DOI:
10.1039/C9RA04494A
(Paper)
RSC Adv., 2019,
9, 26315-26320
Highly selective and sensitive detection of amaranth by using carbon dots-based nanosensor†
Received
15th June 2019
, Accepted 11th August 2019
First published on 22nd August 2019
Abstract
In this work, a novel fluorescence nanosensor for selective and sensitive determination of amaranth was constructed using carbon dots (C-dots). Water soluble C-dots with strong fluorescence were obtained by a simple microwave-assisted method using urea and glycine as raw materials. It was found that amaranth can efficiently and sensitively quench the C-dots fluorescence by the inner filter effect (IFE) and non-radiative energy transfer (NRET) mechanisms. The fluorescence quenching efficiency (F0/F) was strongly correlated with the concentration of amaranth in the 0.2–30 μM range. The detection limit (LOD) is 0.021 μM. There was no significant change in the fluorescence intensity of C-dots when other potentially interfering substances were present in the system. Our C-dots-based nanosensor was successfully utilized for the analysis of amaranth in drinks and showed rapid, sensitive and accurate responses. It indicates that the novel C-dots-based nanosensor has great potential in amaranth detection for real-life applications.
Introduction
Coloration is important for consumer perception and acceptance, because it often correlates with the freshness or acceptability of the food. Food dyes can be either natural or synthetic dyes. Natural dyes are usually extracted from natural sources and show some disadvantages in their properties such as low tinctorial strength, instability toward light, pH, temperature and redox agents, high cost and easy discoloration during processing or storage. Compared with natural dyes, synthetic ones offer colour uniformity, good stability, low cost and strong dyeing power, therefore, they are widely used in the food industry. However, many synthetic dyes pose potential risks to human health and their use is strictly regulated by laws and regulations all over the world.
Amaranth (Fig. S1†), a typical synthetic aromatic azo dye showing fascinating red color, is often used as an additive in foods and soft drinks. However, some studies showed negative effects such as cytotoxicity, cytostatic and genotoxicity of amaranth on human health.1,2 Based on Food and Agriculture Organization (FAO) and World Health Organization (WHO) guidance, the acceptable daily amaranth intake should be within 0–0.5 mg kg−1.3 Thus, the amount of amaranth in food must be strictly controlled and it is of high significance to develop an accurate, sensitive, simple, rapid and economic method for amaranth detection to safeguard human health and food safety. Currently, methods for amaranth determination include high performance liquid chromatography-diode array detector (HPLC-DAD),4,5 high performance liquid chromatography-ultra-violet detection (HPLC-UV),6 liquid chromatography-mass spectrometry (LC-MS),7 electrochemistry,8–10 capillary electrophoresis (CE),11 thin layer chromatography (TLC)12 and enzyme-linked immunosorbent assay (ELISA)13 methods. Although these methods have been effectively used for amaranth detection, they still have some shortcomings such as materials and time consumption, complex operation and expensive instruments.14 Compared with other analytical methods, fluorescence method is a preferable method for trace analysis because of its low cost, simple operation, rapid and high sensitivity.15
Carbon dots (C-dots) represent a new member family of carbon nanomaterials with former family members of fullerenes, nano-diamonds, graphene sheets and carbon nanotubes.16,17 It was first discovered in 2004 during separating and purifying sing-walled carbon nanotubes.18 The C-dots are typically almost spherical with sizes less than 10 nm. The C-dots cores generally consist of amorphous carbon or sp2 hybridized carbons.19 Since C-dots discovery, various methods have been established to synthesize them, including laser ablation,20 chemical and electrochemical oxidation,21,22 carbonizing organic molecules,23 hydrothermal strategies24 and microwave25,26 methods. C-dots, as emerging light-emitting nanomaterials, have drawn great attention because of their prominent characteristics such as facile synthesis, easy functionalization, favorable photoluminescence, good water solubility, high chemical and photo stability, excellent biocompatibility and low cytotoxicity.27–29 These outstanding properties make C-dots a promising material and can be used in the fields of bioimaging,30–32 energy conversion and storage,33 biomedicine34 and photocatalysis.35 In addition, C-dots are also widely used to detect various analytes based on changes in fluorescence intensity. Sooksin's group prepared N-doped C-dots and used them as fluorescence nanosensor for Al3+ detection.36 Zhang's group synthesized C-dots doped with N and applied them as nanosensor for Cr(VI) and ascorbic acid detection.37 Zan's group synthesized C-dots simultaneously doped with P and N and used them as an effective fluorescent nanosensor for nitrite ions detection.38 Dang's group produced C-dots doped with both N and S and used them for the detection of Ag+.39 Zeng's group produced C-dots with poly-ethylenimine functionalized for selective detection of 6-thioguanine.40 However, as far as we aware, research on the application of C-dots as a fluorescent nanosensor for selective and sensitive detection of amaranth was not published in the literature.
