DOI:
10.1039/C9RA02934F
(Paper)
RSC Adv., 2019,
9, 18008-18012
Direct synthesis of covalent triazine-based frameworks (CTFs) through aromatic nucleophilic substitution reactions†
Received
19th April 2019
, Accepted 4th June 2019
First published on 7th June 2019
Abstract
Despite extensive efforts, only three main strategies have been developed to synthesize covalent triazine-based frameworks (CTFs) thus far. We report herein a totally new synthetic strategy which allows C–C bonds in the CTFs to be formed through aromatic nucleophilic substitution reactions. The as-synthesized CTF-1 and CTF-2 exhibited photocatalytic water splitting activity comparable to the CTFs made using ionothermal or Brønsted acid-catalyzed polymerization. Interestingly, CTF-2 distinguished itself by its two-photon fluorescence (emission at ∼530 nm under irradiation at either 400 nm or 800 nm).
Covalent organic networks (CONs), usually porous and physicochemically stable light-weight materials,1 can accommodate, interact with, and discriminate molecules, which provides CONs with many potential applications in catalysis,1i,2 gas separation/storage,1b,1i,2a,3 chemical sensing,4 drug delivery,5 and photovoltaic light harvesting.1b,2a,2b,6 As a subclass of CONs, covalent triazine-based frameworks (CTFs) have attracted researchers' increasing attention worldwide. CTFs represent a class of functionalized polymers based on the trimerization and subsequent oligomerization of aromatic dinitrile monomers in the presence of a catalyst. In brief, there have been three main strategies to synthesize CTFs from aromatic dinitrile monomers: (1) polymerization7 of aromatic dinitrile monomers mediated by ZnCl2 (ionothermal process), or catalyzed by strong Brønsted acid; (2) Friedel–Crafts reaction using 2,4,6-trichloro-1,3,5-triazine (cyanuric chloride);8 and (3) nickel-catalyzed Yamamoto-type Ullmann cross-coupling of 2,4,6-tris-(4-bromo-phenyl)-[1,3,5]triazine.9 While the ZnCl2-mediated ionothermal reaction is still the most widely used method, the high reaction temperature (400 °C) and long reaction time (40 h) limited its practical application. Although variations of the method to reduce the reaction time have been reported, the difficulty of completely removing residual ZnCl2 remained as another obstacle.7b The Brønsted acid method allows the polymerization to occur at ambient temperature, but it can only be applied to certain kinds of aromatic dinitrile monomers. An important environmental issue should not be overlooked in using aromatic dinitrile monomers, especially at large scale, as the dinitrile monomers were often made using over stoichiometric amounts of toxic cyanides, which is of neither economical nor ecological interest. Friedel–Crafts alkylation could work even without any addition of solvent, and is time-efficient, but residues of activating and/or bulking agents need to be removed and recovered upon framework formation. In addition, this approach is only limited to rigid and sterically demanding building blocks. Very recently, Baek's group10 described a synthesis of CTFs at 400 °C through P2O5-mediated condensation of aromatic amide, which can be considered as a variation of ionothermal method of nitriles since P2O5 is known to catalyze the dehydration of amides into nitriles. Impressively, the approach that Jin, Tan and co-workers11 recently reported was by way of condensation of aldehyde and amidine under mild reaction conditions.
Herein, we are reporting a new synthetic strategy in which C–C bonds in the CTFs were formed through aromatic nucleophilic substitution reactions under mild reaction condition (refluxing in toluene). To the best of our knowledge, this is the first time that aromatic nucleophilic substitution was used for C–C bond formation in CTFs, and our method is different in many ways from the previously reported strategies. The as-synthesized CTFs exhibited photocatalytic water splitting activity comparable to the CTFs made using previously reported methods.
