DOI:
10.1039/C9RA02978H
(Paper)
RSC Adv., 2019,
9, 25569-25575
The interaction mechanism between alkaloids and pepsin based on lum-AuNPs in the chemiluminescence analysis
Received
21st April 2019
, Accepted 20th July 2019
First published on 15th August 2019
Abstract
Herein, novel luminol functional gold nanoparticles (lum-AuNPs) were quickly prepared in an alkaline luminol solution with HAuCl4, which had the unique characteristics of uniform size and excellent luminescence properties. A self-made flow injection-chemiluminescence (FI-CL) system was established to study the interaction between pepsin (Pep) and five alkaloids (anisodamine, berberine, reserpine, jatrorrhizine and matrine) using lum-AuNPs as the CL probe. Based on the abovementioned home-made CL system, the possible interaction mechanisms of Pep with five alkaloids have been comprehensively discussed by molecular docking simulation, chemical thermodynamics and kinetic studies. The results indicated that there were obvious CL enhancement and inhibition effects on the lum-AuNPs CL system for the Pep and the complex of Pep/alkaloids, respectively. The possible mechanism for the interaction of Pep–five alkaloids was mainly mediated by the hydrophobic force. The binding constant K and binding site n for the Pep–alkaloid interaction are consistent with the list of Ber > Res > Ani, Jat > Mat, which is relative to the potential of groups of alkaloids interacting with the active site of Pep.
1. Introduction
Protein–drug interaction mechanism studies have attracted significant attention for drug development and clinical safety evaluation.1–5 Martel and others6–9 reviewed the up-to-date research progress on the interaction of proteins with drugs from the point of method principle, information extraction, material consumption and high throughput property. With the gradual improvement of analytical techniques such as circular dichroism spectroscopy,10,11 mass spectrometry,12–17 and time-resolved Fourier transform infrared (TR-FTIR) spectroscopy,18 a large number of studies have been reported on the interaction between proteins and drugs. On this basis, some researchers have developed some new methods based on chemiluminescence (CL),19–21 fluorescence22,23 and ultraviolet spectrum24,25 to study the interaction between proteins and small-molecule drugs with higher sensitivity.
Pepsin (Pep) (MW: 34.5 kDa) is mainly a monomeric, two-domain, l-protein, with a high percentage of acidic residues (43 out of 327). It contains 6 cysteine residues, 5 tryptophan residues, 16 tyrosine residues, 14 phenylalanine residues and so on. The residues Asp32 and Asp215 are the active sites of Pep located on the sides of cavities. Pep, as a digestive protease, is most efficient at cleaving peptide bonds between hydrophobic and aromatic amino acids, and the isoelectric point (PI) of Pep is 5.3 26–28. Recently, Pep has been broadly used as a model protein to investigate the interaction between proteins and ligands. The interaction behaviour of Pep with bisphenol A and nobiletin was systemically investigated using fluorescence spectroscopy, UV visible absorption spectroscopy, resonance light scattering, synchronous fluorescence spectroscopy, 3D spectroscopy and molecular docking (MD), and the relative interaction parameters, such as binding constants and thermo-dynamic parameters, were also provided.
A variety of nanomaterials,29–32 especially functional Au nanoparticles (AuNPs), have attracted significant research interest in the fields of chemistry,33–35 materials science,36–38, biology39 and medicine;40,41 significant efforts have been devoted towards the exploration of synthesis methods for AuNPs, and AuNPs with stability, functionality and special photoelectric chemical properties have been achieved via the formation of bonds between the S and N atoms on the surface of the Au atom under different reaction conditions. The reported methods for the AuNP synthesis have always been complicated and time consuming, and AuNPs with a non-uniform particle size under the interplay of kinetics and thermodynamics are usually obtained; therefore, some new materials as promising alternatives to AuNPs and detection methods have been explored.42–44 Recently, significant efforts have been made for the quick preparation of AuNPs by controlling the quantum confinement phenomena (QCP) with the photochemical effects. This method also provides sufficient chemical groups for the preparation of functional AuNPs.45,46
The aim of this study was to focus on the interaction between five alkaloids and pepsin in the HAuCl4–luminol CL system. The first aspect of novelty is that luminol functional AuNPs can be quickly produced under an alkaline condition by mixing the solution of luminol with HAuCl4; lum-AuNPs of 40 nm size were produced by a photo-induced chemical reaction of luminol. It has higher CL intensity when compared with the traditional luminol CL system. Based on this characteristic of lum-AuNPs, home-made lum-AuNPs as the CL probe were constructed to study the Pep–5 alkaloid interaction by the enhancement and quenching effect of the lum-AuNP-based CL system; the possible mechanism of the Pep–5 alkaloid interaction was elucidated by the home-made lum-AuNP-based CL system and molecular docking from the viewpoint of kinetics and thermodynamics. This is the first study in which lum-AuNPs have been used for investigating the interaction between pepsin and five alkaloids to explore the possible mechanism based on the enhancement and quenching effect of the CL system.
