DOI:
10.1039/C9RA04711E
(Paper)
RSC Adv., 2019,
9, 31013-31020
Synthesis of silver particles stabilized by a bifunctional SiHx–NHy–PMHS oligomer as recyclable nanocatalysts for the catalytic reduction of 4-nitrophenol†
Received
23rd June 2019
, Accepted 28th August 2019
First published on 1st October 2019
Abstract
Bifunctional oligomers with both reducing and stabilizing functionalities were prepared and successfully applied to the preparation of silver colloids of around 2 nm size without employing a strong stabilizer such as S and P, which was quite difficult to achieve. Transmission electron microscopy (TEM) and dynamic light scattering (DLS) were performed to determine the morphology and particle size of the Ag colloids. UV-vis spectroscopy and X-ray absorption spectroscopy (XAS) were implemented to investigate the oxidation state of the Ag colloids. Synthesis parameters such as the density control of the ligating functionalities, the propinquity of the reducing and stabilizing groups, the extent of ligand stabilization and the reducing rates were found to have important effects on the formation and stabilization of Ag colloids. The as-synthesized Ag colloids were very stable even after being deposited on silica; then, they were subjected to calcination to get rid of the organics, which afforded Ag NPs (1.9–3.5 nm) on silica with narrow size distribution. These Ag NPs performed excellently in catalytic 4-nitrophenol reduction with conversion of up to 98% within 10 min. Furthermore, the Ag nanoparticles were quite stable and exhibited excellent reusability for seven successive reaction cycles without obvious decay. The straightforward synthesis of the ultra-small and stable Ag NPs has the potential for applications in the synthesis of other supported late transition metals.
Introduction
Nanoparticles of noble metals, especially metal nanoparticles in the quantum-size domain, have attracted tremendous attention due to their extensive applications in electronics, optics, catalysis, and biomedical and environmental fields.1–3 Silver nanoparticles have been widely studied due to their attractive spectrum of physical properties and their significant potential for selective activity in a very wide range of applications including antimicrobial agents, wastewater purification, electronics, and biological sensing and imaging.4–9
Among these applications, the employment of silver NPs in sewage treatment is of great significance due to the intrinsic electronic properties and particular catalytic performance. For example, 4-nitrophenol (4-NP) is one of the most toxic pollutants that exists widely in both industrial and agriculture effluents such as petrochemicals and pharmaceuticals.10 It is listed as a “priority pollutant” since it can hardly be removed by natural degradation and is genotoxic and carcinogenic to animals and humans.11,12 The catalytic reduction of 4-NP over noble-metal NPs has been demonstrated to be an efficient and eco-friendly procedure for producing 4-aminophenol (4-AP), which is an important intermediate for fine chemicals such as synthetic dyes, pharmaceuticals and pesticides.10,13 Therefore, the reduction of 4-NP to 4-AP was chosen as a model reaction to quantitatively evaluate the catalytic properties and consider pollutant treatment and green regeneration.14,15
The intrinsic properties of catalytic silver nanoparticles are predominantly determined by their size, shape, structure, composition and crystallinity. However, silver NPs are thermodynamically unstable and are inclined to agglomerate to minimize the surface energy, resulting in a significant decrease in catalytic activity. Colloidal silver particles of less than 2 nm size have shown many extraordinary properties since their size is fairly close to the Fermi wavelength. It has been demonstrated that there exists a strong size effect on the catalytic performance in this range.16–19 Jiang20 investigated the catalytic oxidation of CO on the Ag38 cluster supported by graphdiyne and provided intensive understanding of its high activity originating from the intrinsic activity of the Ag38 cluster. Zamborini and co-workers addressed the size-dependent electrochemical oxidation of silver nanoparticles as a function of particle size.21 In view of the strong correlation between stability, particle size and catalytic properties, great efforts have been made to design strategies to prepare small silver colloids, especially those less than 2 nm in size.
The concept of stabilizing silver nanoparticles is one of the potential strategies for conquering the aggregation problem. In most cases, stabilized nanoparticles are coordinated with strong surfactants to prevent agglomeration and they might show specific surface properties;22 therefore, the selection of a stabilizer is crucial. Assuming that the stabilizer interacts weakly with Ag, the Ag atoms will migrate to form large Ag particles. Likewise, if the stabilizer interacts strongly with Ag, it could alter the surface nature of the particles so as to affect the catalytic performance significantly. Ideally, it is desirable to develop synthetic strategies to synthesize silver nanoparticles, which provide flexibility for functionalizing the nanoparticles according to the need. Many reports have shown that bifunctional stabilizers have been applied successfully in many cases such as polyoxometalates,23 dithiocarboxylates,24 dithiocarbamates,25 mercaptosuccinic acid,26 2-mercaptopropionic acid,27 mercaptocarbonyl compounds28 and ethylenediamine tetraacetate.29 As for the bifunctional stabilizers, one functional group is ligated to the silver particle, and the other one offers a charged layer that can stabilize the particles and prevent them from aggregating by charge–charge repulsion. Ultimately, we would like to synthesize stable silver particles with high catalytic activity, but there are two crucial problems worth considering. First, both sulfur and phosphorus have strong chemical interactions with Ag, which can influence the chemical properties of the surface atoms. Thus, the activation of silver catalyst often need harsh treatment to remove the stabilizer which will lead to notable coarsening of the silver particles. Second, it is accepted that residual S or P can poison the Ag catalysts in the presence of stable sulfates or phosphates.30,31 Thus, there is a strong potential to identify bifunctional stabilizers that on the one hand interact more weakly with Ag as compared with S and P and on the other hand can ligate and stabilize silver nanoparticles with narrow size distribution.
