DOI:
10.1039/C9RA04965G
(Paper)
RSC Adv., 2019,
9, 29141-29148
A bifunctional sensor based on diarylethene for the colorimetric recognition of Cu2+ and fluorescence detection of Cd2+†
Received
2nd July 2019
, Accepted 4th September 2019
First published on 17th September 2019
Abstract
A novel bifunctional sensor based on diarylethene with a benzyl carbazate unit was synthesized successfully. It not only served as a colorimetric sensor for the recognition of Cu2+ by showing changes in absorption spectra and solution color, but also acted as a fluorescent sensor for the detection of Cd2+ through obvious emission intensity enhancement and fluorescence color change. The sensor exhibited excellent selectivity and sensitivity towards Cu2+ and Cd2+, and the limits of detection for Cu2+ and Cd2+ were 8.36 × 10−8 mol L−1 and 1.71 × 10−7 mol L−1, respectively, which were much lower than those reported by the WHO and EPA in drinking water. Furthermore, its application in practical samples demonstrated that the sensor can be effectively applied for the detection of Cu2+ and Cd2+ in practical water samples.
Introduction
Heavy metal contamination is harmful to the environment and human health and is attracting considerable attention nowadays. Among various heavy metals, copper is the third most copious trace element in human bodies and plays a critical role in a series of physiological processes.1–4 However, abnormal levels of Cu2+ are detrimental to human health, not only leading to hematologic manifestations5 and several neurodegenerative diseases, such as Wilson's disease, Menkes disease, Parkinson's disease, and Alzheimer's disease,6–10 but also resulting in gastrointestinal disorders and damage to the liver and kidneys.11,12 According to the regulations of the U.S. Environmental Protection Agency (EPA), the maximum acceptable concentration of copper is nearly 20 μM in drinking water.13 The World Health Organization (WHO) has also stated the maximum allowable level of copper at 31.47 μM in drinking water.14 On the other hand, cadmium, one of the most important heavy metal elements, has been widely used in various agricultural and industrial activities including electroplating, paint pigmentation, nickel–cadmium batteries, and chemical fertilizers,15–17 which has resulted in the widespread distribution of Cd2+ in the environment. Cd2+ is highly toxic in nature even at very low concentrations, and the maximum permissible concentrations of cadmium ions in drinking water are regulated by the WHO and EPA to be 3 μg L−1 and 1.8 μg L−1, respectively.18,19 Furthermore, Cd2+ is not only non-biodegradable, but can also be easily absorbed and accumulated in human bodies and food chains from the environment and retained over a long period of time,20 which causes serious damage to the human nervous system, kidneys, lungs, and many other tissues.21–24 Therefore, it is particularly important to monitor the levels of Cu2+ and Cd2+ in environmental and biological samples.
A number of analytical methods have been utilized for the detection of heavy metal ions, such as atomic absorption spectroscopy (AAS), inductively coupled plasma mass spectrometry (ICP-MS), and electrochemical methods;25–28 however, the expensive instruments, time-consuming operations, in situ analysis, and real-time environmental monitoring are the main drawbacks. Compared to the abovementioned methods, chemosensing as one of the most promising approaches has attracted more attention due to its advantages of a fast response, high sensitivity, high selectivity, and real-time analysis.29,30 Up to now, great efforts have been devoted to develop highly selective and sensitive chemosensors for the detection of heavy metal ions. Although sensors for the colorimetric recognition of Cu2+ (ref. 31−36) and fluorescence detection of Cd2+ have been extensively reported,37–40 some of them display low sensitivity to Cu2+ or Cd2+. On the other hand, there are few reports on chemosensors capable of detecting copper ions by colorimetry and cadmium ions by the fluorescence method.41 Hence, the design and synthesis of a highly sensitive and selective sensor for the colorimetric identification of Cu2+ and fluorescence-based identification of Cd2+ are still huge challenges.