2. Results and discussion
2.1 Characterization of lum-AuNPs
To further understand the luminescence properties of lum-AuNPs, the UV spectra were obtained by UV-Vis absorption spectroscopy in the range of 190–700 nm (Fig. 1); the results indicated that the lum-AuNPs have three characteristic absorption peaks at 225 nm, 300 nm and 367 nm, which were different from those of luminol and HAuCl4. They had an obvious red-shift when compared with the 223 nm, 298 nm and 350 nm peaks of luminol under the same alkaline conditions. The possible reason for this result may be the cumulative effect of the Au atoms in the lum-AuNPs. The stability of the lum-AuNPs formed in the luminol–HAuCl4 alkaline mixture solution was also investigated; the result indicated that lum-AuNPs had better stability in the alkaline solution due to the formation of bonds between the N and the Au atoms on the surface of the Au atom when compared with some other AuNPs prepared using citric acid as a ligand absorbed on the surface of AuNPs via the formation of the C–Au bond.
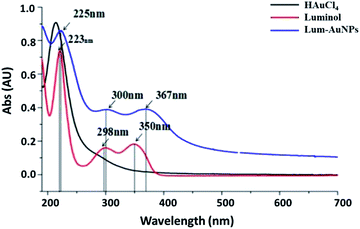 |
| Fig. 1 UV-Vis spectra of lum-AuNPs, luminol and HAuCl4 in the range of 190–700 nm. | |
Moreover, the TEM image shows that the lum-AuNPs have the same morphology and particle size, that is, 40 nm or so, and the surface is smooth and has obvious texture appearance (Fig. 2); we speculate that the possible reason for this may be the strong hydrophobic nature of the alkaline solution.
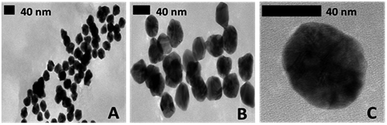 |
| Fig. 2 TEM image of the lum-AuNPs prepared in the luminol–HAuCl4 alkaline solution at the pH value of 10.6. | |
The XRD spectrum (Fig. 3) showed that the lum-AuNPs prepared using the luminol–HAuCl4 home-made CL system had virtually no adulterating element, and only the Au, Na, C, O and Cl elements were present; from the view of particle size and surface ligand, lum-AuNPs has the similar size and shape, which may be caused by the quick CL reaction performed by the QCP effect under the condition of alkaline.
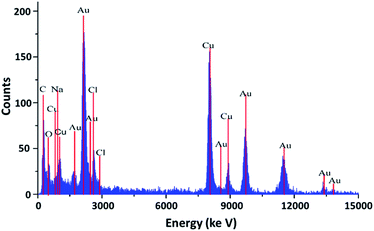 |
| Fig. 3 XRD pattern of the lum-AuNPs in the system of luminol–HAuCl4. | |
2.2 Study on the CL system of lum-AuNPs-Pep
The static injection CL method was used to evaluate the CL kinetics process in the CL system of lum-AuNPs-Pep. As shown in Fig. 4, the Imax value of the CL intensity for the lum-AuNPs-O2 CL system has obviously increased from 165 to 278, and the Tmax value has reduced from 21.8 s to 21.4 s in the presence of Pep in the system. Interestingly, the relative CL intensity of the lum-AuNP-O2 system increases with the increasing Pep concentration, and the CL intensity increment is proportional to the Pep concentrations ranging from 0.01 to 1.00 μmol L−1, with the linear equation of ΔICL = 106.27CPep + 15.05 (R2 = 0.9938); this indicates that the electron transfer rate in the lum-AuNPs can be accelerated by the change in Pep conformation due to the redox reaction of luminol with the excited 3-aminophthalate.
