handling properties and bread volume and softness, and change the water absorption properties of AX.9 Pn acts by cleaving the backbone of AX, solubilizing WUAX and removing AX that interfere with the formation of the gluten network, thus modifying the functionalities and structural features of AX.10 Another common approach is to incorporate oxidative enzymes, where glucose oxidase (GOX) catalyzes the formation of protein disulfide bonds to improve the dough quality.11 In addition, this can result in the oxidative crosslinking of AX or even form gels.12 Oxidative cross-linked AX has a reduced monomeric ferulic acid content and increases its molecular weight,13 affecting the viscosity and water absorption of flour and consequently bread quality.14 Recent studies have focused on the combination of Pn + GOX to improve product quality. The synergy of a combination of GOX and Pn treatment performs better than individual treatment. The mechanism of this synergy is probably due to the solubilization of WUAX, increases of glutenin macropolymers and the formation of protein–polysaccharide conjugates.15
It has been revealed that the prebiotic potential of AX could be affected by its complex structural features. AX or arabinoxylan oligosaccharides (AXOS), derived by Pn, can reduce the pH, suppress proteolytic breakdown and induce a selective bifidogenic response due to its low molecular weight.16,17 It has been shown that oxidative cross-linked AX modified with GOX has less monomeric ferulic acid content and a higher molecular weight, unlike AX modified with Pn.13 However, the prebiotic potential of WEAX modified with GOX is not clear. Furthermore, the effect of cross-linked WEAX through ferulic acid on its fermentation properties has not been studied. In addition, the fermentation characteristics of WEAX modified with a combination of Pn + GOX also warrants further investigation.
The aim of the present study was to investigate the use of GOX alone and the combination of Pn + GOX to modify WEAXs to obtain different degrees of cross-linked WEAXs. In addition, we examined the structural properties as well as the prebiotic potential of WEAXs modified with GOX alone and the combination of Pn + GOX. The findings from this research may be used to improve the quality and nutritional function of wheat products.
Materials and methods
Materials
Australian white wheat, supplied by the Beijing Gu Chuan Food Co. Ltd, was selected to obtain refined wheat flour through the use of a Bulhler Laboratory mill (MLU 202). WEAX was extracted according to the method reported by Wei Yang et al.18 Wheat flour was mixed with excess deionized water (1
:
10, w/w) for 40 min before centrifugation. The supernatant was mixed with α-amylase (BAN 480 L, Novozymes) and amyloglucosidase (AMG 300 L, Novozymes) to remove the starch. After centrifugation, ethanol was gradually added to the supernatant to a final concentration of 65%. After standing overnight, the precipitate was dissolved in deionized water, then ethanol was added as described above and the precipitates were again isolated and dried. Pentopan Mono BG (Pn, 2500 FXU-W/g) and GLUZYME Mono 10000 BG (GOX, 10
000 GODU/g) were supplied by Novozymes (China) Investment Co. Ltd. (Tianjin, China). Peroxidase (POX) was purchased from Sigma Aldrich (St. Louis, MO, USA). All other chemicals used were of analytical grade.
Preparation of the enzyme-treated AX samples
Preparation of WEAX without enzyme treatment (control). WEAX was dissolved in 10 mmol L−1 phosphate buffer solution pH 6.0 (4%, w/v).
Preparation of WEAXs treated with 50 μg g−1 (w/w, enzyme/WEAX) GOX (GOX 50) and 400 μg g−1 (w/w, enzyme/WEAX) GOX (GOX 400). Glucose (0.033 μg g−1, w/w, enzyme/WEAX) and peroxidase (4.8 PU g−1) were added to the dissolved WEAX solutions, and GOX (50 μg g−1 or 400 μg g−1, w/w, enzyme/WEAX) was used as a cross-linking agent. The solutions were allowed to react for 1 h at ambient temperature.
Preparation of WEAXs treated with Pn + GOX (Pn 25 + GOX 400 and Pn 200 + GOX 400). The dissolved WEAX solutions were incubated with different doses of Pn (25 μg g−1 and 200 μg g−1, w/w, enzyme/WEAX, for 10 min at 38 °C, which are similar to the processing conditions for when Pn is used to treat flour products) and then the solutions were heated in a boiling water bath for 10 min. Afterwards, the mixtures were treated with GOX (400 μg g−1, w/w, enzyme/WEAX) as described above.
Rheological measurements of WEAXs with enzyme treatment
The differences in the magnitude of the cross-linked WEAXs were tested by small amplitude oscillatory shearing using a strain-controlled rheometer (Discovery HR-2 rheometer, TA Instruments, New Castle, DE, USA). The samples were tested for 1 h at 25 °C at a frequency of 1.0 Hz and 1% strain.
AX content, monosaccharide composition and ferulic acid content of WEAXs with enzyme treatment
AX content in the samples was analyzed using orcinol–hydrochloric acid–ferric acid. The monosaccharide compositions of the sample were assayed by ion exchange chromatography (ICS 3000, Dionex) with a Dionex CaroPAC™ PA20 (3 × 150 mm) column. Briefly, the samples were hydrolyzed in 4 mol L−1 trichloroacetic acid at 120 °C for 2 h. The obtained hydrolysates were dried and dissolved in deionized water. The mobile phases used ultrapure water (A), 250 mmol L−1 NaOH (B) and 1 mol L−1 CH3COONa (C) with 0.5 mL min−1 under the following stepped gradient: 94% A and 6% B for 20 min, and then 89% A, 6% B and 5% C for 15 min.
Extractions of free and bound ferulic acid were carried out using the previously described methods.19 The content of ferulic acid was measured by high-performance liquid chromatography (HPLC) with a C18 column (250 × 4.6 mm; 5 μm). The mobile phases used acetonitrile (A) and 1% acetic acid (B) under the following stepped gradient: 95% B to 85% B for 30 min with 1.0 mL min−1. The peaks were monitored at 320 nm using UV spectra.