In this work, we first reported the application of C-dots as a fluorescent nanosensor for the detection of amaranth. The C-dots were synthesized using urea and glycine as raw materials. As-synthesized C-dots exhibit excellent solubility in water and show strong fluorescence, which can be efficiently quenched by amaranth. Using these properties of C-dots, we developed a selective and sensitive nanosensor based on fluorescence quenching to detect amaranth. This C-dots-based nanosensor was successfully implemented for amaranth analysis in drinks. The proposed fluorescence method not only exhibited excellent sensitivity and selectivity but also demonstrated its feasibility for practical applications.
Experimental
Materials
Amaranth (95%) was bought from Aladdin Chemistry Limited Company (Shanghai, China). Urea was from Tianjin Jinbei Fine Chemical Limited Company (Tianjin, China). Quinine sulfate was obtained from Sinopharm Chemical Reagent Limited Company (Shanghai, China). Dibasic sodium phosphates (Na2HPO4), sodium hydroxide (NaOH), hydrochloric acid (HCl), sodium dihydrogen phosphate (NaH2PO4) and potassium bromide (KBr) were acquired from Tianjin Zhiyuan Chemical Reagent Limited Company (Tianjin, China). Starch, KCl, CaCl2, ZnCl2, FeCl3 and NaCl were bought from Jiangsu Qiangsheng Functional Chemistry Limited Company (Changshu, China). Glycine, phenylalanine, vitamin C, vitamin B1, tyrosine, threonine, alanine and glutamic acid were purchased from Tianjin Damo Chemical Reagent Factory (Tianjin, China). Glucose and malt sugar were purchased from Tianjin Beichen Fangzheng Chemical Reagent Factory (Tianjin, China). All reagents were analytically pure and used without further purification. Deionized water (resistivity of 18.2 MΩ cm−1) was used in all experiments. Phosphate buffer saline (PBS) solutions with different pH values of 2–12 were obtained by adding different amounts of NaOH or HCl (1 M) to 0.01 M mixture solution of Na2HPO4 and NaH2PO4. 2 mM amaranth standard solution was prepared by using deionized water as solvent and keep in a refrigerator at 4 °C for further use.
Synthesis of C-dots
The C-dots were produced by microwave irradiation of a solution containing urea and glycine. To prepare this solution, 2.0 g of urea was mixed with 2.0 g of glycine in 40 mL of deionized water. The mixed solution was irradiated in a microwave reactor for 3 min with microwave radiation at 800 W. The resulting brown products were then mixed with 60 mL of deionized water and dialyzed using dialysis tubing (MWCO, 500–1000 Da) against deionized water for 12 h, after which the C-dots solution was freeze dried.
Characterization
Transmission electron microscopy (TEM) was performed by a FEI Tecnai F-20 TEM (Hillsboro, USA) instrument at an accelerating voltage of 200 kV. TEM samples were prepared by dropping the C-dots aqueous solution onto carbon-coated copper grid which was left to dry before analysis. Fourier transform infrared (FTIR) spectra were recorded using Bruker Tensor 27 FTIR spectrometer (Bruker, Germany) at a resolution of 4 cm−1 in the range of 400–400 cm−1. The C-dots/KBr disk was prepared by mixing few milligrams of C-dots with about 100 mg of KBr powder, grinding to approximately 2 μm in a mortar and subjecting to 29.7 MPa pressure by a pressing machine. X-ray photoelectron spectroscopy (XPS) was performed by using Krato XPS instrument (Krato, UK) with AIKα radiation operating at 1486.6 eV. UV-vis absorption spectra were recorded on TU-1800 spectrometer (Beijing, China) in 1 cm quartz cell and the spectra were collected from 200 to 700 nm. Fluorescence spectra were obtained using F-2500 spectrometer (Hitachi, Japan) equipped with a xenon lamp as the excitation light source. Time-resolved fluorescence decay spectra were performed on an Edinburgh FLS 920 spectrometer (Edinburgh, UK) with the excitation wavelength at 320 nm.