Herein, bifunctional SiHx–NHy–PMHS oligomers were synthesized and used to successfully prepare stable and uniform silver colloids smaller than 2 nm without using a strong stabilizer such as S and P, which is known to be difficult to accomplish. Significantly, by designing both reducing and stabilizing groups in a single oligomer, their interactions with the silver precursor and the proximity of the functional groups could be manipulated, so that the reduction and nucleation processes of silver nanoparticles could be controlled at the atomic level as well as in the subsequent stabilization process. We designed and synthesized a functionalized oligomer as a stabilizer containing two types of groups for the fabrication of colloidal Ag with the size of around 2 nm. The –SiH groups functioned as the reducing agent, the –NH groups were coordinated to the surface Ag atoms, and the particles were stabilized from agglomeration. Besides, the ratio of the two ligands could be adjusted by a hydrolytic process. Furthermore, another transition metal could be incorporated into the resulting colloids, which could be potentially used to design and synthesize binary noble catalysts. Subsequently, the preparation process was monitored by recording the NMR spectra in solution, and we obtained deep insights into the synthesis parameters that determined the morphology of silver colloids. We confirmed the essential proximal location of the –NH groups and the reducing SiH ligand; we also established that the density of the ligating groups, the proximity of the ligating and reducing groups and the rates of reduction have a strong effect on the particle sizes of silver colloids. The as-synthesized Ag colloids were very stable even after being deposited on silica; subsequently, they were calcined to burn the organics in He and O3 atmospheres, which afforded Ag NPs (1.9–3.5 nm) on silica with narrow size distribution. Finally, the reduction of 4-nitrophenol to 4-aminophenol with excess NaBH4 was employed as a probe reaction to screen the activities of these AgNPs. The reaction kinetics were investigated by monitoring the time-dependent conversion of 4-nitrophenol in the system. Moreover, these catalysts could be recycled for seven successive cycles without obvious decay, indicating that this is a promising method for fabricating stable silver catalysts for 4-nitrophenol treatment in large-scale processes. This novel and facile method provides potential applications for the design of heterogeneous catalysts and can furnish grounds for fundamental catalysis research.
Experimental
Materials
All the reagents and solvents were purchased from commercial companies and were used as received without further purification. Poly(methylhydro)siloxanes (PMHS I, 1700–3200 g mol−1), palladium hydroxide on carbon (30 wt% loading), silver acetate (99.0%), 4-nitrophenol (≥99.0%), sodium borohydride (≥99.0%), toluene (anhydrous, 99.8%), tetrahydrofuran (99.9%), ethyl ether (≥99.0%), calcium sulfate (drierite, 8 mesh), triethylsilane (99%) were purchased from Sigma-Aldrich. N-Methyl-aza-2,2,4-trimethylsilacyclopentane was purchased from Gelest. Celite and sodium sulfate (≥99.0%) were purchased from EMD Chemicals. Experiments referring to compounds II and III were performed under N2 protection either in an N2 glovebox or using a Schlenk line.
Synthesis of bifunctional oligomer SiHx–NHy–PMHS
Scheme 1 shows the steps in the synthesis of the bifunctional amino hydrosiloxane SiHx–NHy–PMHS. First, 0.64 mL PMHS I containing 10 mmol SiH groups was added to a Schlenk flask that was preloaded with 20 mL THF. Quantitative H2O (90 mg, 180 mg, 300 mg) was added to partially or totally oxidize the –SiH groups to –SiOH groups in the presence of Pearlman's catalyst to form SiHx–SiOHy–PMHS (II or III). The reaction was monitored by the H2 evolution. Once the H2 evolution ceased, the remaining mixture was dried thoroughly with sodium sulfate. Column chromatography was performed to remove the Pearlman's catalyst, in which sandwiched Celite and Drierite were layered using diethyl ether as the eluent. After that, the –SiOH groups of synthesized II or III were quantitatively reacted with evaporation to generate the desired SiHx–NHy–PMHS oligomer IV or N-methyl-aza-2,2,4-trimethylsilacyclopentane under N2 protection. The mixture was stirred for around 30 min followed by the addition of solvent V. The as-synthesized compounds were characterized with NMR spectra; they are shown in Fig. S1 and S2.†1H NMR (400 MHz, CDCl3) δ 0–0.015 (–Si(CH3)3, –Si(CH3)2, –SiHCH3, –SiCH3); 0.38 (–SiCH2); 0.63 (–SiCH2); 0.84 (–NH); 0.92 (CH3CH–); 1.80 (CH3CH–); overlapped 2.43 (–NHCH2, –NHCH3); 4.8(SiH–); 7.26 (CDCl3).13C NMR (100 MHz, CDCl3) δ −2.64 (–Si(CH3)3); 1.09 (–SiCH3); 1.37 (–Si(CH3)2); 20.96 (CH3CH–); 24.55 (–SiCH2); 29.09 (CH3CH–); 36.58 (–NHCH3); 61.64 (–NHCH2); 77.00 (CDCl3). 29Si NMR (80 MHz, CDCl3) δ −66.91 (–SiCH3); −36.60 (–SiHCH3); 6.32 (–Si(CH3)3); 7.97 (–Si(CH3)2).