While electrophilic substitution reactions are common for aromatic compounds, only some aryl halides can undergo substitution reactions with strong nucleophiles,12 and some of such reactions have been used in covalent organic frameworks (COFs) preparation.13 We therefore envisioned that the chlorines in cyanuric chloride could be displaced by a strong nucleophilic reagent, for example, phenyllithium, and such a reaction could be used to prepare CTFs. To test the feasibility of this idea, we carried out a model reaction of phenyllithium (PhLi) and cyanuric chloride, which, we delightly found, gave 2,4,6-triphenyl-1,3,5-triazine (TriPh-triazine) in an isolated yield of 87% (Scheme 1 and ESI†).
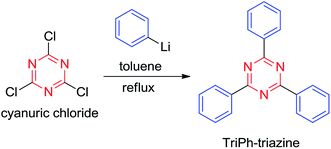 |
| Scheme 1 Reaction of phenyllithium and cyanuric chloride. | |
Under very similar reaction conditions employed in the model reaction, two covalent triazine frameworks, CTF-1 and CTF-2 were successfully synthesized in gram-scale through aromatic nucleophilic substitution reaction of cyanuric chloride with para-dilithiumaromatic reagents in yields of 88% and 96%, respectively. The para-dilithiumaromatic reagents (1,4-dilithiumbenzene and 4,4′-dilithiumbiphenyl) used in the CTFs preparation were obtained through reactions of para-diiodoaromatic reagents with n-butyllithium, a widely used reagent in organic synthesis (caution: a highly reactive reagent and standard operating procedure should be strictly followed) (Scheme 2 and ESI†). Albeit aromatic nucleophilic substitution reactions of cyanuric chloride was previously employed in the preparation COFs through the formation of C–X (X = N, S, O) bonds,14 such reactions have not been used to prepare COFs through C–C bond formation.
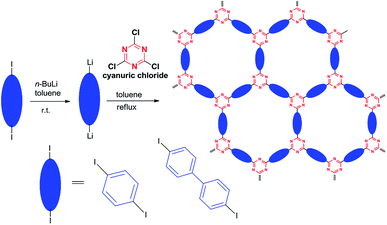 |
| Scheme 2 Synthesis of CTF-1 and CTF-2. | |
CTF-1 and CTF-2 were characterized by Fourier transform infrared (FTIR) spectroscopy, solid-state 13C cross polarization magic angle spinning nuclear magnetic resonance spectroscopy (13C CP/MAS NMR), X-ray photoelectron spectroscopy (XPS) analysis, thermogravimetric (TG) analysis, field emission scanning electron microscopy (FE-SEM), field emission transmission electron microscopy (FE-TEM), powder X-ray diffraction (PXRD) analysis, ultraviolet-visible diffuse reflectance spectroscopy (UV-Vis DRS) and solid-state photoemission spectroscopy.
The solid-state 13C NMR spectra of CTF-1 and CTF-2 (Fig. 1A) showed the presence of sp2-hybridized carbon of the triazine unit (∼170 ppm) as well as peaks of the aromatic rings (150–120 ppm).7d In the FTIR spectrum of CTF-1 (Fig. 1B) (CTF-2, see ESI, Fig. S12†), the disappearance of characteristic C–Cl band of cyanuric chloride at 850 cm−1,8 together with the appearance of vibrational bands of triazine units at 1510 cm−1 (C–N stretching) and 1360 cm−1 (benzene ring breathing) implied the formation of CTFs.15
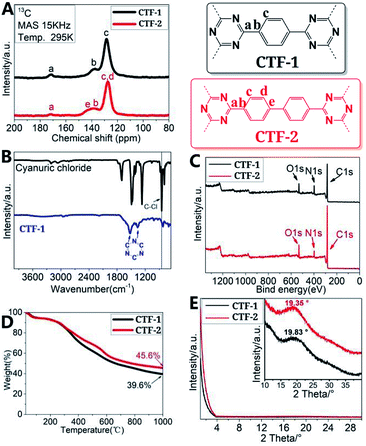 |
| Fig. 1 (A) Solid-state 13C CP/MAS NMR and repeating units of CTFs; (B) FTIR of CTF-1; (C) XPS survey spectra of CTFs; (D) TGA of CTFs; (E) PXRD of CTFs. | |