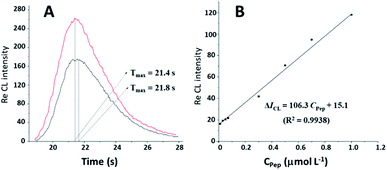 |
| Fig. 4 The system of lum-AuNPs (black) and lum-AuNP-Pep (red) CL graph (A). The linear relationship graph (B) of lum-AuNPs CL with different concentrations of Pep. The concentration of lum-AuNPs was 0.1 μmol L−1, and the concentration range of Pep was 0.01–1.0 μmol L−1. | |
2.3 Study on the photochemical behaviour of five alkaloids in the lum-AuNP CL system
Based on the interaction between Pep and small-molecule drugs, the interactions between Pep and 5 alkaloids were also investigated in the lum-AuNP home-made CL system. The detailed graphs of 5 alkaloids at different concentrations in the Pep-based lum-AuNP CL system are shown in Fig. 5. Although these 5 alkaloids existed in the entire CL system, the CL intensity showed different decreasing trends at different concentrations of these alkaloids. However, for the lum-AuNPs-O2 system, no obvious differences in the presence and absence of alkaloids were observed. Based on this phenomenon, we can elucidate that there is an obvious interaction between the lum-AuNPs and the alkaloids; this means that Pep acts as a protein. Thus, the CL intensity quenching effect of different alkaloids on the lum-AuNPs-Pep system might be caused by the interaction of Pep with alkaloids. The possible CL mechanism of the lum-AuNPs-Pep–alkaloid system can be explained as follows: under alkaline conditions, the Au3+ ions and negatively charged amino acid residues on the protein can form the Pep–Au3+ complex on the surface of Pep. Due to the unstable conformation of Pep in an alkaline solution, the negatively charged polar residues with abnormally high pKa values can promote the Au3+ ion flow to the vicinity of negatively charged amino acid residues based on the principle of charge density matching and form sub-nanometer AuNPs; then, under the alkaline conditions, luminol forms the excited 3-aminophthalate by the active free radicals in the solution, which promotes the Au3+ ions on the surface of the protein to further accumulate in the core of Au to form AuNPs containing luminol. Moreover, microscopic changes in the Pep conformation could accelerate the electron transfer rate of the excited 3-aminophthalate, leading to the enhancement of CL (CEC); on the other hand, alkaloids present in the CL system would exert an inhibitory effect, i.e. the complex quenching effect of CL (CQC) based on the cascading progress of Pep–alkaloid interaction, on the CL intensity.
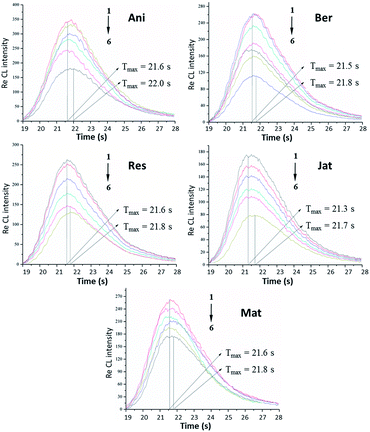 |
| Fig. 5 The CL of 5 alkaloids at different concentrations in the lum-AuNPs-Pep CL system. The linear ranges of Ani, Ber, Res, Jat and Mat were 0.05–70, 0.01–30, 0.07–50, 0.05–50 and 0.03–30 nmol L−1, respectively. The concentration of lum-AuNPs and Pep was 0.1 and 1.0 μmol L−1. | |
Under the above mentioned optimal experimental conditions, a series of standard solutions of 5 alkaloids were determined by the lum-AuNPs-Pep CL system. In the presence of 5 alkaloids, i.e. anisodamine, berberine, reserpine, jatrorrhizine and matrine, the CL intensity decrement is proportional to the logarithm of alkaloid concentration in the range of 0.05–70 nmol L−1, 0.01–30 nmol L−1, 0.07–50 nmol L−1, 0.05–50 nmol L−1 and 0.03–30 nmol L−1, respectively, by following the general equation of ΔICL = A
ln
C + B (herein, the slope A represents the determination sensitivity of alkaloids by lum-AuNPs-Pep CL system). The results are presented in Table 1, which shows the determination sensitivity of alkaloids in the lum-AuNP system ranked in the order of Mat > Jat > Res > Ani > Ber.
Table 1 Linear relationship, LOD and correlation coefficient of 5 alkaloids in the lum-AuNPs-Pep CL system
Drugs |
The linear relationship |
Concentration range (nmol L−1) |
LOD (pmol L−1) |
r |
Ber |
ΔICL = 11.21 ln C + 60.96 |
0.01–30 |
3.20 |
0.9824 |
Ani |
ΔICL = 12.09 ln C + 62.45 |
0.05–70 |
16.0 |
0.9801 |
Res |
ΔICL = 23.56 ln C + 78.29 |
0.07–50 |
23.0 |
0.9806 |
Jat |
ΔICL = 36.12 ln C + 54.89 |
0.05–50 |
16.0 |
0.9895 |
Mat |
ΔICL = 43.26 ln C + 49.23 |
0.03–30 |
10.0 |
0.9837 |
2.4 Study on the interaction between Pep and 5 alkaloids
To gain greater insights into the mechanisms of interaction between Pep and 5 alkaloids, the van't Hoff equation was adopted for obtaining the thermodynamic and kinetic parameters using the FI-CL model of lg[(I0 − I)/I] = lg
K + n
lg[D] (I0 and I refer to the CL intensity of the lum-AuNPs-Pep system with and without alkaloids, respectively, and [D] refers to the alkaloid concentration). The binding parameters of Pep with 5 alkaloids are listed in Table 2 with the plots of lg[(I0 − I)/I] vs. lg[D]. The results showed that the apparent binding constants K of Pep with alkaloids at the different temperature. The number of binding sites n reveals that there are different levels of binding sites for different alkaloids. The binding abilities of alkaloids to Pep followed the order Ber > Res > Ani, Jat > Mat.