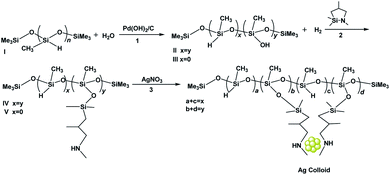 |
| Scheme 1 Synthesis of the bifunctional SiHx–NHy–PMHS oligomer for Ag colloid preparation. | |
Synthesis of silver colloids with as-synthesized bifunctional oligomer
Synthesis of amine-functionalized silver colloids. Quantitative SiHx–NHy–PMHS was dissolved in THF solution in an ice bath. Then, aliquots of 6 mM AgNO3 solution in methanol were added to the vial. In these experiments, the amounts of oligomer and AgNO3 added were calculated with respect to the –SiH or –NH group. When –SiH was not an internal portion of the oligomer, a mild reductant, triethylsilane, was introduced into the system. Different ratios of SiH/NH/Ag were examined, which are shown in Table 1. The total amount of the mixture was maintained at 10 mL and the silver concentration was maintained at 3 mM finally. The reaction was kept for one hour at 0 °C. Silica-supported silver nanoparticles were made by grafting Ag colloids onto Cabosil-L90 immersed in THF and then calcined in He and O3/O2 atmospheres in sequence at 150 °C.
Table 1 Average Ag particle size in different preparations
Sample |
H2O addeda(mg) |
Amine and silane carrier |
Synthesis solution conditionsb |
Ag colloid diametere (nm) |
[Olig]c (mM) |
[Amine]d(mM) |
Ag/SiH |
T (°C) |
Quantitative H2O was added to partially or totally oxidize SiH to silanols in the presence of Pearlman's catalyst.
N/Ag atomic ratio was 10.
Based on an average of 38 siloxane units per oligomer.
Calculated using the extent of conversion of SiH to amino units.
Based on 300 particles.
|
A |
0 |
PMHS I |
0.8 |
— |
1 : 10 |
0 |
10.5 ± 3.3 |
B |
90 |
SiH–NH–PMHS V |
1.6 |
30 |
1 : 10 |
0 |
1.2 ± 0.4 |
C |
180 |
Et3SiH + NH–PMHS IV |
0.8 |
30 |
1 : 3 |
0 |
2.5 ± 0.9 |
D |
300 |
Et3SiH + NH–PMHS IV |
0.8 |
30 |
1 : 10 |
0 |
3.6 ± 1.5 |
E |
90 |
SiH–NH–PMHS V |
1.6 |
30 |
1 : 10 |
25 |
5.4 ± 1.94 |
Sample characterization
NMR spectra were collected with an Agilent DD2 spectrometer and the data were processed with MNova 9.0 software. The morphologies of Ag colloids were examined using the TEM model on a JEOL 2100-F transmission electron microscope. High angle annular dark-field STEM images were obtained with a field emission gun at 200 kV. Particle size distribution was analyzed by counting more than 300 particles based on the images. Dynamic light scattering spectra were obtained with Brookhaven BI9000, which could measure the hydrodynamic diameters of the Ag colloids. A Thermo ESCALAB 250Xi instrument was used to collect X-ray photoelectron spectra. It was equipped with an electron flood gun using Al Kα radiation (1486.6 eV). An HP8452 diode array spectrophotometer was used to acquire the UV-vis absorption spectra. Beamline 5-BMD was used to obtain X-ray absorption spectra in the fluorescence mode. The data were collected at a temperature of about 25 K, using a helium cryostat, in order to reduce the Debye–Waller effects. Silver foil and silver nitrate were applied as the standards. Demeter was used to analyze the XAS data.
Catalytic test of the as-synthesized catalysts
The reduction of 4-NP with excess NaBH4 solution was used as a model reaction to quantitatively evaluate the catalytic activity of the silver nanocatalysts. The reaction kinetics did not depend on the concentration of the NaBH4 solution. Once NaBH4 was added to the 4-NP solution, the color immediately changed from light yellow to yellow-green due to the formation of 4-nitrophenolate ions in the solution. Then, the yellow-green color gradually faded over time after introducing silver nanocatalysts due to the conversion of 4-NP to 4-AP. The reduction process was monitored by UV-vis spectra collected at different time intervals.
Typically, 40 mL mixed aqueous solution containing 3 mM 4-NP and 100 mM NaBH4 was prepared. Then, the Ag nanocatalyst (containing 0.005 mmol Ag) was added to the reaction with continuous stirring to form a suspension at room temperature. At appropriate time intervals, an aliquot of the reaction mixture was taken out with a syringe and filtered with membrane filters. The UV-vis absorption spectrum of the filtrate was recorded immediately. The reaction rate was measured based on the absorbance intensity of the 4-nitrophenolate ion peak at 400 nm as a function of time.
When studying the recycling of the active catalyst Ag/SiO2-1 system, the solution obtained after the reaction was filtered with a membrane filter and the solid was washed with deionized water for several times. After that, the recovered catalyst was dried in the oven at 80 °C for the next run under the same conditions.