In the XPS spectra of the CTFs, the appearance of the peaks with binding energies of 286.2 eV and 398.4 eV, assigned to C 1s and N 1s of the triazine (C–N
C) ring, respectively, together with the peak at ∼284.3 eV, assigned to C 1s of the aromatic carbons,7d,16 implied the existence of the triazine and aromatic units. The ratios of Ctriazine
:
Cphenyl in the XPS spectra of CTF-1 and CTF-2 were 1
:
2.55 and 1
:
5.71, respectively, which were close to their theoretical values of 1
:
3 and 1
:
6 (Fig. 1C, S6 and S7 in ESI†). The O 1s peaks seen in Fig. 1C are from physically adsorbed oxygen or water molecules which are usually found in carbon-based materials.9b,17 Scarcely any Cl or I peaks were found in the XPS spectra of CTF-1 and CTF-2 (Fig. 1C and Tables S1 and S2, ESI†), which indicated the completeness of aromatic nucleophilic substitution of cyanuric chloride with the corresponding aryl dilithium reagents. TG analysis shows that both CTF-1 and CTF-2 started decomposition at ∼300 °C and experienced ∼60% weight loss at 1000 °C in nitrogen atmosphere (Fig. 1D). Extended porous network structures with branched morphologies for the as-synthesized CTFs were observed by using FE-SEM (Fig. S19, ESI†) and TEM images (Fig. S19, ESI†). Powder X-ray diffraction (PXRD) patterns of the CTFs showed no distinct sharp peaks, but curved broad peaks at around 2θ = ∼19–20° (Fig. 1E) were observed, declaring their amorphous nature. No long-range crystallographic order for both CTFs indicated that the pore structures of the resulted CTFs maybe formed from unordered stacking and curling of the products. Such PXRD results are expected as the reaction was carried out under refluxing in toluene with vigorous stirring – the conditions unfavourable for the formation of crystalline products.
The porous properties of the both CTFs were evaluated by nitrogen adsorption–desorption measurement at 77 K. Both samples exhibited the type IV adsorption isotherm character,18 typical for materials with mesoporous porosity (Fig. S8, ESI†). The BET surface areas of CTF-1 and CTF-2 are ca. 40–100 m2 g−1, and their pore diameters were calculated to be around 15–24 nm by Barrett–Joyner–Halenda (BJH) method (Table S3, ESI†). The surface areas of the CTFs synthesized using our method are larger than those of the CTFs obtained though Brønsted-acid method (5–8 m2 g−1),7d but smaller than that of the CTF-1 obtained through ionothermal (ZnCl2) process (791 m2 g−1).7b In the ionothermal (ZnCl2) process, zinc chloride might act as template and present in the as-synthesized CTFs products, removing the zinc chloride from the as-synthesized CTFs products could potentially result in higher porosity.19
The UV-Vis diffuse reflectance spectra (UV-Vis DRS) of CTF-1 and CTF-2 (Fig. S10, ESI†) showed the intrinsic absorptions in the range of visible light, which are attributed to π → π* electron transition of Csp2 and Nsp2 in the polymeric networks.7d The Kubelka–Munk transformed reflectance spectra,20 as the mathematical equivalent transformation of UV-Vis DRS, showed band gaps of 2.62 eV and 2.90 eV of CTF-1 and CTF-2 (Fig. 2A), respectively.