Diarylethene derivatives as sensors for detecting ions have attracted considerable interest owing to their good thermal stability, excellent fatigue resistance, and high reactivity.42–45 In recent years, numerous multifunctional diarylethene-based sensors have been designed and synthesized. In contrast, only a few studies about sensors based on diarylethene for the colorimetric detection of Cu2+ and fluorescence detection of Cd2+ have been reported.41 In this work, a novel sensor for the colorimetric recognition of Cu2+ and fluorescence detection of Cd2+ based on diarylethene with a benzyl carbazate unit was successfully designed and synthesized, as displayed in Scheme 1. Meanwhile, its multifunctional switching characteristics induced by light, Cu2+, and Cd2+ were systematically investigated in THF. The structural characterization data of 1o including 1H NMR, 13C NMR, and ESI-MS are presented in supplementary information (Fig. S1–S3†).
 |
| Scheme 1 The synthetic route and photochromism of 1o. | |
Experimental
General methods
All the chemical reagents used in the experiments were purchased from commercial sources and were not purified further prior to use. The solutions of metal ions (0.1 mol L−1) used in the tests were prepared by dissolving the corresponding metal nitrates in distilled water except for K+, Ba2+, Hg2+ and Sn2+ (their counter ions were chloride ions). The NMR spectra were measured with tetramethylsilane (TMS) as the internal standard on a Bruker AV400 spectrometer. Mass spectra were recorded with a Bruker Amazon SL ion trap mass spectrometer (ESI) using methanol as the solvent. The melting points were determined on a WRS-1B melting point apparatus. The absorption spectra and fluorescence spectra were collected on an Agilent 8454 UV/vis spectrometer and a Hitachi F-4600 fluorescence spectrophotometer, respectively. Moreover, MUA-165 UV lamp and MVL-210 visible lamp were used for photoirradiation. The fluorescence quantum yield was determined on an Absolute PL Quantum Yield Spectrometer QYC11347-11. Infrared spectra (IR) were collected on a BrukerVertex-70 spectrometer. Unless otherwise indicated, all measurements were made at room temperature, and the sample concentration was maintained at 2.0 × 10−5 mol L−1.
Synthesis of the target compound
The target compound 1o was synthesized through a simple one-step reaction, and the synthetic route is shown in Scheme 1. First, compound 2 was synthesized according to a previously reported method.46,47 Second, compound 2 (0.098 g, 0.2 mmol) and compound 3 (0.034 g, 0.2 mmol) were dissolved in absolute ethanol (5.0 mL) in a round-bottom flask (25 mL), followed by the addition of one drop of ethylic acid in the reaction solution. A purple precipitate was observed after the reaction system was continuously stirred for 12 h at room temperature. Then, the resulting purple precipitate was filtered, washed for 3 times with absolute ethanol and dried in vacuum to obtain 1o as a purple solid with a yield of 57% (0.072 g). Mp: 450–452 K. 1H NMR (400 MHz, DMSO-d6, TMS), δ (ppm): 1.83 (s, 3H), 1.92 (s, 3H), 2.38 (s, 3H), 5.18 (s, 2H), 6.82 (s, 1H), 7.42–7.30 (m, 5H), 7.68 (s, 1H), 7.86 (d, 1H, J = 8.0 Hz), 8.04 (s, 1H), 8.09 (d, 1H, J = 8.0 Hz), 8.84 (s, 1H), 11.57 (s, 1H). 13C NMR (100 MHz, DMSO-d6, TMS), δ (ppm): 14.4, 14.5, 15.1, 66.7, 116.3, 120.2, 123.9, 124.8, 124.9, 126.0, 128.5, 128.6, 128.9, 129.1, 130.1, 133.6, 136.9, 138.1, 138.7, 140.3, 142.9, 144.6, 146.2, 152.9, 153.7. IR (KBr, ν, cm−1): 3551, 3484, 3413, 2963, 2923, 2856, 1752, 1603, 1548, 1497, 1444, 1378, 1345, 1262, 1222, 1106, 1053. ESI-MS: m/z = 636.0, [M + H+]+ calcd 636.1.
Results and discussion
Photochromic properties of 1o
The photochromic properties of 1o were investigated upon irradiation with UV/vis in a THF solution (2.0 × 10−5 mol L−1) at room temperature. As shown in Fig. S4,† 1o shows a sharp absorption peak at 336 nm (ε = 4.2 × 104 mol−1 L cm−1), which can be attributed to the π–π* transition.48 Upon irradiation with a 365 nm light, the absorption peak at 336 nm decreased gradually, while a new absorption band centered at 563 nm (ε = 1.1 × 104 mol−1 L cm−1) appeared and increased gradually due to the formation of the closed-ring isomer 1c with increased π–electron delocalization in the molecule.49,50 The color of the solution changed from colorless to purple simultaneously. After irradiating with a 365 nm light for 2 min, the photostationary state (PSS) was reached, and an obvious isosbestic point was observed at 355 nm, indicating that photochromism is a two-component reaction.51–53 Contrarily, upon irradiation with visible light (λ > 500 nm) for 2.0 min, the color of the solution of 1c changed from purple to colorless, indicating that the ring-closed isomer 1c underwent a cycloreversion reaction and returned to the initial ring-open isomer 1o structure.54,55 The quantum yields of the cyclization and cycloreversion reactions of 1o were determined to be 0.30 and 0.03, respectively, with 1,2-bis(2-methyl-5-phenyl-3-thienyl)perfluorocyclopentene as a ref. 56.