Molecular weights of WEAXs after enzyme treatment
The molecular weights of WEAX, GOX 50, and Pn 200 + GOX 400 were determined using high-performance size-exclusion chromatography with online multiangle laser light-scattering detection (HPSEC-MALLS). The eluent was 0.1 mol L−1 sodium chloride containing 0.02% NaN3 with a 0.5 mL min−1 flow rate at ambient temperature. The data were analyzed using ASTRA software (Version 5.3.4, Wyatt, Santa Barbara, CA, USA).
The microstructures of WEAXs after enzyme treatment
The surface morphology of the samples was investigated by scanning electron microscopy (SEM). The samples were frozen in liquid nitrogen and then sublimated rapidly to ensure that no water was present in the samples. The samples were coated with sputtered gold before being photographed.
In vitro human fecal fermentation
Autoclaved medium without carbohydrate was prepared before the experiments. Fecal samples were collected from three healthy donors who had not received antibiotics for at least 3 months and had no history of bowel disorders. Fecal slurries were obtained by mixing sterilized sodium phosphate buffer (0.1 M, pH 6.5) with the feces and then it was filtered to remove large particles. WEAXs were added to 2% (w/v) to the fecal slurries, which were used as blanks. Fecal slurries with 2% inulin and fructooligosaccharide (FOS) (w/v) were used as positive control samples. The culture medium (fecal slurries with or without carbohydrate) were maintained anaerobically by flushing the headspace with oxygen-free nitrogen at 37 °C for 24 h. Each fermentation was carried out in triplicate.
Analyses for fermentation products
The samples were centrifuged (10
000 × g, 20 min) to obtain the supernatants and the precipitates. The contents of SCFAs and pH were analyzed using the supernatants and the precipitates were used to analyze the bacterial populations. The values of pH were measured with a pH meter. The contents of SCFAs were measured by gas chromatography (GC) with a DB-FFAP column (15 m × 0.32 mm × 0.25 μm) after pretreatment with metaphosphoric acid.
DNA of the bacterial populations was extracted from the precipitates and the bacterial populations were quantified by quantitative real time PCR as described previously20 with a few modifications. The primers of bifidobacteria were 5′-TCGCGTCCGGTGTGAAAG-3′ and 5′-CCACATCCAGCATCCAC-3′. The PCRs were conducted using the following program: 2 min for enzyme activation at 50 °C, 10 min for denaturation at 95 °C, 40 cycles of 15 s at 95 °C, 1 min at 60 °C, and a final gradient of 60 °C to 95 °C.
WEAX consumption
After 24 h of fermentation, the culture medium was freeze-dried for analysis of WEAXs consumption. Residual carbohydrate content, ferulic acid content, monosaccharide composition and the molecular weights of WEAXs in the culture medium were recorded and measured as mentioned above. The bioaccessibilities of the AX were calculated from the percentage of the utilized AX content relative to the total AX content.
Statistical analysis
Data were analyzed using repeated measures analysis of variance using SPSS 21.0 statistical software (SPSS Inc., Chicago, IL, USA). The results are expressed as the means ± standard deviation. Statistically significant differences were defined as p < 0.05.
Results and discussion
Features of enzyme-treated WEAXs
Rheological properties. The storage (G′) and loss (G′′) modulus changes in WEAX solutions with/without enzyme treatment were monitored to show the different cross-linked degrees through enzyme treatment21 and the results are shown in Fig. 1. The value of G′′ was approximately 2.0 pascal (Pa) in the WEAX solution alone and was significantly higher than that of G′ (0.2 Pa). The values of G′ and G′′ were similar over time, indicating that the solution was viscous. After adding GOX, the values of G′ and G′′ increased (Fig. 1B and C) because GOX could produce an oxidative coupling through arabinoxylan ferulate esters.13 In GOX 50, the values of G′ and G′′ both increased and the value of G′′ was still significantly higher than that of G′. This result showed that a weak gel was formed in GOX 50 and the viscosity of the solution was higher than its elasticity. When the concentration of GOX was further increased (GOX 400), the value of G′ was increased significantly and was higher than that of G′′ from 630 s. It exhibited a solution to gel transition and strong gelation occurred in GOX 400. It was easy to form gels as the content of GOX increased.22
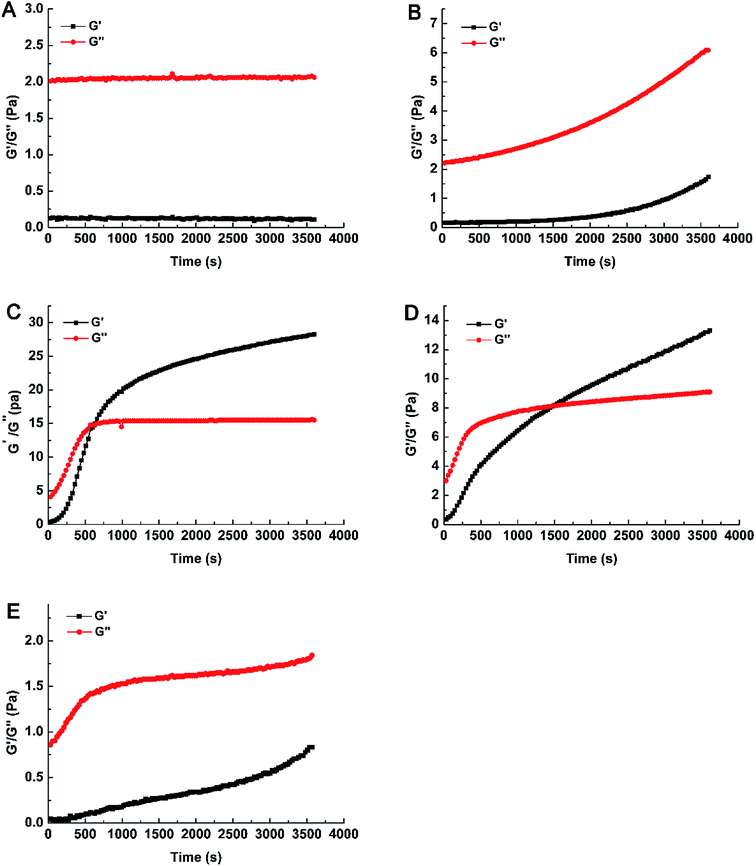 |
| Fig. 1 Monitoring the storage (G′) and loss (G′′) modulus of WEAX solutions with/without enzyme treatment. (A) WEAX; (B) GOX 50; (C) GOX 400; (D) Pn 25 + GOX 400; (E) Pn 200 + GOX 400. WEAX: WEAX without enzyme treatment; GOX 50: WEAX treated with GOX (50 μg g−1 WEAX); GOX 400: WEAX treated with GOX (400 μg g−1 WEAX); Pn 25 + GOX 400: WEAX treated with GOX (400 μg g−1 WEAX) and Pn (25 μg g−1 WEAX); Pn 200 + GOX 400: WEAX treated with GOX (400 μg g−1 WEAX) and Pn (200 μg g−1 WEAX). | |
Compared with GOX 400, the values of G′ and G′′ were decreased with the addition of Pn and GOX (Fig. 1D and E). As the content of Pn increased, the values of G′ and G′′ both decreased. When the content of Pn was 25 μg g−1 (w/w, enzyme/WEAX), the gelation time (1500 s) increased and the gelation rate decreased. The value of G′′ in Pn 25 + GOX 400 was approximately two-fold lower compared to GOX 400. Increasing the content of Pn (200 μg g−1, w/w, enzyme/WEAX) resulted in only weak gelation in the sample. This relationship was associated with a lower density of polysaccharide chains produced by Pn, which decreased the probability of the establishment of a three-dimensional AX network. Hence, WEAXs with different cross-linked degrees were prepared. Weak gels were formed in GOX 50 and Pn 200 + GOX 400, while strong gels were formed in GOX 400 and Pn 25 + GOX 400.