Table 2 Kinetic and thermodynamic parameters of five alkaloids in the lum-AuNPs-Pep CL system
Drug |
Temperature (K) |
Binding constants (L mol−1) |
Number of binding sites |
ΔH (kJ mol−1) |
ΔS (J mol−1 K−1) |
ΔG (kJ mol−1) |
Ani |
283 |
1.9 × 106 |
1.23 |
268.85 |
10.32 |
−28.07 |
298 |
7.1 × 106 |
1.74 |
−43.54 |
313 |
2.9 × 106 |
1.56 |
−56.21 |
Ber |
283 |
1.9 × 105 |
1.51 |
302.75 |
12.91 |
−33.26 |
298 |
1.1 × 106 |
1.89 |
−36.64 |
313 |
4.9 × 105 |
1.72 |
−42.06 |
Res |
283 |
8.9 × 105 |
1.21 |
488.85 |
17.14 |
−38.17 |
298 |
2.4 × 106 |
1.83 |
−54.98 |
313 |
1.9 × 106 |
1.59 |
−69.34 |
Jat |
283 |
2.6 × 105 |
1.21 |
219.32 |
18.24 |
−26.54 |
298 |
6.4 × 106 |
1.75 |
−42.36 |
313 |
5.3 × 106 |
1.62 |
−60.15 |
Mat |
283 |
1.6 × 106 |
0.64 |
413.26 |
28.57 |
−37.12 |
298 |
5.7 × 106 |
0.92 |
−57.69 |
313 |
2.9 × 106 |
0.78 |
−69.32 |
Enthalpy change (ΔH), entropy change (ΔS) and binding free energy change (ΔG) of Pep with alkaloids were obtained using the van't Hoff equation ln
K = −ΔH/RT + ΔS/R. The thermodynamic parameters of alkaloid binding to Pep are listed in Table 2 with the plots of ln
K vs. 1/T. It is shown that there are strong binding affinities of Pep towards different alkaloids. The signs for ΔS and ΔH are positive, and the sign for ΔG is negative, suggesting that the binding process is spontaneous and exothermic, and the hydrophobic interaction force plays a major role in the binding of alkaloids to the Pep active sites.
2.5 Molecular docking
AutoDock and PyMOL were employed to simulate the process of molecular docking of Pep and 5 alkaloids. The molecular docking results are presented in Table 3 and Fig. 6. It has been found that all alkaloids bind to the Pep cavity located among two sides of Pep with the residues Asp32 and Asp215. For the binding of hydrophilic alkaloids to Pep, it has been found that the binding cavity is formed by the hydrophilic residues Asp32, Gly217, Gly76, Glu288, and Leu220, which are mainly distributed on the two sides of the cavity of Pep; this suggests that hydrophilic interaction is the dominant stable force for the binding of hydrophilic alkaloids to Pep. From the results of molecular docking, it is clear that Mat, Ani and Res share the same amino acid residue Asp32; however, they have some other different amino acid residues such as Gly217 and Glu288; similarly, Ber and Jat share the same residue Glu288; however, they have other different residues such as Gly76, Asp32 and Leu220.