Sensing of amaranth
For analysis of amaranth, 1800 μL PBS solution (0.01 M, pH = 7) was mixed with 200 μL of C-dots solution (1 mg mL−1) and then 30 μL of amaranth or sample solution at different concentration (0–2 mM) was added (final concentration of the amaranth is 0–30 μM). The mixed solution was hand-shaken and incubated for 5 min at ambient environment. 10 nm was set as the emission and excitation slit width. Fluorescence intensity was obtained at 320 nm excitation wavelength. Quenching efficiency was calculated based on the relative fluorescence intensity (F0/F), where F0 and F are C-dots fluorescence intensity without and with amaranth presence in the solution, respectively. Other substances such as sugars (glucose, starch and malt sugar), vitamins (vitamin C and vitamin B1), amino acids (glycine, phenylalanine, tyrosine, threonine, alanine and glutamic acid) and salts (KCl, CaCl2, ZnCl2, FeCl3 and NaCl) were measured under the same conditions for selectivity test.
Results and discussion
Characterization of C-dots
To study the optical characteristics of the C-dots, the UV-vis absorption and fluorescence spectra were characterized. UV-vis absorption spectra of C-dots (Fig. 1A) showed a peak appear at 320 nm because of n → π* transition of C
N and C
O,41–43 which suggesting nitrogen and oxygen-containing functional groups on the surface of the C-dots. C-dots solution is light yellow color (inset of Fig. 1A). It changed to bright blue fluorescence after exposure to UV light. The maximum emission and excitation wavelengths were observed at 380 and 320 nm, respectively. C-dots fluorescence emission spectra depended on excitation wavelengths (Fig. 1B). When excitation wavelength changed from 300 nm to 430 nm, the C-dots emission spectra red-shifted from 375 nm to 475 nm because of presence of various functional groups on C-dots surface and various sizes of C-dots.44 C-dots quantum yield (ΦS) was 13%, which was determined using quinine sulfate as a reference (Fig. S2†).
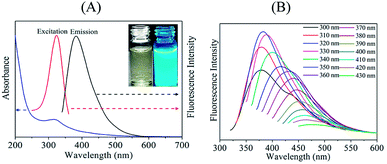 |
| Fig. 1 (A) UV-vis absorption (blue line), excitation (red line) and emission (black line) spectra of the C-dots. Inset: photograph image of the C-dots solution under daylight (left) and UV light (right). (B) Fluorescence emission spectra of the C-dots at different excitation wavelengths (300–430 nm). | |
TEM was applied to characterize the particle size and morphology of the C-dots (Fig. 2). TEM image shows the C-dots have good dispersion and are nearly spherical. The C-dots particle sizes were distributed in a wide range of 1.3–5.6 nm. The average particle size was 3.3 nm, which was calculated by averaging particle sizes of 100 random particles.
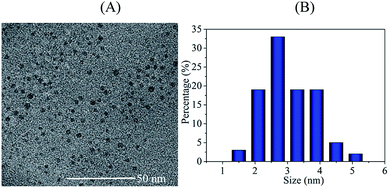 |
| Fig. 2 (A) TEM image and (B) particle size distribution of the C-dots. | |
C-dots surface functional groups were determined using FTIR (Fig. S3†). A broad absorption band between 3000 and 3500 cm−1 is attributed to O–H and N–H stretching vibrations.45 The absorption bands at 2845 and 2927 cm−1 are ascribed to stretching vibration of C–H. Absorption bands at 1459, 1605 and 1721 cm−1 correspond to the CH2, C
N and C
O stretching vibrations, respectively. Peaks associated with stretching vibrations of C–N and CO–NH appear at 1155 and 1395 cm−1, respectively.46,47 Band at 1080 cm−1 is assigned to the C–O bond.48 Furthermore, the XPS analysis was also performed to further investigate the detailed compositional information of the C-dots (Fig. 3). Full XPS spectrum (Fig. 3A) shows three main peaks at 285.8, 531.5 and 399.7 eV, which corresponded to C 1s, O 1s and N 1s, respectively, confirming the C-dots mainly contained C, O and N. In detail, the high-resolution C 1s spectrum (Fig. 3B) contained peaks at 288.3, 285.9 and 284.5 eV, which correspond to C
O, C–N and C
C bonds, respectively.49 High-resolution N 1s spectrum (Fig. 3C) demonstrated peaks corresponding to N–H at 402.2 eV and C–N group at 399.9 eV.50 High-resolution O 1s spectrum (Fig. 3D) confirmed presence of C–O and C
O groups with corresponding peaks at 531.7 and 531.3 eV. Both the FTIR and XPS results agreed on existence of amide, amine, carboxyl and hydroxyl groups on the surface of the C-dots, which ensure excellent water solubility and strong fluorescence of C-dots.