Results and discussion
Interaction of AgNO3 with the bifunctional oligomer SiHx–NHy–PMHS
The resonances of the protons of the Ag colloid and the corresponding oligomers are shown in Fig. 1. The two protons of NH–CH2– were diastereotopic and appeared as broad and multiplet peaks together with the NH–CH3 resonance at around 2.3–2.4 ppm. Based on the integrated areas of NH–, NH–CH2– and NH–CH3, we inferred that the integration accounted for the majority of the protons associated with the amine groups in the oligomer. The existence of the –SiH groups in oligomer IV resulted in the immediate reduction of Ag(I) after mixing with the Ag precursor. The following remarkable changes were observed and the resonance shifted from δ 0.85 to 2.5 ppm (Fig. 1A and B). The overlapping NH–CH2– and NH–CH3 peaks now separated into two peaks: a relatively broader one at δ 2.38 ppm and a sharper one at δ 2.48 ppm in the resonance peaks of NH–, NH–CH2– and NH–CH3. The NH– peak was at 2.48 ppm. However, the addition of Et3SiH in oligomer V gave rise to the reduction of Ag(I) after mixing with the Ag precursor and obvious variations in the peaks of NH–, NH–CH2– and NH–CH3. The NH– resonance shifted from δ 0.85 to 1.25 ppm (Fig. 1C and D). A new broad peak that appeared at 2.9 ppm (Fig. 1D) could be assigned to NH–CH2– and NH–CH3 of oligomer IV, which coordinated to Ag(I). The overall prominent shifts demonstrated that the structure was altered in oligomers IV and V. We inferred that Ag(I) was reduced by SiH and the formed compound could easily react with a neighboring NH– group in the form of a new silazane bond accompanied by the protonation of another amine group.32 The proposed equations are listed below. These structural modifications gave rise to the observed spectroscopic shifts and essentially reflected the conformational changes in the oligomer. | –NHR + AgNO3 = Ag(NHR)NO3 | (1) |
| 2(NHR)AgINO3 + 2R3SiH = 2NHRAg0 + 2R3Si(NO3) + H2 | (2) |
| R3Si(NO3) + 2NHR2 = R3SiNR2 + R2(NH2)+(NO3)− | (3) |
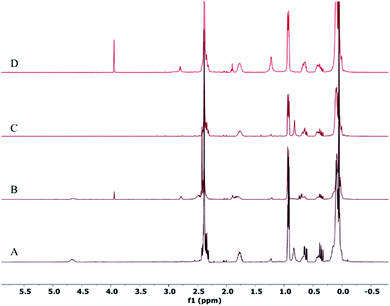 |
| Fig. 1
1H NMR spectra of Ag colloid samples after the addition of AgNO3 to SiHx–NHy–PMHS IV and V. (A–D) Correspond to SiHx–NHy–PMHS IV, Ag-IV, V and Ag-V. | |
Characterization of silver colloids synthesized with bifunctional oligomers
The TEM images of samples A to E are shown in Fig. 2. Not surprisingly, sample A showed large non-uniform Ag agglomerates larger than 10 nm. This is not difficult to understand since this sample was prepared without amine ligands on the backbone of the oligomer, which demonstrated that amine ligands are imperative to stabilize small Ag colloids. For sample B, the predominant Ag particles were around 1.2 nm in diameter, while for samples C and D, the Ag particles were around 2.5–3.6 nm in diameter. For sample B, it was observed that the particle size of the Ag colloids (Table 1) was smaller (compared with those for C and D) and the uniformity was much higher. The difference was that the sample B was prepared with a bifunctional oligomer possessing internal reducing (–SiH) groups and ligating (amine) groups. However, samples C and D were synthesized with oligomers containing amine groups only, and the external reducing –SiH groups were added in the process. It can be deduced that the influence of the proximity of the silane and amine groups on the morphology of Ag colloids might be important; the more proximal the reducing groups and ligating groups, the smaller the silver particle size and distribution. More importantly, using a relatively lower concentration of triethylsilane as the external reducing agent resulted in much smaller particles of Ag colloids (compared with samples C and D). A lower concentration of the reductant probably led to a much slower reduction rate of the reaction. This allowed the amine groups to coordinate with Ag atoms more effectively to prevent Ag from agglomeration to some degree. This demonstrated that the reduction rate was quite important for controlling the morphology of Ag colloids. This result was consistent with the outcomes that the Ag colloids made at 0 °C were much smaller and more narrowly distributed than the samples made at 25 °C (compared with samples B and E). It was easy to understand that either the mobility of the Ag atoms or the reduction rate of the reaction was expected to be much slower at a relatively lower temperature, which could generate smaller Ag colloids.