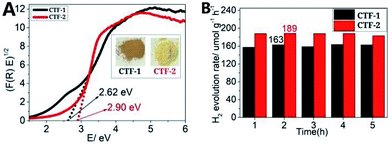 |
| Fig. 2 (A) Kubelka–Munk transformed reflectance spectra of CTFs (inset: optical images of CTFs under visible light); (B) water-splitting reaction catalyzed by the two CTFs. | |
Theoretical calculation (DFT) indicated that the top levels of the valence band (VB) of the CTFs were more positive than the redox potential of O2/H2O (1.23 V vs. NHE), while the bottom levels of their conduction band (CB) were more negative than the redox potential of H+/H2 (0 V vs. NHE) (Fig. S9, ESI†), which implied that the CTFs could be used in photocatalytic water splitting, like those described in previous work.7d,21 The photo-catalytic water splitting abilities (hydrogen evolution reaction, HER) of the as-synthesized CTFs were thus evaluated in comparison with those of previously reported results under visible light (>420 nm) irradiation with triethanolamine (TEOA) as a photogenerated hole scavenger (ESI†). Both CTFs showed water splitting ability under visible light (>420 nm), with CTF-2 possessing a slightly better catalytic activity than CTF-1 (Fig. 2B). Nonetheless, significant improvement was obviously needed on the photocatalytic activity toward water splitting for either CTFs.
In addition to the absorption property and photo-catalytic water splitting abilities (HER) of the as-synthesized CTFs, the emission property of the CTFs was also evaluated. Both CTFs exhibits similar fluorescence spectra under identical higher-energy irradiation (400 nm, violet), as shown in Fig. 3A and B.
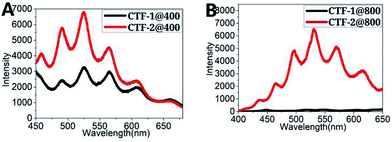 |
| Fig. 3 (A) Solid-state single photo fluorescence spectra (A) (400 nm excitation) and two-photo fluorescence spectra (B) (800 nm excitation) (the intensities of incident light on CTFs at 400 nm and 800 nm are the same, respectively). | |
Interestingly, CTF-2 was found to display distinct two-photo-excited fluorescence (TPF) under lower-energy irradiation using a femtosecond laser pulse (800 nm, infrared) (red curve in Fig. 3B). The observation of two-photon excited fluorescence property, or up-conversion phenomenon, of CTF-2 implied that CTF-2 has a higher two-photo absorption cross section. The difference in the two-photo absorption cross section between CTF-1 and CTF-2 could possibly be attributed to their structural difference. As described in a previous report,22 structural features including effectiveness of conjugation, increased conjugation length, good planarity and strong electron donating ability were all critical to enhance the two-photon absorption cross sections. While the aromatics-linked triazine frameworks in repetitive structures made the both CTFs maintain good planarity, the effectively conjugated aryl units are longer in the biphenyl-linked CTF-2. The molecular force field (MM2)23 calculation (ESI†) indicated that the charge distributions of triazine rings (electron deficient parts) in the two CTFs were almost the same (Ctriazine[+0.318]) (ESI†), but the electron rich portions (aryl rings) of CTF-1 and CTF-2 hold different charge distributions (CTF-1 Caromatic [−0.035] and CTF-2 Caromatic [−0.041]), which could partially explain why CTF-2 has a higher two-photo absorption cross section value. The up-conversion phenomenon of CTF-2 implies that CTF-2 could be excited to the same excited state by either absorbing one photo under higher-energy irradiation (400 nm) or absorbing two photons under lower-energy irradiation (800 nm). As lower-energy irradiation (800 nm) provides a better penetration in scattering or absorbing media,24 especially in organic and biological materials,22 CTF-2 could possibly be used in non-destructive photoimaging and optical memory.25
Conclusions
In conclusion, we have developed a novel synthetic approach to make covalent triazine-based frameworks CTF-1 and CTF-2 by means of aromatic nucleophilic substitution of cyanuric chloride with organic lithium reagents. The as-synthesized CTFs were found to exhibit the expected photocatalytic properties in water-splitting reaction (HER), similar to the CTFs obtained by other means. Additionally, CTF-2's two-photon fluorescence property could allow it to be a potentially useful material in non-destructive photoimaging and optical memory.
Conflicts of interest
The authors declare no conflict of interest.