Composition and molecular size distributions of enzyme-treated WEAX samples. The content of ferulic acid was significantly different in the WEAX alone and enzyme modified WEAXs (Table 1). The bound ferulic acid content of the WEAX preparation alone was 2339 μg g−1 AX, consistent with other studies.23 Compared with WEAX alone, the content of bound ferulic acid in enzyme-treated WEAXs significantly decreased (GOX 50, GOX 400, Pn 25 + GOX 400 and Pn 200 + GOX 400 were 70%, 63%, 77% and 86% lower, respectively) (p < 0.05), which was due to the formation of ferulic acid dimers and trimers. The content of ferulic acid in the weak gels was significantly lower than that in the strong gels under the same enzyme action (GOX 50 > GOX 400 and Pn 200 + GOX 400 > Pn 25 + GOX 400) (p < 0.05). All Pn + GOX-modified WEAXs had lower ferulic acid content than GOX-treated WEAXs. These results may be attributable to the shortened chains of WEAXs due to the addition of Pn, which may make it easier to form intermolecular diferulic acid bridges under oxidative conditions.
Table 1 Composition data of WEAX samples with/without enzyme treatmenta
Sample |
AX (%) |
Monosaccharide composition (%) |
A/X |
Ferulic acid (μg g−1) |
Ara |
Xyl |
Glc |
Gal |
Free |
Bound |
Means of triplicates ± standard deviation are presented. Mean values in the same column followed by different superscript letters are significantly different (p < 0.05). WEAX: WEAX without enzyme treatment; GOX 50: WEAX treated with GOX (50 μg g−1 WEAX); GOX 400: WEAX treated with GOX (400 μg g−1 WEAX); Pn 25 + GOX 400: WEAX treated with GOX (400 μg g−1 WEAX) and Pn (25 μg g−1 WEAX); Pn 200 + GOX 400: WEAX treated with GOX (400 μg g−1 WEAX) and Pn (200 μg g−1 WEAX). Ara: arabinose; Xyl: xylose; Glc: glucose; Gal: galactose. |
WEAX |
85.96 ± 2.24 |
31.77 |
64.84 |
1.60 |
1.79 |
0.49 |
71.10 ± 0.69 |
2339 ± 97a |
GOX 50 |
84.71 ± 1.24 |
30.36 |
63.07 |
4.74 |
1.83 |
0.48 |
87.71 ± 15.11 |
709.1 ± 46c |
GOX 400 |
83.69 ± 2.06 |
30.88 |
62.71 |
4.64 |
1.77 |
0.49 |
80.84 ± 6.90 |
871.3 ± 13.7b |
Pn 25 + GOX 400 |
83.27 ± 1.35 |
30.61 |
63.33 |
4.35 |
1.71 |
0.48 |
69.07 ± 5.55 |
547.4 ± 3.2d |
Pn 200 + GOX 400 |
83.80 ± 2.19 |
30.75 |
63.39 |
4.12 |
1.74 |
0.49 |
65.14 ± 5.92 |
313.7 ± 3.5e |
The molecular weight distributions of WEAXs were analyzed by HPSEC-MALLS and are presented in Fig. 2. The addition of GOX at 50 μg g−1 (w/w, enzyme/WEAX) to WEAX showed a slight increase in molecular weight, whereas the mean molecular weight of Pn 200 + GOX 400 was slightly lower, but not significantly different compared to WEAX alone (Fig. 2). There were no significant differences among the AX contents or the A/X ratios of all samples (Table 1).