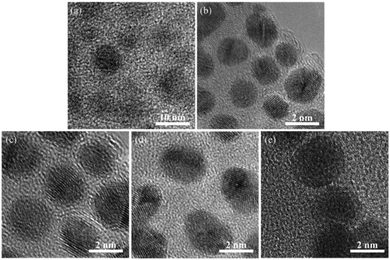 |
| Fig. 2 TEM images of Ag colloid. (a–e) Correspond to sample A to E in Table 1. | |
Apart from the TEM images, another measurement was also obtained to characterize the Ag colloids. Dynamic light scattering can provide information about the hydrodynamic diameters of the obtained colloids. The data are shown in Fig. 3; the data indicated that the average particle sizes of the colloids were 11.7, 1.9, 3.4, 3.9, and 6.1 nm for samples A–E, respectively, which exhibited the same trend as that observed in the TEM images. The data provided by dynamic light scattering reflected the particle size of the entire colloid in addition to that of the sole Ag particles. In other words, actually, the Ag particles were embedded in a shell of the functionalized oligomer. It was not surprising that the particle sizes determined by DLS were somewhat larger than those derived from the TEM images since the data from TEM represented the individual Ag particle size rather than that of the whole colloid.
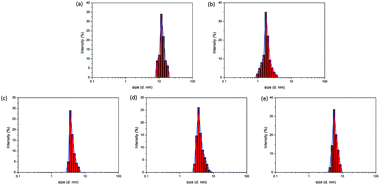 |
| Fig. 3 DLS data for the Ag colloids. (a–e) Correspond to samples A to E in Table 1. | |
UV-visible spectroscopy was performed as well to characterize the Ag colloids. As shown in Fig. 4, no distinct absorption peaks appear for the polymer shell in the visible region, while Ag colloids under a different preparation process exhibit a wide absorption band in the visible light region centered around 400–420 nm. This is the characteristic absorption for the surface plasmon resonance (SPR) peaks of Ag NPs.33–36 For the sample B (spectrum a), the SPR peak at approximately 400 nm was the widest, indicating that the silver nanostructures were the smallest. The obvious red shift in the absorption band of Ag NPs (from a to c) indicated that the particle size gradually increased, causing the SPR peaks to narrow and increase in intensity.37 These observations were consistent with the trends determined by TEM.
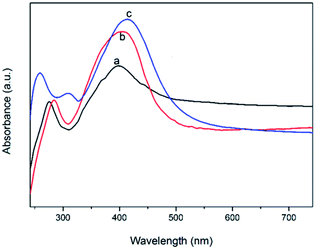 |
| Fig. 4 UV-visible spectra of Ag colloid. (a–c) Correspond to samples B–D in Table 1. | |
Ag XANES was performed to investigate the oxidation states of Ag by the intensity of a white line at the edge energy. Fig. 5 shows the XANES spectra of the silver colloids with the standard silver foil and silver nitrate. The silver nitrate absorption edge appeared at higher energy than that of the silver foil due to the oxidation state of Ag+. Since the Ag atom in silver nitrate lost a “d” electron, the “1s” orbital electrons were deshielded from the nucleus; therefore, their binding energy increased.38 The spectra obtained from samples B to D were very similar, and their absorption edges appeared at the same energy as that of the silver foil. No intense white line characteristic of AgNO3 was observed. This information confirmed that the samples were completely reduced and that silver existed as Ag(0), which agreed well with the UV-vis results.
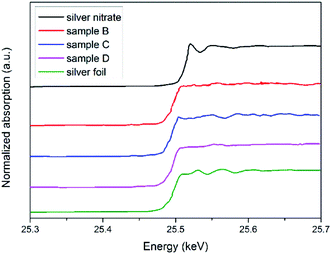 |
| Fig. 5 Normalized absorption spectra at the Ag K-edge. From top to bottom, silver nitrate, sample B, sample C, sample D, silver foil (Ag0), respectively. | |
Characterization of silica-supported Ag nanoparticles
The Ag colloid with the smallest particle size and the narrowest distribution (sample B) was prepared to synthesize supported Ag/SiO2 nanoparticles. The colloidal particles were deposited onto silylated silica and calcined in He and O3/O2 at 150 °C, which could burn and get rid of all the organics and thus convert siloxane into SiO2.39 Among the samples, the Ag loadings were 1 wt%, 2 wt%, 3 wt%, and 4 wt%; the samples were denoted as Ag/SiO2-1, Ag/SiO2-2, Ag/SiO2-3, and Ag/SiO2-4, respectively.
XPS analysis was conducted to further elucidate the surface composition of the silica-supported Ag nanoparticles. For all the Ag/SiO2 samples, there was no detectable Cl, N or S by XPS in any of the samples. The peaks with binding energies of 282.7, 530.3, 100.7 and 366.3 eV were attributed to C 1s, O 1s, Si 2p and Ag 3d, respectively, indicating the presence of the C, O, Si and Ag elements in the samples (Fig. S3†). Higher-resolution spectra were obtained to investigate the electronic states of the elements. In the spectrum of Ag 3d in Fig. 6, two peaks centered at 368.2 and 374.2 eV with a spin–orbit separation of 6 eV can be assigned to Ag 3d5/2 and Ag 3d3/2, respectively. These results further verified that only metallic Ag oxidation states were present in the supported Ag samples.40 Furthermore, the intensity of Ag 3d increased with the increase in Ag loading, which was consistent with the results of our experiments.