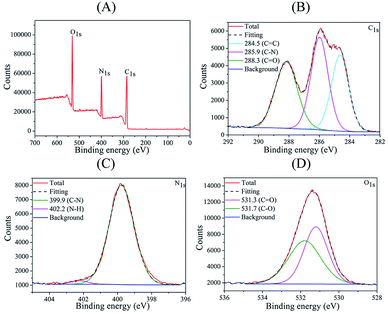 |
| Fig. 3 (A) Full scan XPS spectrum, (B) C 1s spectrum, (C) N 1s spectrum, and (D) O 1s spectrum of the C-dots. | |
Parameter optimization for amaranth sensing
To obtain an effective and very sensitive fluorescent nanosensor based on C-dots for amaranth sensing, we optimized factors including dosage of C-dots, pH of buffer solution and incubation time. 10 μM amaranth was used in the whole optimization experiments and the quenching efficiency (F0/F) was used as the response of each test.
Fig. S4A† shows the effect of pH on the F0/F in the 2–12 pH range. As the pH increased from 2 to 7, the F0/F initially increased and then showed a decrease trend when pH was higher than 7. The highest F0/F value was achieved at pH 7. This is due to the reaction of the –OH of amaranth, which directly influence the interaction between amaranth and C-dots.51 Therefore, this value was selected as the optimal one for further sensing experiments.
Fig. S4B† shows the effect of the C-dots dosage on the F0/F in the range of 140–280 μL. We found that F0/F increased as the C-dots dosage increased from 140–200 μL and then gradually decreased when the C-dots dosage beyond 200 μL. Thus, we chose 200 μL as the optimum one for further sensing experiments.
Effect of the incubation time between C-dots and amaranth on F0/F value is depicted in Fig. S4C.† The F0/F value reached a maximum when incubation time was 1 min. There was no significant change in F0/F after incubation for 1 min, indicating that the C-dots based nanosensor is fast for the detection of amaranth. In order to ensure the stability and the reproducibility of the experimental results, 5 min was chosen conservatively as the optimized incubation time. In conclusion, the optimum conditions for the detection of amaranth were pH 7, 200 μL of C-dots solution and 5 min incubation time.
Amaranth detection
C-dots fluorescence response to amaranth was analyzed under the optimal conditions discussed above and is displayed in Fig. 4A. As amaranth concentration increased, C-dots fluorescence intensity gradually decreased. It indicated that C-dots fluorescence quenching strongly correlated with amaranth concentration. As displayed by Fig. 4B, C-dots fluorescence quenching positively correlated with amaranth concentration in the 0.2–30 μM range. The relationship was linearly fitted (R2 = 0.9989) using the following equation: F0/F = 0.292[C] + 1. The limit of detection (LOD) and the limit of quantity (LOQ) of the proposed C-dots based nanosensor for amaranth detection was measured to be 0.021 μM (S/N = 3) and 0.07 μM (S/N = 10), respectively.
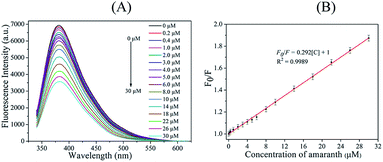 |
| Fig. 4 (A) Fluorescence emission spectra of C-dots with addition of different concentrations of amaranth. (B) The plot of F0/F against amaranth concentration in the range of 0.2–30 μM. The error bars represent the standard deviation of three measurements. | |
For accuracy and precision evaluation, we performed five consecutive analyses of amaranth content at concentrations equal to 1, 15 and 30 μM. As shown by Table S1,† the obtained recoveries were 103%, 102.7% and 98.7% with the corresponding relative standard deviation (RSD) equal to 2.5%, 1.2% and 1.9%, respectively. Thus, our developed novel nanosensor shows excellent precision and accuracy in amaranth detection. Comparison of our sensor performance with other analytical methods of amaranth detection is listed in Table S2.†5,7,8,10–13,52 Our proposed method achieves a lower LOD than other reported methods even though it is not as good as ELISA and HPLC-MS detection methods.7,13 However, it is noteworthy that most other reported analytical methods require long analysis time, costly equipment and complex operations while our proposed method is of rapid response, good accuracy and precision, simple operation and low cost, all of which are beneficial to routine amaranth detection in real samples.