Acknowledgements
This work was financially supported by the National Key Research Program (2017YFA0206500), the National Natural Science Foundation of China (21871281), the Science and Technology Commission of Shanghai Municipality (16DZ1100300, 18DZ1100403), and the Young Innovation Fund of Shanghai Advanced Research Institute (171008).
Notes and references
-
(a) F. J. Uribe-Romo, J. R. Hunt, H. Furukawa, C. Klock, M. O'Keeffe and O. M. Yaghi, J. Am. Chem. Soc., 2009, 131, 4570 CrossRef CAS PubMed;
(b) Z. H. Xiang and D. P. Cao, J. Mater. Chem. A, 2013, 1, 2691–2718 RSC;
(c) A. G. Slater and A. I. Cooper, Science, 2015, 348, 6238 CrossRef PubMed;
(d) P. Kuhn, A. Forget, D. S. Su, A. Thomas and M. Antonietti, J. Am. Chem. Soc., 2008, 130, 13333–13337 CrossRef CAS PubMed;
(e) J. X. Jiang, F. Su, A. Trewin, C. D. Wood, N. L. Campbell, H. Niu, C. Dickinson, A. Y. Ganin, M. J. Rosseinsky, Y. Z. Khimyak and A. I. Cooper, Angew. Chem., Int. Ed., 2007, 46, 8574–8578 CrossRef CAS PubMed;
(f) C. S. Diercks and O. M. Yaghi, Science, 2017, 355, 6328 CrossRef PubMed;
(g) A. I. Cooper, Adv. Mater., 2009, 21, 1291–1295 CrossRef CAS;
(h) N. Chaoui, M. Trunk, R. Dawson, J. Schmidt and A. Thomas, Chem. Soc. Rev., 2017, 46, 3302–3321 RSC;
(i) R. Dawson, A. I. Cooper and D. J. Adams, Prog. Polym. Sci., 2012, 37, 530–563 CrossRef CAS;
(j) Y. H. Xu, S. B. Jin, H. Xu, A. Nagai and D. L. Jiang, Chem. Soc. Rev., 2013, 42, 8012–8031 RSC;
(k) S. Das, P. Heasman, T. Ben and S. L. Qiu, Chem. Rev., 2017, 117, 1515–1563 CrossRef CAS PubMed.
-
(a) P. J. Waller, F. Gandara and O. M. Yaghi, Acc. Chem. Res., 2015, 48, 3053–3063 CrossRef CAS PubMed;
(b) V. S. Vyas, F. Haase, L. Stegbauer, G. Savasci, F. Podjaski, C. Ochsenfeld and B. V. Lotsch, Nat. Commun., 2015, 6, 8508 CrossRef CAS PubMed;
(c) J. Artz, Chemcatchem, 2018, 10, 1753–1771 CrossRef CAS;
(d) A. V. Bavykina, M. G. Goesten, F. Kapteijn, M. Makkee and J. Gascon, Chemsuschem, 2015, 8, 809–812 CrossRef CAS PubMed;
(e) L. Chen, Y. Yang and D. L. Jiang, J. Am. Chem. Soc., 2010, 132, 9138–9143 CrossRef CAS PubMed;
(f) K. Iwase, T. Yoshioka, S. Nakanishi, K. Hashimoto and K. Kamiya, Angew. Chem., Int. Ed., 2015, 54, 11068–11072 CrossRef CAS PubMed;
(g) P. Katekomol, J. Roeser, M. Bojdys, J. Weber and A. Thomas, Chem. Mater., 2013, 25, 1542–1548 CrossRef CAS;
(h) P. Kaur, J. T. Hupp and S. T. Nguyen, ACS Catal., 2011, 1, 819–835 CrossRef CAS;
(i) L. Z. Peng, P. Liu, Q. Q. Cheng, W. J. Hu, Y. A. Liu, J. S. Li, B. Jiang, X. S. Jia, H. Yang and K. Wen, Chem. Commun., 2018, 54, 4433–4436 RSC;
(j) C. Perego and R. Millini, Chem. Soc. Rev., 2013, 42, 3956–3976 RSC;
(k) X. R. Wang, X. Han, J. Zhang, X. W. Wu, Y. Liu and Y. Cui, J. Am. Chem. Soc., 2016, 138, 12332–12335 CrossRef CAS PubMed;
(l) Y. G. Zhang and S.