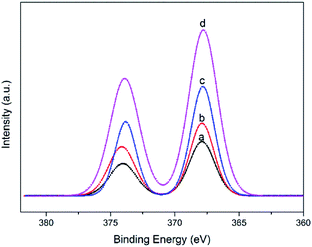 |
| Fig. 6 XPS spectra of Ag 3d: (a–d) correspond to Ag/SiO2-1, Ag/SiO2-2, Ag/SiO2-3, Ag/SiO2-4. | |
The STEM images of Ag/SiO2-1, Ag/SiO2-2, Ag/SiO2-3, and Ag/SiO2-4 are shown in Fig. 7. The particle size of the Ag colloid used for the preparation of catalysts was 1.2 ± 0.4 nm, as shown in Fig. 2. The Ag particle sizes of the catalysts gradually increased from 1.9 nm to 3.5 nm as the silver loading increased from 1 wt% to 4 wt%. The reason was that the Ag particles in these samples were surrounded by siloxane oligomers and the silver loading increased as the organics increased. We had to remove the organics after the thermal treatment to achieve the Si–O–Si cluster around the Ag nanoparticles so as to prevent the Ag particles from agglomerating to some degree. During calcination, the organics served as the fuel to cause local heating. The initial growth of Ag clusters probably occurred within the same Ag-PMHS particle. With more organics being burnt and more overheating, collisions between particles occurred, where interparticle exchange of Ag particles took place and this enabled their subsequent agglomeration. It can be concluded that the Ag particles prepared in this method were highly dispersed and were relatively stable under thermal conditions.
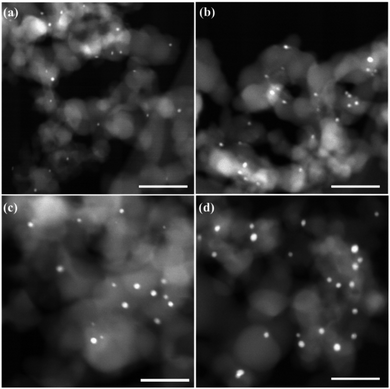 |
| Fig. 7 STEM images of Ag/SiO2. (a–d) Correspond to Ag/SiO2-1, Ag/SiO2-2, Ag/SiO2-3, Ag/SiO2-4. Scale bar is 20 nm. | |
Catalytic performance of Ag/SiO2 nanoparticles
The catalytic reduction of 4-NP in the presence of NaBH4 was chosen as a model reaction to quantitatively evaluate the catalytic activity of the prepared Ag/SiO2 samples. Although the reaction is thermodynamically favorable, it can hardly be triggered without catalysts. It has been widely applied to evaluate the catalytic activities of various metallic NPs.41,42 The reaction can be monitored in real-time by UV-vis absorption spectroscopy. The 4-NP solution exhibited a strong absorption peak at 317 nm in neutral or acidic conditions. Upon the addition of the NaBH4 solution, the characteristic peak of 4-NP immediately shifted from 317 to 400 nm (Fig. 8a), accompanied with a color change from light yellow to yellow-green due to the formation of the 4-nitrophenolate ions.43,44 Notably, there was no change in the maximum absorption over time even after the superfluous addition of the NaBH4 solution. The reduction of 4-NP did not occur without the use of Ag NPs as the catalysts. After the Ag/SiO2-1 nanoparticles were added, the characteristic absorption peak of the 4-nitrophenolate ions at 400 nm gradually decayed, while a new peak at 295 nm, ascribed to the only product 4-AP, appeared (Fig. 8b). Meanwhile, the color of the 4-nitrophenolate ions gradually diminished within 10 min.
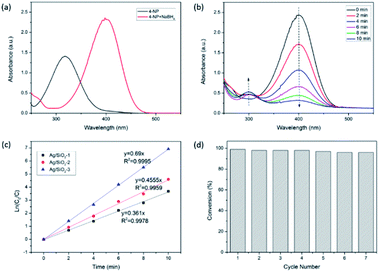 |
| Fig. 8 The catalytic performance of the assembled Ag/SiO2 NPs towards the reduction of 4-NP. (a) UV-vis spectra of the 4-NP solution after the addition of NaBH4; (b) time-dependent UV-vis spectra of the reaction with Ag/SiO2-1; (c) reaction rate plots using Ag/SiO2 with different Ag loadings and different values of C0, where C0 is the initial concentration of 4-NP and C is the concentration of 4-NP at a particular time; catalyst dosage is 50 mg; (d) conversion efficiency of 4-NP for 7 successive reaction cycles. | |
The concentration of NaBH4 was supposed to remain constant during the entire reaction period. Owing to the excess amount of NaBH4 in this reaction, pseudo-first-order kinetics based on 4-NP can be employed to calculate the reaction rate.45 The linear relationship between ln(C0/C) and the reaction time was observed with different Ag loadings (Fig. 8c), which further confirmed the applicability of the pseudo-first-order kinetics. The first-order rate constants of Ag/SiO2-1, Ag/SiO2-2, and Ag/SiO2-3 were calculated to be 0.361 min−1, 0.455 min−1, and 0.69 min−1, respectively. This value of k was relatively higher than those reported in the literature using different supported Ag NPs prepared through different experimental protocols.46 The electron transfer from the BH4− ions to the 4-nitrophenolate ions occurred via the surface of the metal nanoparticles.47 A higher Ag loading meant more Ag-active surfaces, thus favoring the reaction.