Quenching mechanism analysis
To understand mechanism of C-dots fluorescence quenching by amaranth, we used UV absorption spectra of amaranth and the emission and excitation spectra of C-dots. Absorption spectrum of amaranth overlaps with the emission and excitation spectra of C-dots (Fig. S5A†). This indicated that C-dots fluorescence quenching by amaranth might be attributed to inner filter effect (IFE) or fluorescence resonance energy transfer (FRET).53 For deeper understanding of this quenching mechanism, the time-resolved fluorescence decay spectra of C-dots were measured in the presence and absence of amaranth (Fig. S5B†). The fluorescence lifetime of C-dots did not change with or without amaranth, which points at IFE-based quenching mechanism.54 Meanwhile, the UV absorption spectra of C-dots under different amaranth concentration were recorded (Fig. S5C†). The UV absorption of C-dots at 523 nm gradually increased as amaranth concentration increased. We obtained a good linear correlation between UV absorption intensity and the amaranth concentration, which further confirmed the occurrence of IFE.55 In addition, the structure of amaranth contains hydroxyl groups, and the surface of C-dots contains a large number of hydroxyl and carboxyl groups as determined by IR and XPS, the hydroxyl groups of amaranth bind to the hydroxyl and carboxyl groups of C-dots through hydrogen bonding, allowing an effective non-radiative energy transfer (NRET) from the C-dots to amaranth, resulting in quenching of C-dots.56 So the mechanism of C-dots fluorescence quenching by amaranth was caused by IFE and NRET.
Interference study
To test specificity and selectivity of our developed nanosensor during amaranth analysis in real samples, C-dots fluorescence responses were analyzed under the presence of various potentially-interfering substances such as sugars (glucose, starch and malt sugar), vitamins (vitamin C and vitamin B1), amino acids (glycine, phenylalanine, tyrosine, threonine, alanine and glutamic acid) and salts (KCl, CaCl2, ZnCl2, FeCl3 and NaCl). As shown by Fig. 5, C-dots fluorescence is significantly quenched by amaranth at the concentration of 5 μM. In contrast, presence of other interfering substances even at concentrations 20–60 times higher than amaranth concentration did not show any significant interference on the C-dots fluorescence. Thus, our as-synthesized C-dots can be used to construct highly selective nanosensor to detect amaranth in real samples.
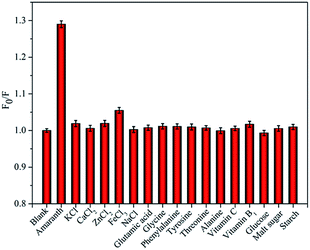 |
| Fig. 5 Fluorescence response of C-dots in the presence of amaranth (5 μM), salts (100 μM), amino acids (300 μM), vitamins (300 μM) and sugars (300 μM). The error bars represent the standard deviation of three measurements. | |
Detection of amaranth in drinks
To further evaluate the practicability and accuracy of our C-dots-based nanosensor, we tested it using grape juice drinks (the main ingredients: water, sugar, citric, vitamin C, sodium carboxymethyl cellulose, aspartame, acesulfame potassium, essence, grape juice and amaranth), which were purchased from a local store and used as received. Table 1 shows the analytical data and recovery values of three drink samples. The recovery values ranged from 96.4% to 103.6% and the RSD (n = 3) was less than 2.8%, which indicates that our developed nanosensor is very accurate and can be used for amaranth detection in consumer beverages.