N. Riduan, Chem. Soc. Rev., 2012, 41, 2083–2094 RSC;
(m) K. Schwinghammer, S. Hung, M. B. Mesch, J. Senker and B. V. Lotsch, Energy Environ. Sci., 2015, 8, 3345–3353 RSC;
(n) L. Li, W. Fang, P. Zhang, J. Bi, Y. He, J. Wang and W. Su, J. Mater. Chem. A, 2016, 4, 12402–12406 RSC;
(o) J. Bi, W. Fang, L. Li, J. Wang, S. Liang, Y. He, M. Liu and L. Wu, Macromol. Rapid Commun., 2015, 36, 1799–1805 CrossRef CAS PubMed.
-
(a) A. Bhunia, D. Esquivel, S. Dey, R. Fernandez-Teran, Y. Goto, S. Inagaki, P. Van der Voort and C. Janiak, J. Mater. Chem. A, 2016, 4, 13450–13457 RSC;
(b) O. Buyukcakir, S. H. Je, S. N. Talapaneni, D. Kim and A. Coskun, ACS Appl. Mater. Interfaces, 2017, 9, 7209–7216 CrossRef CAS PubMed;
(c) S. S. Han, H. Furukawa, O. M. Yaghi and W. A. Goddard, J. Am. Chem. Soc., 2008, 130, 11580 CrossRef CAS PubMed;
(d) S. S. Han, J. L. Mendoza-Cortes and W. A. Goddard, Chem. Soc. Rev., 2009, 38, 1460–1476 RSC;
(e) N. Huang, X. Chen, R. Krishna and D. L. Jiang, Angew. Chem., Int. Ed., 2015, 54, 2986–2990 CrossRef CAS PubMed;
(f) J. L. Mendoza-Cortes, S. S. Han, H. Furukawa, O. M. Yaghi and W. A. Goddard, J. Phys. Chem. A, 2010, 114, 10824–10833 CrossRef CAS PubMed;
(g) J. L. Mendoza-Cortes, T. A. Pascal and W. A. Goddard, J. Phys. Chem. A, 2011, 115, 13852–13857 CrossRef CAS PubMed;
(h) P. Puthiaraj, S. S. Kim and W. S. Ahn, Chem. Eng. J., 2016, 283, 184–192 CrossRef CAS;
(i) K. K. Wang, H. L. Huang, D. H. Liu, C. Wang, J. P. Li and C. L. Zhong, Environ. Sci. Technol., 2016, 50, 4869–4876 CrossRef CAS PubMed;
(j) W. Zhang, C. Li, Y. P. Yuan, L. G. Qiu, A. J. Xie, Y. H. Shen and J. F. Zhu, J. Mater. Chem., 2010, 20, 6413–6415 RSC;
(k) X. Zhu, C. C. Tian, S. M. Mahurin, S. H. Chai, C. M. Wang, S. Brown, G. M. Veith, H. M. Luo, H. L. Liu and S. Dai, J. Am. Chem. Soc., 2012, 134, 10478–10484 CrossRef CAS PubMed.
-
(a) G. Das, B. P. Biswal, S. Kandambeth, V. Venkatesh, G. Kaur, M. Addicoat, T. Heine, S. Verma and R. Banerjee, Chem. Sci., 2015, 6, 3931–3939 RSC;
(b) S. Y. Ding, M. Dong, Y. W. Wang, Y. T. Chen, H. Z. Wang, C. Y. Su and W. Wang, J. Am. Chem. Soc., 2016, 138, 3031–3037 CrossRef CAS PubMed;
(c) Z. P. Li, Y. W. Zhang, H. Xia, Y. Mu and X. M. Liu, Chem. Commun., 2016, 52, 6613–6616 RSC;
(d) L. Xiang, Y. L. Zhu, S. Gu, D. Y. Chen, X. Fu, Y. D. Zhang, G. P. Yu, C. Y. Pan and Y. H. Hu, Macromol. Rapid Commun., 2015, 36, 1566–1571 CrossRef CAS PubMed.