Table 3 The molecular docking results of Pep and 5 alkaloids
Drug |
Active site |
Binding distance Å |
Binding constants (L mol−1) |
Surface area (Å2) |
ΔG° (298.13 K) (kJ mol−1) |
Ani |
Asp32 |
2.1 |
8.91 × 106 |
218.45 |
−39.52 |
Gly217 |
2.5 |
Ber |
Gly76 |
2.2 |
5.17 × 105 |
175.42 |
−31.59 |
Glu288 |
3.5 |
Res |
Asp32 |
2.5 |
1.17 × 106 |
119.38 |
−60.23 |
Glu288 |
2.9 |
Jat |
Glu288 |
2.0 |
3.90 × 106 |
219.52 |
−39.15 |
Leu220 |
2.1 |
Mat |
Asp32 |
2.2 |
1.60 × 106 |
342.56 |
−60.12 |
2.0 |
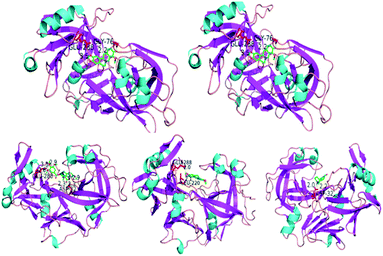 |
| Fig. 6 The docking complex of Pep and 5 alkaloids, Ani (Asp32 and Gly217), Ber (Gly76 and Glu288), Res (Asp32 and Glu288), Jat (Glu288 and Leu220) and Mat (Asp32). | |
The bond distance and occupancy surface area obtained from the results of molecular docking show that alkaloids have a different conformation-induced trend due to the binding of different active functional groups to the corresponding amino acid residues in the cavity of Pep. The binding constant K and binding free energy ΔG for the Pep–alkaloid interaction are consistent with the results obtained by the proposed FI-CL analysis, following the same order of Ber > Res > Ani, Jat > Mat.
2.6 Discussions on the interaction mechanism between Pep and 5 alkaloids
Using lum-AuNPs as the CL probe, Pep and the complex of Pep/alkaloids exhibit an enhancement effect and inhibition effect on the lum-AuNPs CL system. Based on abovementioned results, combined with the molecular docking result, the possible interaction mechanism of Pep with 5 alkaloids in the lum-AuNP CL system has been discussed as follows:
(1) Asp32 and Asp215 are the two active sites of Pep; this is consistent with the results reported in the literature28 and these active sites have an enhancement effect on the luminol CL intensity. The lum-AuNP CL probes, which are formed by the process of molecular absorption between luminol and the AuNPs via the formation of the N–Au bonds when luminol and the AuNPs are mixed together, can further increase the electron transfer rate of luminol and excited 3-aminophthalate to enhance the CL intensity of the system. The results for this process could enhance the CL intensity due to the quantum confinement effect of intermediate product of luminol.
(2) Considering the interaction between Pep and 5 alkaloids, the results obtained are as follows: Ani interacts with Asp32 and Gly217; Ber interacts with Gly76 and Glu288; Res interacts with Asp32 and Glu288; Mat interacts with Glu288 and Leu220; and Jat interacts with Asp32. Asp32 mainly exhibits biological activity in the bioconformation of Pep; however, other amino acid residues have a strong hydrophilic effect on the active site of protein and can induce a synergistic effect on the conformation of Pep via the interaction of hydrogen bond forces and hydrophobicity. Combined with the special characteristic of amino acid residues, it showed that the conformation change of Pep is the result of synergistic effect of those alkaloids interacted with the corresponding amino acid residues and give rise to the CL intensity changed via the lum-AuNPs mediated.
Thus, the entire possible mechanism can be simplified as the following steps: (1) in the lum-AuNP pepsin CL system, “tiny” changes in the conformation of Pep can be enlarged by the CL intensity via the formation of lum-AuNPs in the CL system; (2) there is an obvious enhancement or quenching effect of CL in the presence or absence of 5 alkaloids in the lum-AuNPs-Pep CL system.
3. Experimental
3.1 Chemicals and reagents
All the reagents used in this study were of analytical grade. Water was purified using the Milli-Q system (Millipore, Bedford, MA, USA) with the resistivity of 18.2 MΩ cm−1 and used throughout the experiment. Luminol (Fluka, Biochemika, Switzerland) and Pep (porcine gastric mucosa, Sigma-Aldrich, St. Louis, MO, USA) were used without further purification. Moreover, five alkaloids (anisodamine, berberine, reserpine, jatrorrhizine, and matrine) were purchased from the National Institute of Control of Pharmaceutical and Biological Products, China. Chloroauric acid (HAuCl4, analytical grade) was purchased from Shanghai Reagent Factory, China.
A stock solution of five alkaloids (anisodamine, berberine, reserpine, jatrorrhizine, and matrine) (1.0 mmol L−1) and Pep (100.0 μmol L−1) was prepared in purified water and stored at 4 °C. A working standard solution of five alkaloids and Pep was prepared daily by diluting the stock solution appropriately with purified water. A stock solution of luminol (2.5 × 10−2 mol L−1) was prepared by dissolving 0.44 g luminol in a 100 mL NaOH (1.0 × 10−1 mol L−1) solution in a brown calibrated flask. A stock solution of HAuCl4 (2.5 × 10−2 mol L−1) was prepared by dissolving 1.0 g HAuCl4 in 100 mL purified water and stored at 4 °C.