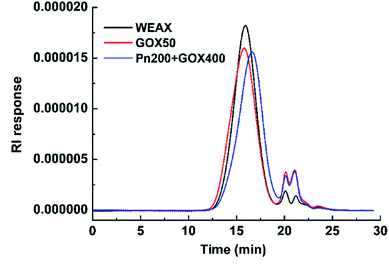 |
| Fig. 2 Molecular weight distributions of the WEAX samples. WEAX: WEAX without enzyme treatment; GOX 50: WEAX treated with GOX (50 μg g−1 WEAX); Pn 200 + GOX 400: WEAX treated with GOX (400 μg g−1 WEAX) and Pn (200 μg g−1 WEAX). The mean molecular weights of WEAX, GOX 50 and Pn 200 + GOX 400 were 1.44 × 105 Da, 2.46 × 105 Da and 1.15 × 105 Da, respectively. | |
The microstructures of enzyme-treated WEAX samples. The surface microstructures of WEAXs with/without enzyme treatment are shown in Fig. 3. Before enzyme treatment, the surface microstructure of WEAX was laminated (Fig. 3A). However, after enzyme treatment, three-dimensional, porous and heterogeneous network structures were formed (Fig. 3B–E) with cross-linked filamentous structures clearly seen on the surface. GOX 400 (Fig. 3C) presented a more compact microstructure in comparison with the geometry and pore sizes of the GOX 50 structure (Fig. 3B). These results are related to the strong cross-linked structure in GOX 400 and are consistent with the changes of the rheological properties (Fig. 1) in GOX 50 and GOX 400. WEAXs treated with Pn + GOX showed thin pore walls with bigger holes, which increased with an increasing Pn content (Fig. 3D and F) compared to WEAX-treated with GOX alone owing to the degradation of the WEAX polymers after the addition of Pn.
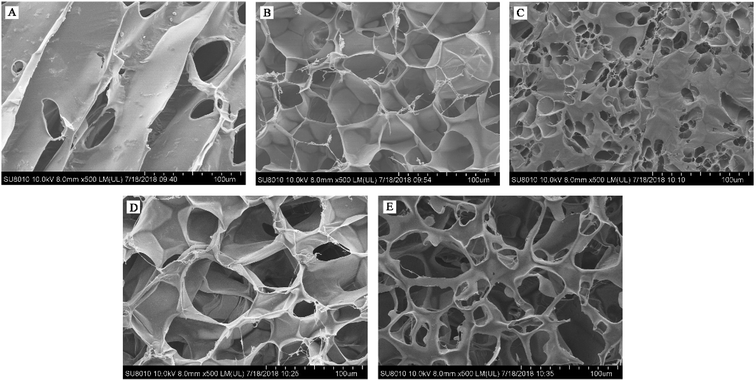 |
| Fig. 3 SEM showing the surface microstructures of the WEAX samples. (A) WEAX; (B) GOX 50; (C) GOX 400; (D) Pn 25 + GOX 400; (E) Pn 200 + GOX 400. WEAX: WEAX without enzyme treatment; GOX 50: WEAX treated with GOX (50 μg g−1 WEAX); GOX 400: WEAX treated with GOX (400 μg g−1 WEAX); Pn 25 + GOX 400: WEAX treated with GOX (400 μg g−1 WEAX) and Pn (25 μg g−1 WEAX); Pn 200 + GOX 400: WEAX treated with GOX (400 μg g−1 WEAX) and Pn (200 μg g−1 WEAX). | |
In vitro fecal fermentation of WEAXs after enzyme treatment
The prebiotic potentials of WEAXs treated with enzymes were evaluated by in vitro fermentation with human fecal microbiota following the methods of Barry et al.24 After 24 h, the bacterial population of bifidobacteria, the accumulation of SCFAs, pH shifts, and the consumption of WEAXs were examined. For comparative purposes, the same type of information was obtained from experiments using inulin and FOS (the positive control, which has a recognized bifidogenic effect),25 and for a medium without a carbon source (negative control) with outcomes measured at 0 and 24 h fermentation.
Effect of enzyme-treated WEAXs on bacterial populations. Bifidobacteria are beneficial bacteria and the prebiotic potential of dietary fiber can be strongly affected by the baseline levels of the two types of bacteria. Fig. 4 shows the bacterial populations of bifidobacteria after 24 h of incubation in anaerobic batch culture following supplementation with inulin, FOS or WEAX (2% w/v). The content of bifidobacteria in the culture medium without carbohydrate (control at 24 h) were decreased by 20%, compared to baseline (0 h), indicating that gut microbiota need carbohydrates to maintain growth. At 24 h, the presence of WEAX resulted in significant increases in the numbers of bifidobacteria (p < 0.05) compared to the controls (Fig. 4A and B). It has been shown previously that bifidobacteria can produce xylosidase, arabinofuranohydrolase and xylanase and thereby utilize arabinose and xylose.26,27
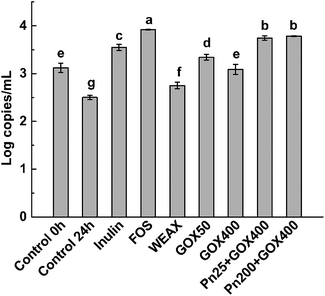 |
| Fig. 4 Bacterial populations of bifidobacteria at 24 h using inulin, FOS and enzyme-treated WEAXs as substrates, compared to the control, using in vitro fermentation for 24 h. Means of triplicates ± standard deviation are presented. Mean values followed by different letters were significantly different (p < 0.05). WEAX: WEAX without enzyme treatment; GOX 50: WEAX treated with GOX (50 μg g−1 WEAX); GOX 400: WEAX treated with GOX (400 μg g−1 WEAX); Pn 25 + GOX 400: WEAX treated with GOX (400 μg g−1 WEAX) and Pn (25 μg g−1 WEAX); Pn 200 + GOX 400: WEAX treated with GOX (400 μg g−1 WEAX) and Pn (200 μg g−1 WEAX). | |
All WEAXs stimulated the growth of bifidobacteria. At 24 h, bifidobacteria levels were higher by 10%, 34%, 24%, 50% and 51% for WEAX alone, GOX 50, GOX 400, Pn 25 + GOX 400 and Pn 200 + GOX 400, respectively, compared to the control at 24 h. All enzyme-treated WEAXs resulted in significant increases in the numbers of bifidobacteria compared to WEAX alone (p < 0.05) (Fig. 4). The increase in the level of bifidobacteria was highest for Pn 25 + GOX 400 and Pn 200 + GOX 400 among all of the WEAXs. Meanwhile, in GOX-treated WEAXs, the amount of bifidobacteria was 10% higher in the culture medium with GOX 50 compared to GOX 400 (Fig. 4A).