Table 1 Analytical results of amaranth in grape juice drinks (n = 3)
Sample |
Original found (μM) |
Added (μM) |
Total found (μM) |
Recovery (μM) |
RSD (%) |
1 |
55.1 |
50 |
103.3 |
96.4 |
2.2 |
2 |
54.7 |
100 |
158.3 |
103.6 |
2.8 |
3 |
55.3 |
200 |
251.6 |
98.2 |
1.4 |
Conclusion
We have successfully constructed and applied a new fluorescence nanosensor based on C-dots for the determination of amaranth. This sensor is based on fluorescence quenching of C-dots by amaranth. Specific quenching mechanism was related to the IFE and NRET. C-dots were synthesized using urea and glycine as precursors through a quick, inexpensive and environmentally friendly microwave-assisted method. As-synthesized C-dots display excellent solubility in water and strong fluorescence. The nanosensor constructed using C-dots as active material exhibited excellent selectivity and sensitivity towards amaranth. We obtained a good linear relationship between amaranth concentration and F0/F in the 0.2–30 μM range. Detection limit of amaranth using this method was 0.021 μM. Comparison with previous methods showed that our novel sensor has several advantages, which include its simplicity in design, fabrication and operation, low cost, rapid response, good selectivity and high sensitivity. Furthermore, the as-constructed nanosensor was successfully utilized for the detection of amaranth in consumer beverages. It indicates that the presented nanosensor is a promising sensing platform for amaranth detection.
Conflicts of interest
There are no conflicts to declare.
Acknowledgements
The authors gratefully thank the financial supports by the Doctoral Science Research Fund of Shanxi Datong University under grants No. 2014-B-13 and 2016-B-13, Industry Key Research and Development Plan Project Fund of Datong City under grant No. 2018014 as well as the National Nature Science Foundation of China under grant No. 21375083.
Notes and references
- R. Sarıkaya, M. Selvi and F. Erkoç, Chemosphere, 2012, 8, 974–979 CrossRef PubMed.
- P. Mpountoukas, A. Pantazaki, E. Kostareli, P. Christodoulou, D. Kareli, S. Poliliou, C. Mourelatos, V. Lambropoulou and T. Lialiaris, Food Chem. Toxicol., 2010, 10, 2934–2944 CrossRef PubMed.
- J. Zhang, M. L. Wang, C. S. Tu, W. C. Wang and Z. D. Chen, Food Chem., 2014, 160, 11–15 CrossRef CAS PubMed.
- K. S. Minioti, C. F. Sakellariou and N. S. Thomaidis, Anal. Chim. Acta, 2007, 583, 103–110 CrossRef CAS PubMed.
- G. Karanikolopoulos, A. Gerakis, K. Papadopoulou and I. Mastrantoni, Food Chem., 2015, 177, 197–203 CrossRef CAS PubMed.
- H. Wu, J. B. Guo, L. M. Du, H. Tian, C. X. Hao, Z. F. Wang and J. Y. Wang, Food Chem., 2013, 141, 182–186 CrossRef CAS PubMed.
- X. H. Chen, Y. G. Zhao, H. Y. Shen, L. X. Zhou, S. D. Pan and M. C. Jin, J. Chromatogr. A, 2014, 1346, 123–128 CrossRef CAS PubMed.
- J. Ju and L. P. Guo, Chin. J. Anal. Chem., 2013, 41, 681–686 CrossRef CAS.
- Y. D. Gao, L. Wang, Y. L. Zhang, L. N. Zou, G. P. Li and B. X. Ye, Talanta, 2017, 168, 146–151 CrossRef CAS PubMed.
- J. Zhang, M. L. Wang, C. Shentu, W. C. Wang and Z. D. Chen, Food Chem., 2014, 160, 11–15 CrossRef CAS PubMed.
- M. Ryvolová, P. Táborský, P. Vrábel, P. Krásenský and J. Preisler, J. Chromatogr. A, 2007, 1141, 206–211 CrossRef PubMed.
- F. I. Andrade, M. I. F. Guedes, Í. G. P. Vieira, F. N. P. Mendes, P. A. S. Rodrigues, C. S. C. Maia, M. M. M. Ávila and L. M. Ribeiro, Food Chem., 2014, 157, 193–198 CrossRef PubMed.
- B. Zhang, D. L. Du, M. Meng, S. A. Eremin, V. B. Rybakov, J. H. Zhao, Y. M. Yin and R. M. Xi, Food Anal. Methods, 2014, 7, 1498–1505 CrossRef.
- S. Qian, L. Qiao, W. Xu, K. Jiang, Y. Wang and H. Lin, Talanta, 2019, 194, 598–603 CrossRef CAS PubMed.