Apart from the activity and kinetics, the stability and reusability of the as-prepared Ag catalyst were further examined. It was demonstrated that the catalytic performance of the as-synthesized supported Ag NPs was considerably stable without any obvious decay even after seven successive experimental runs (Fig. 8d). The preeminent catalytic performance and reusability could be due to the quite small and highly dispersed Ag NPs anchored on the silica support. The comparison of the TEM images and XPS spectra before and after seven recycles is shown in ESI (Fig. S4 and S5†). A comparison of the sample before and after the catalytic evaluation was made. It was observed that there was no obvious increase either in the structure or the properties of the reused catalyst. This indicated that the as-synthesized Ag/SiO2 catalyst was stable and did not agglomerate considerably during the catalytic performance evaluation.
Conclusions
In summary, we developed a practical and simple strategy that did not require strong stabilizers (S and P) to synthesize silver colloids. The preparation process was controlled by functionalized siloxane oligomers that played dual roles in encapsulation with both ligating functionalities (–NH) and reducing functionalities (–SiH). This strategy was successfully employed to mediate the variation in silver colloids even those smaller than 2 nm. The variation of the ratios of the functional groups permitted us to define relevant synthesis parameters, for instance, the proximity of silane and amine, the extent of stabilization of the bifunctional ligands and the reaction rates of Ag reduction, which could determine the morphology of the resulting Ag colloids. The Ag colloids obtained by this method were very stable even after being deposited on silica and went through He and O3/O2 calcination to get rid of the organics. Efficient assembly and high-density dispersion of Ag particles ranging from 1.9 to 3.5 nm with narrow size distribution were achieved on the surface of silica. They exhibited a high performance in the catalytic reduction of 4-NP to 4-AP with conversion of 98% within 10 min in the presence of NaBH4. Furthermore, the Ag nanoparticles were quite stable and exhibited excellent reusability for seven successive reaction cycles without obvious decay. Because the principles applied in this synthesis are general, the synthetic route can be applicable to synthesize many other late transition metal colloids with controllable morphology. Therefore, it can provide many promising applications in other fields such as in sensors, catalysis and antibacterial studies.
Conflicts of interest
There are no conflicts to declare in this paper.
Acknowledgements
This work was funded by the State Key Laboratory of Solidification Processing in NWPU (Grant No. SKLSP201816), Xi’an Science and Technology Bureau Innovation Leading Projects (Grant No. 201805037YD15CG21(23)) and China Postdoctoral Science Foundation (Grant No. 2018M643699).
Notes and references
- L. Djerahov, P. Vasileva, I. Karadjova, R. M. Kurakalva and K. K. Aradhi, Carbohydr. Polym., 2016, 147, 45 CrossRef CAS.
- K. Esumi, R. Isono and T. Yoshimura, Langmuir, 2004, 20, 237 CrossRef CAS.
- Z. Wang, E. Beletskiy, S. Lee, X. Hou, Y. Wu, T. Li, M. Kung and H. Kung, J. Mater. Chem. A, 2014, 33, 1743 Search PubMed.
- V. K. Sharma, R. A. Yngard and Y. Lin, Adv. Colloid Interface Sci., 2009, 145, 83 CrossRef CAS.
- K. D. Hermanson, S. O. Lumsdon, J. P. Williams, E. W. Kaler and O. D. Velev, Science, 2001, 294, 1082 CrossRef CAS.
- A. I. Lukman, B. Gong, C. E. Marjo, U. Roessner and A. T. Harris, J. Colloid Interface Sci., 2011, 353, 433 CrossRef CAS.
- T. Maneerung, S. Tokura and R. Rujiravanit, Carbohydr. Polym., 2008, 72, 43 CrossRef CAS.
- M. Maillard, S. Giorgio and M. P. Pileni, J. Phys. Chem. B, 2003, 107, 2466 CrossRef CAS.
- G. Mcnay, D. Eustace, W. E. Smith, K. Faulds and D. Graham, Appl. Spectrosc., 2011, 65, 825 CrossRef CAS.
- W. Shen, Y. Qu, X. Pei, S. Li, S. You, J. Wang, Z. Zhang and J. Zhou, J. Hazard. Mater., 2017, 321, 299 CrossRef CAS PubMed.
- W. Zhang, F. Tan, W. Wang, X. Qiu, X. Qiao and J. Chen, J. Hazard. Mater., 2012, 217–218, 36 CAS.
- C. Zhang, R. Zhang, S. He, L. Li, X. Wang, M. Liu and W. Chen, Chemcatchem, 2017, 9, 980 CrossRef CAS.
- X. Wang, Z. Zhao, D. Ou, B. Tu, D. Cui, X. Wei and M. Cheng, Appl. Surf. Sci., 2016, 385, 445 CrossRef CAS.
- L. Yi, F. You, Y. Yuan, C. Yu, F. Cheng and S. C. Jiang, J. Mater. Chem., 2012, 22, 21173 RSC.
- H. Hu, J. H. Xin, H. Hu, X. Wang, D. Miao and Y. Liu, J. Mater. Chem. A, 2015, 3, 11157 RSC.
- Y. Lei, F. Mehmood, S. Lee, J. Greeley, B. Lee, S. Seifert, R. E. Winans, J. W. Elam, R. J. Meyer and P. C. Redfern, Science, 2010, 328, 224 CrossRef CAS PubMed.