SCFAs productions and pH change. The SCFAs produced by fermentation of nondigestible dietary fibers by colonic bacteria can decrease the intestinal pH and inhibit the growth of harmful bacteria.28 Table 2 shows the concentrations of SCFAs and pH shifts after in vitro fermentation of inulin, FOS and WEAXs by colonic bacteria. All of the cultures supplemented with WEAXs contained significantly higher concentrations of acetic acid, propionic acid and butyric acid than the controls at 24 h (p < 0.05), with acetic acid being the dominant SCFA (Table 2).
Table 2 Concentrations of SCFAs (mmol L−1) and pH changes after in vitro fecal fermentation of inulin, FOS and enzyme-treated WEAXs compared to controls using in vitro fermentation for 24 ha
Sample |
Acetic acid |
Propionic acid |
Butyric acid |
pH |
WEAX: WEAX without enzyme treatment; GOX 50: WEAX treated with GOX (50 μg g−1 WEAX); GOX 400: WEAX treated with GOX (400 μg g−1 WEAX); Pn 25 + GOX 400: WEAX treated with GOX (400 μg g−1 WEAX) and Pn (25 μg g−1 WEAX); Pn 200 + GOX 400: WEAX treated with GOX (400 μg g−1 WEAX) and Pn (200 μg g−1 WEAX). Means of triplicates ± standard deviation are presented. Mean values in the same column followed by different superscript letters are significantly different (p < 0.05). |
Control 0 h |
0.40 ± 0.03f |
0.10 ± 0.01f |
1.14 ± 0.00d |
7.88 ± 0.03a |
Control 24 h |
2.03 ± 0.06e |
0.56 ± 0.03e |
1.14 ± 0.01d |
7.45 ± 0.03b |
Inulin |
20.42 ± 0.98b |
1.40 ± 0.02d |
2.30 ± 0.06bc |
4.86 ± 0.02e |
FOS |
14.13 ± 0.28d |
1.52 ± 0.11d |
2.60 ± 0.15ab |
4.63 ± 0.02f |
WEAX |
14.29 ± 1.20d |
2.39 ± 0.02c |
2.22 ± 0.12c |
5.43 ± 0.02c |
GOX 50 |
18.15 ± 0.54c |
2.72 ± 0.03b |
2.38 ± 0.13bc |
5.47 ± 0.05c |
GOX 400 |
18.41 ± 0.84c |
2.42 ± 0.08c |
2.42 ± 0.13bc |
5.42 ± 0.08c |
Pn 25 + GOX 400 |
17.85 ± 0.46c |
2.50 ± 0.13c |
2.43 ± 0.17bc |
5.41 ± 0.04c |
Pn 200 + GOX 400 |
23.50 ± 0.64a |
3.39 ± 0.12a |
2.90 ± 0.17a |
5.20 ± 0.05d |
The results showed that the SCFAs content was significantly different depending on which WEAX was used. The content of acetic acid was significantly higher in the enzyme-treated compared to the nonenzyme treated WEAXs cultures (p < 0.05) (Table 2). The contents of acetic acid and butyric acid in Pn 200 + GOX 400 were approximately 28% and 21% higher, respectively, compared to the other enzyme-treated WEAXs; however, there was no significant difference in the content of acetic acid and butyric acid (p > 0.05) for the other WEAXs. After 24 h, the content of propionic acid in the cultures containing GOX 50 and Pn 200 + GOX 400 was 14% and 42% higher, respectively, than in those supplemented with other WEAXs. The best-known metabolic pathway for acetate and propionate production from gut bacteria involves the metabolism of polysaccharides. Acetate production is mainly by bifidobacteria, and a higher production level of this acid could be related to increased numbers of these bacteria. Propionate can also be produced from fermentable carbohydrates involved with Propionibacterium spp groups and Bacteroides fragilis.29
The SCFAs produced by gut microbiota have important roles. Acetate is often associated with activation of acetyl-CoA carboxylase and changes in the expression profile of regulatory neuropeptides favor appetite suppression.30 Propionate is also associated with the synthesis of cholesterol and might also influence glucose metabolism and postprandial blood glucose levels.31,32 Butyrate, an energy source for epithelial cells, has been reported to play a role in colon cancer prevention.33,34
The increased SCFAs contents were consistent with the decrease in the observed pH values. After 24 h of fermentation, the pH values of all fecal cultures with WEAXs dropped significantly to a range of 5.20–5.47 compared with the control (pH 7.45). These low pH values could promote the bioavailability of calcium and magnesium, inhibit the growth of harmful bacteria and improve the colonic environment.35
The present study showed that higher amounts of bifidobacteria and SCFAs occurred following in vitro fermentation of WEAXs by colonic microbiota, confirming WEAXs have prebiotic potential. Pn 200 + GOX 400 had the highest levels of bifidobacteria and SCFAs than the other WEAXs. Untreated WEAX had the lowest amount of bifidobacteria. Pn 25 + GOX 400 had a stronger prebiotic potential than the GOX treated WEAXs. The levels of bifidobacteria and propionic acid in the culture with GOX 50 were significantly higher compared to GOX 400. The prebiotic potential of the weak gel (GOX 50 and Pn 200 + GOX 400) was significantly higher than the strong gel (GOX 400 and Pn 25 + GOX 400). The different prebiotic potentials of WEAXs with or without enzyme treatment were related to their different microstructures. The three-dimensional, porous and heterogeneous network microstructures of WEAXs after enzyme treatment could retain more culture medium and gut microbiota in the structures of the WEAXs and increase their contact areas with the WEAXs. WEAXs in the weak gels had more contact area than that in the strong gels under the same enzyme treatment. Furthermore, the fine chemical structural details of WEAXs might also impact their prebiotic potentials.
WEAX consumption
The consumption of WEAXs with or without enzyme treatment after fermentation were assessed by measuring their monosaccharide compositions and molecular weights of their residual WEAXs, the bioaccessibilities of the WEAXs, and the ferulic acid contents of the fermentation media (Table 3 and Fig. 5).