-
(a) A. Rengaraj, P. Puthiaraj, Y. Haldorai, N. S. Heo, S. K. Hwang, Y. K. Han, S. Kwon, W. S. Ahn and Y. S. Huh, ACS Appl. Mater. Interfaces, 2016, 8, 8947–8955 CrossRef CAS PubMed;
(b) H. R. Wang, W. W. Zhu, L. Z. Feng, Q. Chen, Y. Chao, Z. L. Dong and Z. Liu, Nano Res., 2018, 11, 3244–3257 CrossRef CAS.
-
(a) C. R. Mulzer, L. X. Shen, R. P. Bisbey, J. R. McKone, N. Zhang, H. D. Abruna and W. R. Dichtel, ACS Cent. Sci., 2016, 2, 667–673 CrossRef CAS PubMed;
(b) F. X. Yang, S. S. Cheng, X. T. Zhang, X. C. Ren, R. J. Li, H. L. Dong and W. P. Hu, Adv. Mater., 2018, 30, 1702415 CrossRef;
(c) X. Feng, X. S. Ding and D. L. Jiang, Chem. Soc. Rev., 2012, 41, 6010–6022 RSC.
-
(a) M. J. Bojdys, J. Jeromenok, A. Thomas and M. Antonietti, Adv. Mater., 2010, 22, 2202 CrossRef CAS PubMed;
(b) P. Kuhn, M. Antonietti and A. Thomas, Angew. Chem., Int. Ed., 2008, 47, 3450–3453 CrossRef CAS PubMed;
(c) P. Kuhn, A. Thomas and M. Antonietti, Macromolecules, 2009, 42, 319–326 CrossRef CAS;
(d) Z. A. Lan, Y. X. Fang, Y. F. Zhang and X. C. Wang, Angew. Chem., Int. Ed., 2018, 57, 470–474 CrossRef CAS PubMed.
-
(a) S. Dey, A. Bhunia, D. Esquivel and C. Janiak, J. Mater. Chem. A, 2016, 4, 6259–6263 RSC;
(b) P. Puthiaraj, S. M. Cho, Y. R. Lee and W. S. Ahn, J. Mater. Chem. A, 2015, 3, 6792–6797 RSC.
-
(a) Z. H. Xiang and D. P. Cao, Macromol. Rapid Commun., 2012, 33, 1184–1190 CrossRef CAS PubMed;
(b) Z. H. Xiang, D. P. Cao, L. Huang, J. L. Shui, M. Wang and L. M. Dai, Adv. Mater., 2014, 26, 3315 CrossRef CAS PubMed;
(c) Z. H. Xiang, X. Zhou, C. H. Zhou, S. Zhong, X. He, C. P. Qin and D. P. Cao, J. Mater. Chem., 2012, 22, 22663–22669 RSC.
- S. Y. Yu, J. Mahmood, H. J. Noh, J. M. Seo, S. M. Jung, S. H. Shin, Y. K. Im, I. Y. Jeon and J. B. Baek, Angew. Chem., Int. Ed., 2018, 57, 8438–8442 CrossRef CAS PubMed.
-
(a) M. Y. Liu, Q. Huang, S. L. Wang, Z. Y. Li, B. Y. Li, S. B. Jin and B. Tan, Angew. Chem., Int. Ed., 2018, 57, 11968–11972 CrossRef CAS;
(b) K. W. Wang, L. M. Yang, X. Wang, L. P. Guo, G. Cheng, C. Zhang, S. B. Jin, B. Tan and A. Cooper, Angew. Chem., Int. Ed., 2017, 56, 14149–14153 CrossRef CAS.