- C. Qu, D. Zhang, R. Yang, J. Hu and L. Qu, Spectrochim. Acta, Part A, 2019, 206, 588–596 CrossRef CAS PubMed.
- S. Y. Lim, W. Shen and Z. Q. Gao, Chem. Soc. Rev., 2015, 44, 362–381 RSC.
- S. Mondal, R. Thirupathi and H. S. Atreya, RSC Adv., 2015, 5, 4489–4492 RSC.
- X. Y. Xu, R. Ray, Y. L. Gu, H. J. Ploehn, L. Gearheart, K. Raker and W. A. Scrivens, J. Am. Chem. Soc., 2004, 126, 12736–12737 CrossRef CAS PubMed.
- F. Wang, S. Pang, L. Wang, Q. Li, M. Kreiter and C.-Y. Liu, Chem. Mater., 2010, 22, 4528–4530 CrossRef CAS.
- H. Goncalves, P. A. S. Jorge and J. R. A. Fernandes, Sens. Actuators, B, 2010, 145, 702–707 CrossRef CAS.
- Y. Li, Y. Hu, Y. Zhao, G. Shi, L. Deng, Y. Hou and L. Qu, Adv. Mater., 2011, 23, 776–780 CrossRef CAS PubMed.
- X. Xu, R. Ray, Y. Gu, H. J. Ploehn, L. Gearheart, K. Raker and W. A. Scrivens, J. Am. Chem. Soc., 2004, 126, 12736–12737 CrossRef CAS PubMed.
- L. Tang, R. Ji, X. Cao, J. Lin, H. Jiang, X. Li, K. S. Teng, C. M. Luk, S. Zeng and J. Hao, ACS Nano, 2012, 6, 5102–5110 CrossRef CAS PubMed.
- Y. Chen, Y. Wu, B. Weng, B. Wang and C. Li, Sens. Actuators, B, 2016, 223, 689–696 CrossRef CAS.
- X. Wang, K. Qu, B. Xu, J. Ren and X. Qu, J. Mater. Chem., 2011, 21, 2445–2450 RSC.
- H. Zhu, X. L. Wang, Y. L. Li, Z. J. Wang, F. Yang and X. R. Yang, Chem. Commun., 2009, 34, 5118–5120 RSC.
- S. Y. Lim, W. Shen and Z. Gao, Chem. Soc. Rev., 2015, 44, 362–381 RSC.
- F. Lu, Y. Song, H. Huang, Y. Liu, Y. Fu, J. Huang, H. Li, H. Qu and Z. Kang, Carbon, 2017, 120, 95–102 CrossRef CAS.
- Q. Xu, T. Kuang, Y. Liu, L. Cai, X. Peng, T. S. Sreeprasad, P. Zhao, Z. Yu and N. Li, J. Mater. Chem. B, 2016, 4, 7204–7219 RSC.
- J. Bhamore, S. Jha, T. J. Park and S. K. Kailasa, Sens. Actuators, B, 2018, 277, 47–54 CrossRef CAS.
- S. K. Kailasa, S. Ha, S. H. Baek, L. M. T. Phan, S. Kim, K. Kwak and T. J. Park, Mater. Sci. Eng., C, 2019, 98, 834–842 CrossRef CAS PubMed.
- V. Mehta, S. Jha and S. K. Kailasa, Mater. Sci. Eng., C, 2014, 38, 20–27 CrossRef CAS PubMed.
- Z. Ma, Y.-L. Zhang, L. Wang, H. Ming, H. Li, X. Zhang, F. Wang, Y. Liu, Z. Kang and S.-T. Lee, ACS Appl. Mater. Interfaces, 2013, 5, 5080–5084 CrossRef CAS PubMed.
- T. Feng, X. Ai, G. An, P. Yang and Y. Zhao, ACS Nano, 2016, 10, 4410–4420 CrossRef CAS PubMed.
- E. G. Ju, Z. Liu, Y. D. Du, Y. Tao, J. S. Ren and X. G. Qu, ACS Nano, 2014, 8, 6014–6023 CrossRef CAS PubMed.
- S. Sooksin, V. Promarak, S. Ittisanronnachai and W. Ngeontae, Sens. Actuators, B, 2018, 262, 720–732 CrossRef CAS.