Table 3 Changes of molecular weights and A/X ratios of WEAXs after enzyme treatment using in vitro fermentation for 24 ha
Sample |
Mw (Da) |
Monosaccharide composition (%) |
A/X |
Ara |
Xyl |
Glc |
Gal |
WEAX: WEAX without enzyme treatment; GOX 50: WEAX treated with GOX (50 μg g−1 WEAX); GOX 400: WEAX treated with GOX (400 μg g−1 WEAX); Pn 25 + GOX 400: WEAX treated with GOX (400 μg g−1 WEAX) and Pn (25 μg g−1 WEAX); Pn 200 + GOX 400: WEAX treated with GOX (400 μg g−1 WEAX) and Pn (200 μg g−1 WEAX). Ara: arabinose; Xyl: xylose; Glc: glucose; Gal: galactose. Mw: molecular weight. |
WEAX |
2.84 × 104 |
30.82 |
66.78 |
1.23 |
1.17 |
0.46 |
GOX 50 |
2.93 × 104 |
30.11 |
67.28 |
1.39 |
1.22 |
0.45 |
GOX 400 |
2.72 × 104 |
29.22 |
68.05 |
1.69 |
1.04 |
0.43 |
Pn 25 + GOX 400 |
2.24 × 104 |
29.84 |
67.88 |
1.43 |
0.85 |
0.44 |
Pn 200 + GOX 400 |
2.12 × 104 |
29.36 |
68.38 |
1.27 |
0.99 |
0.43 |
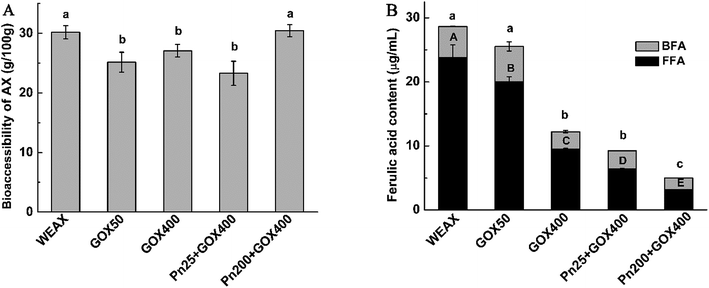 |
| Fig. 5 Bioaccessibilities of WEAXs and the contents of ferulic acid using in vitro fermentation after 24 h. Means of triplicates ± standard deviation are presented. Mean values in the same row followed by different letters were significantly different (p < 0.05). WEAX: WEAX without enzyme treatment; GOX 50: WEAX treated with GOX (50 μg g−1 WEAX); GOX 400: WEAX treated with GOX (400 μg g−1 WEAX); Pn 25 + GOX 400: WEAX treated with GOX (400 μg g−1 WEAX) and Pn (25 μg g−1 WEAX); Pn 200 + GOX 400: WEAX treated with GOX (400 μg g−1 WEAX) and Pn (200 μg g−1 WEAX). FFA: free ferulic acid; BFA: bound ferulic acid. | |
Molecular weights and A/X ratios of WEAXs after in vitro fermentation. The WEAXs were used by the gut microbiota (their mean molecular weights decreased), but their mean molecular weights showed no significant difference in the supernatants of the culture medium for all WEAXs (p > 0.05) (Table 3). These results were associated with xylosidase and α-L-arabinofuranosidase produced by bifidobacteria, and xylanase produced by other microbiota, that could synergistically degrade the AXs.36,37 Xylosidase can release xylose from the nonreducing end of AX, and xylanase can hydrolyze the 1,4-β-D-xylosidic linkage between the xylose residues in a random manner to cleave AX and shorten the main chain.38 α-L-Arabinofuranosidase hydrolyzes the terminal nonreducing α-arabinofuranoses from AX, removing the arabinose from the branched chain.39The A/X ratios were slightly reduced in all fermentation media (Table 3). The decreases in the A/X ratios might be due to the removal of arabinosyl moieties from the polymer by arabinofuranosidase, which then becomes available for fermentation. Despite the degradation of arabinoses and xyloses occurring almost simultaneously, a marked shift was observed in the type of WEAXs. Ferulic acid crosslinking significantly lowered the A/X ratio at 24 h (p < 0.05). This coincided with the ratio of A/X decreasing when supplemented with cross-linked AX, while in the case of noncross-linked polymers, the ratio decreased before 12 h and then increased after 12 h.40 It has been postulated that the network microstructures of WEAXs after enzyme treatment could partially increase the contact areas of WEAXs and gut microbiota. The decreases in the A/X ratios resulted from arabinofuranosidase activity removing arabinosyl moieties from the polymers, which then became available for fermentation. However, it was more difficult for the xylanase to degrade the xylan backbone of the oxidation crosslinking WEAX, because the crosslinking structure restricted the access of xylanolytic enzymes to their target sites. Thus, arabinose side chains could be utilized at a greater rate than xyloses utilization.
Bioaccessibility of WEAXs. All WEAX preparations were partially fermentable, but the extent of the bioaccessibility of WEAXs differed (Fig. 5A). The highest bioaccessibility of WEAXs was observed for WEAX and Pn 200 + GOX 400, whereas GOX 50, GOX 400 and Pn 25 + GOX 400 had the lowest bioaccessibility. Therefore, WEAX and Pn 200 + GOX 400 could be better utilized by gut microbiota. Mark et al. found that AX was utilized more rapidly than the viscous, cross-linked derivative of AX.40 The xylan backbone was more resistant to degradation because the crosslinked structure restricted the access of xylanolytic enzymes to their target sites. However, the bioaccessibility of Pn 200 + GOX 400 had no significant difference from that of WEAX without enzyme treatment (p > 0.05).The results of the microstructure (Fig. 3) showed that Pn 200 + GOX 400 had a thinner pore wall and bigger holes compared with the other enzyme treated WEAXs. In addition to the increased contact area of WEAX and the gut microbiota, degradation of the xylan backbone induced by Pn might be a better possible explanation for these results. It has been suggested that bifidobacteria lack endoxylanase, an enzyme required to degrade AX effectively.28 Before the oxidative crosslinking, the xylan backbone was degraded by Pn. The more nonreductive end xylose of Pn 200 + GOX 400 might be utilized without the need for endoxylanase. Hence, the bioaccessibility and the concentration of bifidobacteria of Pn 200 + GOX 400 were higher than for the other enzyme treated WEAXs.