- C. P. Joshi, M. S. Bootharaju, M. J. Alhilaly and O. M. Bakr, J. Am. Chem. Soc., 2015, 137, 11578 CrossRef CAS.
- K. H. Ng, A. H. Liu and R. M. Penner, Langmuir, 2000, 16, 4016 CrossRef CAS.
- L. Lian, S. A. Mitchell, P. A. Hackett and D. M. Rayner, J. Chem. Phys., 1996, 104, 5338 CrossRef CAS.
- Z. Chen, Z. Wen and Q. Jiang, J. Phys. Chem. C, 2017, 121, 3463 CrossRef CAS.
- O. S. Ivanova and F. P. Zamborini, J. Am. Chem. Soc., 2010, 132, 70 CrossRef CAS.
- H. Weller, Angew. Chem., Int.
Ed., 2010, 32, 41 CrossRef.
- L. Dai, W. You, E. Wang, S. Wu, Z. Su, Q. Du, Y. Zhao and Y. Fang, Cryst. Growth Des., 2009, 9, 2110 CrossRef CAS.
- J. A. Schuerman, F. R. Fronczek and J. Selbin, Inorg. Chim. Acta, 1989, 160, 43 CrossRef CAS.
- P. C. Cheng, I. C. Liu, T. N. Hong, J. H. Chen, S. S. Wang, S. L. Wang and J. C. Lin, Polyhedron, 1996, 15, 2733 CrossRef CAS.
- M. S. Bootharaju and T. Pradeep, Investigation into the reactivity of unsupported and supported Ag7 and Ag8 clusters with toxic metal ions, Langmuir, 2011, 27, 8134 CrossRef CAS.
- D. V. Ozkan, S. Aydin, I. Hocaoglu, F. A. Yagci and N. Basaran, Chem.-Biol. Interact., 2018, 291, 212 CrossRef.
- Y. Bhattacharjee, D. Chatterjee and A. Chakraborty, Sens. Actuators, B, 2018, 255, 210 CrossRef CAS.
- H. Imai, A. Hitoshi Nakamura and T. Fukuyo, Cryst. Growth Des., 2005, 5, 1073 CrossRef CAS.
- C. H. Bartholomew, Mechanisms of catalyst deactivation, Appl. Catal., A, 2001, 212, 17 CrossRef CAS.
- S. Kaserer, K. M. Caldwell, D. E. Ramaker and C. Roth, J. Phys. Chem. C, 2013, 117, 6210 CrossRef CAS.
- H. Yoshida, T. Morishita, H. Fukushima, J. Ohshita and A. Kunai, Org. Lett., 2007, 17, 3367 CrossRef.
- G. Shuang, Z. Zhang, K. Liu and B. Dong, Appl. Catal., B, 2016, 188, 245 CrossRef.
-
T. P. Wallace, The scattering of light and other electromagnetic radiation by Milton Kerker, Academic Press, New York, 1969 Search PubMed.
- X. Wei, C. Shao, X. Li, N. Lu, K. Wang, Z. Zhang and Y. Liu, Nanoscale, 2016, 8, 11034 RSC.
- Z. Zhang, J. Huang, Y. Fang, M. Zhang, K. Liu and B. Dong, Adv. Mater., 2017, 29, 1606688 CrossRef.
- D. L. Van Hyning, W. G. Klemperer and C. F. Zukoski, Langmuir, 2001, 17, 3120 CrossRef CAS.
- J. L. Gardea-Torresdey, E. Gomez, J. R. Peralta-Videa, J. G. Parsons, H. Troiani and M. Jose-yacaman, Langmuir, 2003, 19, 1357 CrossRef CAS.
- N. A. Mashayekhi, Y. Yi. Wu, M. C. Kung and H. H. Kung, Chem. Commun., 2012, 48, 10096 RSC.
- M. Tahir, B. Tahir and N. A. S. Amin, Appl. Catal., B, 2017, 204, 548–560 CrossRef CAS.
- K. Pushpavanam, S. Santra and K. Rege, Langmuir, 2014, 30, 14095 CrossRef CAS.
- X. Zhang and Z. Su, Adv. Mater., 2012, 24, 4574 CrossRef CAS.
- M. Zhu, C. Wang, D. Meng and G. Diao, J. Mater. Chem. A, 2013, 1, 2118 RSC.
- J. Zhou, B. Duan, Z. Fang, J. Song, C. Wang, P. B. Messersmith and H. Duan, Adv. Mater., 2014, 26, 701 CrossRef CAS.
- S. Zhao, Y. Gao, J. Li, G. Zhang, R. Sun and C. P. Wong, RSC Adv., 2015, 5, 56974 RSC.
- S. Lu, J. Yu, Y. Cheng, W. Qian, A. Barras, W. Xu, S. Szunerits, D. Cornu and R. Boukherroub, Appl. Surf. Sci., 2017, 411, 163–169 CrossRef CAS.
- G. Gahlawata and A. Choudhury, RSC Adv., 2019, 9, 12944 RSC.
Footnote |
† Electronic supplementary information (ESI) available: NMR spectra of SiHx–NHy–PMHS, XPS spectra and STEM images of Ag/SiO2-1 before and after the reaction. See DOI: 10.1039/c9ra04711e |
|
This journal is © The Royal Society of Chemistry 2019 |
Click here to see how this site uses Cookies. View our privacy policy here.