- Y. Zhang, X. Fang, H. Zhao and Z. Li, A highly sensitive and selective detection of Cr(VI) and ascorbic acid based on nitrogen-doped carbon dots, Talanta, 2018, 181, 318–325 CrossRef CAS PubMed.
- M. Zan, L. Rao, H. Huang, W. Xie, D. Zhu, L. Li, X. Qie, S.-S. Guo, X.-Z. Zhao, W. Liu and W.-F. Dong, Sens. Actuators, B, 2018, 262, 555–561 CrossRef CAS.
- D. K. Dang, S. Chandrasekaran, Y.-L. T. Ngo, J. S. Chung, E. J. Kim and S. H. Hur, Sens. Actuators, B, 2018, 255, 3284–3291 CrossRef CAS.
- H. Zeng, L. Li, Y. Ding and Q. Zhuang, Talanta, 2018, 178, 879–885 CrossRef CAS PubMed.
- L. Lin, M. Rong, S. Lu, X. Song, Y. Zhong, J. Yan, Y. Wang and X. Chen, Nanoscale, 2015, 7, 1872–1878 RSC.
- L. Lin and S. Zhang, Chem. Commun., 2012, 48, 10177–10179 RSC.
- C. Hu, Y. Liu, Y. Yang, J. Cui, Z. Huang, Y. Wang, L. Yang, H. Wang, Y. Xiao and J. Rong, J. Mater. Chem., 2013, 1, 39–42 RSC.
- Y. Dong, H. Pang, H. B. Yang, C. Guo, J. Shao, Y. Chi, C. M. Li and T. Yu, Angew. Chem., Int. Ed., 2013, 125, 7954–7958 CrossRef.
- S. J. Zhu, Q. N. Meng, L. Wang, J. H. Zhang, Y. B. Song, H. Jin, K. Zhang, H. C. Sun, H. Y. Wang and B. Yang, Angew. Chem., Int. Ed., 2013, 52, 3953–3957 CrossRef CAS PubMed.
- Q. Hu, M. C. Paau, Y. Zhang, X. Gong, L. Zheng, D. Lu, Y. Liu, Q. Liu, J. Yao and M. M. F. Choi, RSC Adv., 2014, 4, 18065–18073 RSC.
- Q. Xu, Y. Liu, C. Gao, J. Wei, H. Zhou, Y. Chen, C. Dong, T. S. Sreeprasad, N. Li and Z. Xia, J. Mater. Chem. C, 2015, 3, 9885–9893 RSC.
- S. Chandra, P. Patra, S. H. Pathan, S. Roy, S. Mitra, A. Layek, R. Bhar, P. Pramanik and A. Goswami, J. Mater. Chem. B, 2013, 1, 2375–2382 RSC.
- Y. Liu, Q. Zhou, Y. Yuan and Y. Wu, Carbon, 2017, 115, 550–560 CrossRef CAS.
- F. Yan, D. Kong, Y. Luo, Q. Ye, J. He, X. Guo and L. Chen, Microchim. Acta, 2016, 183, 1611–1618 CrossRef CAS.
- W. P. Hu, G. D. Cao, W. Dong, H. B. Shen, X. H. Liu and L. S. Li, Anal. Methods, 2014, 6, 1442–1447 RSC.
- S. P. Alves, D. M. Brum, É. C. B. Andrade and A. D. P. Netto, Food Chem., 2008, 107, 489–496 CrossRef CAS.
- H. Liu, C. Xu, Y. Bai, L. Liu, D. Liao, J. Liang, L. Liu and H. Han, Spectrochim. Acta, Part A, 2017, 171, 311–316 CrossRef CAS PubMed.
- Y. Z. Fan, Y. Zhang, N. Li, S. G. Liu, T. Liu, N. B. Li and H. Q. Luo, Sens. Actuators, B, 2017, 240, 949–955 CrossRef CAS.
- Z. Zhang, Y. Liu, Z. Yan and J. Chen, Sens. Actuators, B, 2018, 255, 986–994 CrossRef CAS.
- G. H. G. Ahmed, R. B. Laíño, J. A. G. Calzón and M. E. D. García, Talanta, 2015, 132, 252–257 CrossRef CAS PubMed.
Footnote |
† Electronic supplementary information (ESI) available. See DOI: 10.1039/c9ra04494a |
|
This journal is © The Royal Society of Chemistry 2019 |
Click here to see how this site uses Cookies. View our privacy policy here.