Interestingly, it was revealed that the contents of bifidobacteria in the culture with the enzyme-treated WEAXs were significantly higher compared to WEAX. However, the bioaccessibilities of Pn 25 + GOX 400, GOX 50 and GOX 400 were lower and the bioaccessibility of Pn 200 + GOX 400 showed no significant difference compared to WEAX without enzyme treatment (Fig. 5A). In addition to the increased contact area resulting from GOX and the nonreductive end xylose caused by Pn, there might also be other reasons for their increased prebiotic potential. The composition data of WEAXs with or without enzyme treatment showed that the content of ferulic acid had changed significantly. It was thus demonstrated that ferulic acid, a main phenolic compound bound to AX, showed the potential to exert prebiotic activity.41
The content of ferulic acid after fermentation. Dietary fiber can act as a carrier to take polyphenols to the colon, where it can be fermented by the action of bacterial enzymes.42 The content of ferulic acid after fermentation was detected and is shown in Fig. 5B. After 24 h of fermentation, the content of bound ferulic acid was significantly reduced, and the content of free ferulic acid was significantly increased (p < 0.05). Ferulic acid could be cleaved from the arabinose side chain by feruloyl esterase and utilized by gut microbiota. The metabolites of ferulic acid, such as hydroxyphenylacetic, phenylpropionic, and phenylbutyric acids, could increase beneficial bacteria such as lactobacilli and inhibit the growth of pathogenic bacteria.43,44 Moreover, it has been reported that the formation of ferulic acid cross-links between AX polymers compared with AX without cross-linking significantly reduces the abundance of enterobacteria, which have been frequently identified as the causative agent of diarrhea in humans.40 In this study, the prebiotic potential of WEAXs increased with the formation of diferulic acid. Thus, the formation of diferulic acid bonds between WEAXs might be viewed as being beneficial and might be an explanation as to why the prebiotic potentials of WEAXs after enzyme treatment were higher than WEAXs without enzyme treatment. This might be a reason for the higher prebiotic potentials of the weak gels compared to the strong gels under the same enzyme treatment. Furthermore, current prebiotics, such as fructo-oligosaccharides and inulin, are limited in their presence in the distal colon and are predominantly fermented in the proximal colon. This might coincide with most chronic colonic diseases originating in the distal colon. In this study, the lower bioaccessibilities of the gels formed by WEAX could result in them being more slowly fermented to allow more WEAXs to remain in the distal colon or to increase fecal bulking, which might have health benefits potential. Further studies are warranted to investigate the content, type and mechanisms of crosslinking ferulic acids on their prebiotic potentials.
Conclusions
The findings of this study confirmed that WEAX treated with GOX had higher bifidobacteria populations than untreated WEAX due to the formation of crosslinking ferulic acid. The combined Pn + GOX treated WEAXs had higher bifidobacteria populations than WEAXs treated with GOX alone due to the combination of ferulic acid crosslinking and the degradation of the xylan backbone. The amounts of bifidobacteria and the SCFAs of the weak gels were significantly higher than the strong gels under the same enzyme treatment (p < 0.05). These results suggest that as the content of crosslinking ferulic acid increased, so did the prebiotic potential of WEAX. In conclusion, the combination of Pn and GOX enzyme treatment can improve the quality of wheat products as well as increase the prebiotic potential of AX. The results of this research provide a theoretical basis for improving the quality and nutritional function of wheat products.
Conflicts of interest
The authors declare no conflicts of interest.
Acknowledgements
The authors are very grateful for the national support from the National Natural Science Foundation of China (No. 31471679, 31571768), National Key R&D Program of China (No. 2018YFD0401005) and the Innovation Project of Chinese Academy of Agricultural Sciences and Basic Scientific Fund (S2016JC06).
References
- M. M. Kaczmarczyk, M. J. Miller and G. G. Freund, Metabolism, 2012, 61, 1058–1066 CrossRef CAS PubMed.
- D. Mudgil and S. Barak, Int. J. Biol. Macromol., 2013, 61, 1–6 CrossRef CAS PubMed.
- S. M. Zhou, X. Z. Liu, G. Yan, W. Qiang, D. Y. Peng and C. Li, Carbohydr. Polym., 2010, 81, 784–789 CrossRef CAS.
- L. Cao, X. Liu, T. Qian, G. Sun, Y. Guo, F. Chang, S. Zhou and X. Sun, Int. J. Biol. Macromol., 2011, 48, 160–164 CrossRef CAS PubMed.
- P. D. Cani, A. Everard and T. Duparc, Curr. Opin. Pharmacol., 2013, 13, 935–940 CrossRef CAS PubMed.
- L. H. Wardwell, C. Huttenhower and W. S. Garrett, Curr. Infect. Dis. Rep., 2011, 13, 28 CrossRef PubMed.
- B. V. Mccleary, T. S. Gibson, H. Allen and T. C. Gams, Starch/Staerke, 1986, 38, 433–437 CrossRef CAS.
- K. Autio, Biotechnol. Adv., 2006, 24, 633–635 CrossRef CAS PubMed.
- C. Primo-Martín and M. A. Martínez-Anaya, J. Food Sci., 2003, 68, 31–41 CrossRef.
- M. Wang, V. Tvan and R. J. Hamer, J. Cereal Sci., 2004, 39, 341–349 CrossRef CAS.
- C. Primo- Martín, M. Wang, W. J. Lichtendonk, J. J. Plijter and R. J. Hamer, J. Sci. Food Agric., 2005, 85, 1186–1196 CrossRef.
- G. Niñomedina, E. Carvajalmillán, A. Rasconchu, J. A. Marquezescalante, V. Guerrero, E. Salasmuñoz, M. Bunzel and J. Ralph, Phytochem. Rev., 2010, 9, 111–120 CrossRef.
- A. Bagdi, S. Tömösközi and L. Nyström, Food Hydrocolloids, 2017, 63, 219–225 CrossRef CAS.