3.2 Materials and instruments
The apparatus (Model IFFM-E, Xi'an Remax Electronic Science-Tech. Co. Ltd) of the FI-CL system consisted of a sampling system, a photomultiplier tube (PMT), and a PC with the IFFM-E client system (Remax, Xi'an, China). A polytetrafluoroethylene (PTFE) tube (1.0 mm i.d.) was used to carry the solution. The UV-Vis absorption spectra (225–800 nm) were obtained using the U-3010 spectrophotometer system (Hitachi, Japan). The TEM images were obtained using the Tecnai G2 F20 S-TWI JEM-2010 transmission electron microscope (FEI, USA) operated at 200 kV.
3.3 Preparation of the lum-AuNP solution
Based on previous studies, the prepared lum-AuNPs were modified as follows: 1 mL of 0.1 mmol L−1 HAuCl4 aqueous solution was added to 30 mL ultrapure water. Then, the mixed 400 μL of 2.5 mmol L−1 luminol–NaOH alkaline solution with pH 9.6 was added to the HAuCl4 solution under vigorous stirring. The lum-AuNP dispersion solution was obtained after 1 h of constant stirring when the solution turned from bright yellow to grey black. According to a previous study,46 the lum-AuNP dispersion was successfully prepared (Fig. 7).
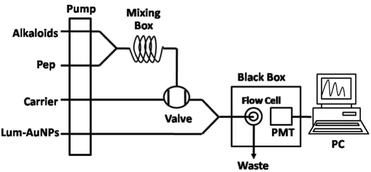 |
| Fig. 7 Schematic of the flow injection chemiluminescence system. | |
3.4 Establishment of the lum-AuNPs-Pep CL system
The system of lum-AuNPs-Pep CL is composed of four pipelines, containing lum-AuNPs, carrying liquid (H2O), Pep solution and alkaline solution. The peristaltic pump was set at the flow rate of 0.2 mL min−1 to promote the movement of solution in these pipelines. When the signal becomes stable, the lum-AuNPs–NaOH solution with pH 10.5 was quantificationally injected using a six-way valve and mixed it with pepsin solution and alkaline solution. The mixture was transported to a disc-shaped sample tube by pipeline, and CL signals were generated. The CL signals were detected by PMT (HV = 650 mV) and then obtained and processed using a computer. The concentration of the sample solution was quantitated by the difference in the relative CL intensity between the sample and the background, that is ΔICL = I0 − IS. I0 and IS are the CL signals of the background and the sample, respectively. According to the precious study,29 the adaptability of the CL system to the foreign species was tested with the standard solution of pepsin (0.1 ng mL−1). For the potential interfering substances in the CL system, the tolerable concentrations of foreign species with respect to 0.1 ng mL−1 pepsin for interference at the 5.0% level were less than 0.5 μg mL−1.
3.5 Molecular docking of five alkaloids with pepsin
The MD of Pep–alkaloids was performed using the open-free software AutoDock 4.2 in the semi-flexible docking mode. The crystal structure of Pep (PDB entry 1YX9) was obtained from the Protein Data Bank. The 3D structures of five alkaloids were generated using the ChemDraw 10.0 and Chem3D 10.0 softwares (Cambridge Soft, USA); moreover, the energy-minimized conformation was obtained by the Gasteiger–Huckel charges with the gradient of 0.005 kcal mol−1.30 With the aid of AutoDock tools, the ligand root of five alkaloids was detected, and rotatable bonds were free-defined. The grid box with 60 Å × 60 Å × 60 Å along the x, y, z axes of 0.375 Å spacing was set in the entire process of MD. The population minimum and maximum numbers of energy evaluation were set as 1.5 × 102 and 2.5 × 106, respectively. The Lamarckian genetic algorithm was applied for the docking simulations. The conformation with the lowest binding energy was analyzed using PyMOL 1.6.0.0.
4. Conclusions
Based on the lum-AuNPs used as the CL probe, the interaction of 5 alkaloids with Pep was systemically studied via a home-made CL analysis platform and the molecular docking technology; the interaction parameters, including the number of binding sites and the thermodynamics parameter, were detected by the molecular docking simulation study and thermodynamic and kinetic analysis of the home-made CL system. The characteristic of the interaction indicated that the interaction between Pep and 5 alkaloids was mainly in the form of hydrophobic force. The binding constant K and binding site n for the Pep–alkaloid interaction are consistent with the order Ber > Res > Ani, Jat > Mat, which is relative to the potential of the groups of alkaloids interacting with the active site of Pep. This study has laid the groundwork for the future studies about the interaction of other proteins and small-molecule drugs based on the CL probe carried by nanoparticles.
Conflicts of interest
There are no conflicts to declare.