-
(a) D. P. Green and D. Zuev, in Encyclopedia of Reagents for Organic Synthesis, John Wiley & Sons, Ltd, 2008, DOI:10.1002/047084289X.rp076.pub2;
(b) L. Xu, P.-F. Wang, J.-J. Zhang, W. Wu, J.-W. Shi, J.-F. Yuan, H. Han and H. Wang, RSC Adv., 2015, 5, 51745–51749 RSC.
- B. Zhang, M. F. Wei, H. Y. Mao, X. K. Pei, S. A. Alshmimri, J. A. Reimer and O. M. Yaghi, J. Am. Chem. Soc., 2018, 140, 12715–12719 CrossRef CAS.
- P. Puthiaraj, Y. R. Lee, S. Q. Zhang and W. S. Ahn, J. Mater. Chem. A, 2016, 4, 16288–16311 RSC.
- Y. H. Xiong, Y. M. Qin, L. J. Su and F. G. Ye, Chem. - Eur. J., 2017, 23, 11037–11045 CrossRef CAS PubMed.
- Z. H. Sheng, L. Shao, J. J. Chen, W. J. Bao, F. B. Wang and X. H. Xia, ACS Nano, 2011, 5, 4350–4358 CrossRef CAS PubMed.
-
(a) D. C. Wei, Y. Q. Liu, Y. Wang, H. L. Zhang, L. P. Huang and G. Yu, Nano Lett., 2009, 9, 1752–1758 CrossRef CAS PubMed;
(b) L. T. Qu, Y. Liu, J. B. Baek and L. M. Dai, ACS Nano, 2010, 4, 1321–1326 CrossRef CAS PubMed.
- K. A. Cychosz, R. Guillet-Nicolas, J. Garcia-Martinez and M. Thommes, Chem. Soc. Rev., 2017, 46, 389–414 RSC.
- S. J. Ren, M. J. Bojdys, R. Dawson, A. Laybourn, Y. Z. Khimyak, D. J. Adams and A. I. Cooper, Adv. Mater., 2012, 24, 2357–2361 CrossRef CAS.
- M. Otsuka, Powder Technol., 2004, 141, 244–250 CrossRef CAS.
-
(a) A. Fujishima and K. Honda, Nature, 1972, 238, 37 CrossRef CAS;
(b) A. Kudo and Y. Miseki, Chem. Soc. Rev., 2009, 38, 253–278 RSC;
(c) G. Zhang, Z.-A. Lan and X. Wang, Angew. Chem., Int. Ed., 2016, 55, 15712–15727 CrossRef.
- B. A. Reinhardt, L. L. Brott, S. J. Clarson, A. G. Dillard, J. C. Bhatt, R. Kannan, L. X. Yuan, G. S. He and P. N. Prasad, Chem. Mater., 1998, 10, 1863–1874 CrossRef CAS.
- N. L. Allinger, J. Am. Chem. Soc., 1977, 99, 8127–8134 CrossRef CAS.
- M. Albota, D. Beljonne, J. L. Bredas, J. E. Ehrlich, J. Y. Fu, A. A. Heikal, S. E. Hess, T. Kogej, M. D. Levin, S. R. Marder, D. McCord-Maughon, J. W. Perry, H. Rockel, M. Rumi, C. Subramaniam, W. W. Webb, X. L. Wu and C. Xu, Science, 1998, 281, 1653–1656 CrossRef CAS.
-
(a) H. M. Kim and B. R. Cho, Chem. Rev., 2015, 115, 5014–5055 CrossRef CAS;
(b) Q. Liu, B. D. Guo, Z. Y. Rao, B. H. Zhang and J. R. Gong, Nano Lett., 2013, 13, 2436–2441 CrossRef CAS PubMed.
Footnote |
† Electronic supplementary information (ESI) available. See DOI: 10.1039/c9ra02934f |
|
This journal is © The Royal Society of Chemistry 2019 |
Click here to see how this site uses Cookies. View our privacy policy here.