- K. Buksa, A. Nowotna and R. Ziobro, Food Chem., 2016, 192, 991–996 CrossRef CAS PubMed.
- L. Liu, W. Yang, S. W. Cui, Z. Jiang, Q. Chen, H. Qian, L. Wang and S. Zhou, Food Hydrocolloids, 2018, 84, 545–551 CrossRef CAS.
- B. Damen, J. Verspreet, A. Pollet, W. F. Broekaert, J. A. Delcour and C. M. Courtin, Mol. Nutr. Food Res., 2011, 55, 1862–1874 CrossRef CAS.
- S. A. Hughes, P. R. Shewry, L. Li, G. R. Gibson, a. M. L. Sanz and R. A. Rastall, J. Agric. Food Chem., 2007, 55, 4589–4595 CrossRef CAS PubMed.
- W. Yang, Z. Jiang, L. Liu, Y. Lin, L. Wang and S. Zhou, J. Sci. Food Agric., 2017, 97, 1034–1041 CrossRef CAS.
- J. Sun, Y.-F. Chu, X. Wu and R. H. Liu, J. Agric. Food Chem., 2002, 50, 7449–7454 CrossRef CAS PubMed.
- A. L. Hartzell, M. X. Maldonado-Gómez, R. W. Hutkins and D. J. Rose, Bioact. Carbohydr. Diet. Fibre, 2013, 1, 81–88 CrossRef CAS.
- A. L. Martínez-López, E. Carvajal-Millan, V. Micard, A. Rascón-Chu, F. Brown-Bojorquez, N. Sotelo-Cruz, Y. L. López-Franco and J. Lizardi-Mendoza, Carbohydr. Polym., 2016, 144, 76–82 CrossRef PubMed.
- M. S. Izydorczyk, C. G. Biliaderis and W. Bushuk, J. Cereal Sci., 1990, 11, 153–169 CrossRef CAS.
- E. Carvajal-Millan, B. Guigliarelli, V. Belle, X. Rouau and V. Micard, Carbohydr. Polym., 2005, 59, 181–188 CrossRef CAS.
- J. L. Barry, C. Hoebler, G. T. Macfarlane, S. Macfarlane, J. C. Mathers and K. A. Reed, Br. J. Nutr., 1995, 74, 303–322 CrossRef CAS PubMed.
- M. Rossi, C. Corradini, A. Amaretti, M. Nicolini, A. Pompei, S. Zanoni and D. Matteuzzi, Appl. Environ. Microbiol., 2005, 71, 6150–6158 CrossRef CAS PubMed.
- R. Crittenden, S. Karppinen, S. Ojanen, M. Tenkanen, R. Fagerström, J. Mättö, M. Saarela, T. Mattila-Sandholm and K. Poutanen, J. Sci. Food Agric., 2002, 82, 781–789 CrossRef CAS.
- K. M. Van Laere and A. G. Beldman GVoragen, Appl. Microbiol. Biotechnol., 1997, 47, 231–235 CrossRef CAS PubMed.
- C. M. Courtin, Crit. Rev. Food Sci. Nutr., 2011, 51, 178–194 CrossRef PubMed.
- T. L. Millier and M. J. Wolin, Appl. Environ. Microbiol., 1996, 62, 1589–1592 Search PubMed.
- G. Frost, M. L. Sleeth, M. Sahuriarisoylu, B. Lizarbe, S. Cerdan, L. Brody, J. Anastasovska, S. Ghourab, M. Hankir and Z. Shuai, Nat. Commun., 2014, 5, 3611 CrossRef CAS PubMed.
- H. H. Cheng and M. H. Lai, J. Nutr., 2000, 130, 1991 CrossRef CAS PubMed.
- J. M. W. Wong, R. De Souza, C. W. C. Kendall, A. Emam and D. J. A. Jenkins, J. Clin. Gastroenterol., 2006, 40, 235–243 CrossRef CAS.
- S. I. Cook and J. H. Sellin, Aliment. Pharmacol. Ther., 1998, 12, 499–507 CrossRef CAS PubMed.
- W. Scheppach, H. P. Bartram and F. Richter, Eur. J. Cancer, 1995, 31, 1077–1080 CrossRef.
- D. J. Rose, J. A. Patterson and B. R. Hamaker, J. Agric. Food Chem., 2010, 58, 493–499 CrossRef CAS PubMed.
- J. Wang, B. Sun, Y. Cao and C. Wang, Carbohydr. Polym., 2010, 82, 419–423 CrossRef CAS.
- H. Pastell, P. Westermann, A. S. Meyer, P. Tuomainen and M. Tenkanen, J. Agric. Food Chem., 2009, 57, 8598 CrossRef CAS PubMed.
- E. Dornez, K. Gebruers, J. A. Delcour and C. M. Courtin, Trends Food Sci. Technol., 2009, 20, 495–510 CrossRef CAS.
- B. C. Saha, Biotechnol. Adv., 2000, 18, 403–423 CrossRef CAS PubMed.
- H. N. E. Mark, J. Hopkins, S. Macfarlane, E. Furrie, G. T. Macfarlane and A. J. McBain, Appl. Environ. Microbiol., 2003, 69, 6354–6360 CrossRef PubMed.
- F. Biasi, M. Astegiano, M. Maina, G. Leonarduzzi and G. Poli, Curr. Med. Chem., 2011, 18, 4851–4865 CrossRef CAS PubMed.
- F. Sauracalixto, J. Agric. Food Chem., 2011, 59, 43–49 CrossRef CAS PubMed.
- F. Sauracalixto, J. Pérezjiménez, S. Touriño, J. Serrano, E. Fuguet, J. L. Torres and I. Goñi, Mol. Nutr. Food Res., 2010, 54, 939–946 CrossRef CAS PubMed.
- D. Hervert-Hernández, C. Pintado, R. Rotger and I. Goñi, Int. J. Food Microbiol., 2009, 136, 119–122 Search PubMed.
Footnote |
† Equal contributors. |
|
This journal is © The Royal Society of Chemistry 2019 |
Click here to see how this site uses Cookies. View our privacy policy here.