Acknowledgements
This work was supported by the grants received from the Primary R&D Plan of Shaanxi Province (2017KW-055, 2018SF-293), the Scientific Research Plan Projects of Shaanxi Provincial Education Department (17JK0764), the Innovation and Entrepreneurship Training Program of Northwest University (2017159, 2018289), the Changjiang Scholars and Innovative Research Team in University (IRT_15R55), the National Natural Science Foundation of China (81603259), and the Opening Foundation of Key Laboratory of Resource Biology and Biotechnology in Western China (Northwest University), Ministry of Education.
Notes and references
- M. J. S. Phipps, T. Fox, C. S. Tautermann and C. K. Skylaris, Chem. Soc. Rev., 2015, 44, 3177–3214 RSC.
- F. B. M. Reinhard, D. Eberhard, T. Werner, H. Franken, D. Childs, C. Doce, M. F. Savitski, W. Huber, M. Bantscheff, M. M. Savitski and G. Drewes, Nat. Methods, 2015, 12, 1129–1131 CrossRef CAS PubMed.
- M. Arai, J. C. Ferreon and P. E. Wright, J. Am. Chem. Soc., 2012, 134, 3792–3803 CrossRef CAS PubMed.
- X. H. Li, P. L. Chavali and M. M. Babu, Science, 2018, 359, 1105–1106 CrossRef CAS PubMed.
- P. N. Lowe, C. K. Vaughan and T. Daviter, Measurement of Protein-Ligand Complex Formation, Springer, Berlin, 2013 Search PubMed.
- J. Antony, S. Grimme, D. G. Liakos and F. Neese, J. Phys. Chem. A, 2011, 115, 11210–11220 CrossRef CAS.
- N. D. Yilmazer and M. Korth, J. Phys. Chem. B, 2013, 117, 8075–8084 CrossRef CAS PubMed.
- L. A. Burns, L. Vázquez-Mayagoitia, B. G. Sumpter and C. D. Sherrill, J. Chem. Phys., 2011, 134, 084107–084131 CrossRef PubMed.
- T. Daviter, N. Chmel and A. Rodger, Circular and Linear Dichroism Spectroscopy for the Study of Protein–Ligand Interactions, Springer, Berlin, 2013, pp. 211–241 Search PubMed.
- H. Nevidalova, L. Michalcova and Z. Glatz, Electrophoresis, 2018, 39, 581–589 CrossRef CAS PubMed.
- N. Jonker, J. Kool, H. Irth and W. M. A. Niessen, Anal. Bioanal. Chem., 2011, 399, 2669–2681 CrossRef CAS.
- C. E. Eyers, M. Vonderach, S. Ferries, K. Jeacock and P. A. Eyers, Curr. Opin. Chem. Biol., 2018, 42, 167–176 CrossRef CAS PubMed.
- A. C. Leney and A. J. R. Heck, J. Am. Soc. Mass Spectrom., 2017, 28, 5–13 CrossRef CAS.
- H. B. Guo, H. Peng and A. Emili, Expert Opin. Drug Discovery, 2017, 12, 1271–1280 CrossRef CAS.
- F. Busch, Z. L. VanAernum, Y. Ju, J. Yan, J. D. Gilbert, R. S. Quintyn, M. Bern and V. H. Wysocki, Anal. Chem., 2018, 90, 12796–12801 CrossRef CAS PubMed.
- R. Gahoual, A. K. Heidenreich, G. W. Somsen, P. Bulau, D. Reusch, M. Wuhrer and M. Haberger, Anal. Chem., 2017, 89, 5404–5412 CrossRef CAS.
- L. F. Cheow, R. Viswanathan, C. S. Chin, N. Jennifer, R. C. Jones, E. Guccione, S. R. Quake and W. F. Burkholder, Anal. Chem., 2014, 86, 9901–9908 CrossRef CAS PubMed.
- A. M. Goodman, O. Neumann, K. Norregaard, L. Henderson, M. R. Choi, S. E. Clare and N. J. Halas, Proc. Natl. Acad. Sci. U. S. A., 2017, 114, 12419–12424 CrossRef CAS PubMed.
- B. M. Dunn, Chem. Rev., 2002, 102, 4431–4458 CrossRef CAS PubMed.
- K. Luo, X. H. Zheng and Z. H. Song, Appl. Biochem. Biotechnol., 2015, 175, 1805–1816 CrossRef CAS PubMed.
- Y. He, B. Xu, W. H. Li and H. L. Yu, J. Agric. Food Chem., 2015, 63, 2930–2934 CrossRef CAS PubMed.
- V. D. Suryawanshi, L. S. Walekar, A. H. Gore, P. V. Anbhule and G. B. Kolekar, J. Pharm. Anal., 2016, 6, 56–63 CrossRef PubMed.
- J. Jiang, Y. Chai and H. Cui, RSC Adv., 2011, 1, 247–254 RSC.
- J. Wang, S. Zhang, J. Zhang, P. Ren, Y. Chen, J. Li, W. Wang, Y. Ma, R. Shi, C. Wang and Z. Yuan, J. Chromatogr. B: Anal. Technol. Biomed. Life Sci., 2011, 879, 1605–1609 CrossRef CAS PubMed.
- R. Gahoual, A. K. Heidenreich, G. W. Somsen, P. Bulau, D. Reusch, M. Wuhre and M. Haberger, Anal. Chem., 2017, 89, 5404–5412 CrossRef CAS PubMed.
- B. M. Dunn, Chem. Rev., 2002, 102, 4431–4458 CrossRef CAS PubMed.
- V. K. Antonov, L. M. Ginodman, V. Kapitannikov, T. N. Barshevskaya, A. G. GurovaL and L. D. Rumsh, FEBS Lett., 1978, 88, 87–90 CrossRef CAS PubMed.
- D. R. Dee and R. Y. Yada, Biochemistry, 2010, 49, 365–371 CrossRef CAS PubMed.
- W. Huang, J. H. Wang, J. Y. Du, Y. Q. Deng and Y. He, Microchim. Acta, 2019, 186, 79 CrossRef PubMed.
- M. X. Zhao, Y. Tao, W. Huang and Y. He, Phys. Chem. Chem. Phys., 2018, 20, 28644–28648 RSC.
- M. X. Zhao, H. L. Yu and Y. He, Sens. Actuators, B, 2019, 283, 329–333 CrossRef CAS.
- Y. Zhou, W. Huang and Y. He, Sens. Actuators, B, 2018, 270, 187–191 CrossRef CAS.
- T. Wang, D. C. Wang, J. W. Padelford, J. Jiang and G. L. Wang, J. Am. Chem. Soc., 2016, 138, 6380–6383 CrossRef CAS PubMed.
- M. G. Méndez-Medrano, E. Kowalska, A. Lehoux, A. Herissan, B. Ohtani, S. Rau, C. Colbeau-Justin, J. L. Rodríguez-López and H. Remita, J. Phys. Chem. C, 2016, 120, 25010–25022 CrossRef.
- T. Shu, L. Su, J. Wang, X. Lu, F. Liang, C. Z. Li and X. J. Zhang, Anal. Chem., 2016, 88, 6071–6077 CrossRef CAS PubMed.
- C. Liu, T. Li, G. Li, K. Nobusada, C. Zeng, G. S. Pang, N. L. Rosi and R. C. Jin, Angew. Chem., Int. Ed., 2015, 54, 9826–9829 CrossRef CAS.
- Y. B. Song, F. Y. Fu, J. Zhang, J. S. Chai, X. Kang, P. Li, S. L. Li, H. P. Zhou and M. Z. Zhou, Angew. Chem., Int. Ed., 2015, 54, 8430–8434 CrossRef CAS PubMed.
- R. Tian, D. P. Yan, C. Y. Li, S. M. Xu, R. Z. Liang, L. Y. Guo, M. Wei, D. G. Evans and X. Duan, Nanoscale, 2016, 8, 9815–9821 RSC.
- X. Y. Zhang, Cell Biochem. Biophys., 2015, 72, 771–775 CrossRef CAS PubMed.
- S. H. Au, J. Edd, D. A. Haber, S. Maheswaran, S. L. Stott and M. Toner, Curr. Opin. Biomed. Eng., 2017, 3, 13–19 CrossRef PubMed.
- X. J. Li and K. J. Kono, Polym. Int., 2018, 67, 840–852 CrossRef CAS.
- M. J. Li, X. X. Huang and H. L. Yu, Mater. Sci. Eng., C, 2019, 101, 614–618 CrossRef CAS PubMed.
- Y. L. Yue and Y. He, Anal. Sci., 2019, 35, 159–163 CrossRef CAS PubMed.
- J. Y. Du, M. X. Zhao, W. Huang, Y. Q. Deng and Y. He, Anal. Bioanal. Chem., 2018, 410, 4519–4526 CrossRef CAS PubMed.
- D. Kato, H. Sakai, T. Saegusa, N. V. Tkachenko and T. Hasobe, J. Phys. Chem. C, 2017, 121, 9043–9052 CrossRef CAS.
- K. Luo, X. Zheng and Z. Song, RSC Adv., 2014, 4, 45230–45233 RSC.
Footnote |
† These authors contributed equally to this work. |
|
This journal is © The Royal Society of Chemistry 2019 |
Click here to see how this site uses Cookies. View our privacy policy here.