Colorimetric sensing of Cu2+ by 1o
The colorimetric properties of 1o towards metal ions including Cu2+, Sn2+, Ca2+, K+, Ag+, Ni2+, Ba2+, Mg2+, Mn2+, Cd2+, Sr2+, Hg2+, Co2+, Cr3+, Fe3+, Pb2+, Zn2+, and Al3+ were investigated in THF. As shown in Fig. 1, upon the addition of 1.0 equiv. of various metal ions, although the absorption at 336 nm shows different changes, only Cu2+ and Ni2+ induced the appearance of new absorption peaks at 413 nm. Meanwhile, the colors of the solutions changed immediately from colorless to yellow and pale yellow with the addition of Cu2+ and Ni2+, respectively. Compared to the color of the solution with Cu2+, the color of the solution with Ni2+ was negligible. Additionally, in order to explore the practical application of 1o in Cu2+ detection, competitive experiments were carried out in the presence of Cu2+ mixed with the other metal ions mentioned above. As shown in Fig. S5,† upon the addition of 1.0 equiv. of Cu2+, the coexistent metal ions, even Ni2+, hardly affect the determination of Cu2+ by 1o in THF. These results suggested that 1o could act as a potential colorimetric sensor of Cu2+ in practical samples.
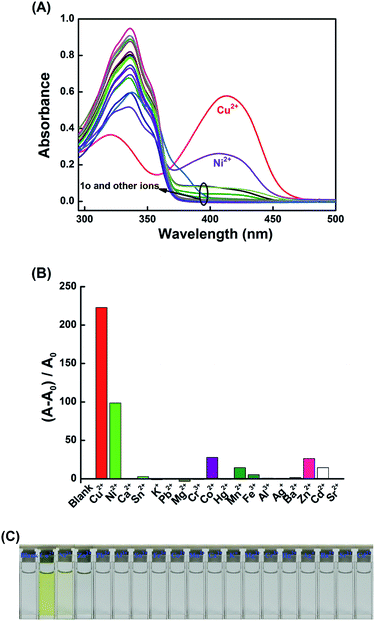 |
| Fig. 1 Changes in the absorption of 1o (2.0 × 10−5 mol L−1) induced by various metal ions (1.0 equiv.) in THF: (A) UV-vis absorption spectral changes; (B) absorbance at 413 nm in the presence of different metal ions; (C) photo demonstrating the colors of the 1o solutions containing different metal ions. | |
UV-vis titration experiments were performed to investigate the binding mode between 1o and Cu2+. As depicted in Fig. 2A, upon the gradual addition of Cu2+ to the solution of 1o, the absorption at 336 nm decreases and a blue shift is observed from 336 nm to 320 nm. At the same time, a new absorption band centered at 413 nm emerged and increased gradually, which might be ascribed to the metal-to-ligand charge transfer (MLCT),57 resulting in a color change of the solution from colorless to yellow. After 0.6 equiv. of Cu2+ was added, the absorbance intensity at 413 nm reached saturation and remained unchanged with further titration (Fig. S6†). An isosbestic point was observed at 365 nm, indicating that only one stable complex 1o-Cu2+ (1o′) was formed between 1o and Cu2+. However, the absorption spectrum of 1o could not be recovered when excessive EDTA was added.
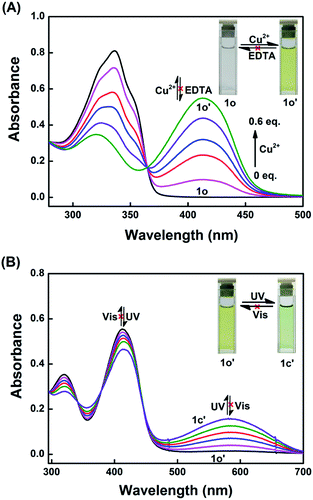 |
| Fig. 2 Absorption spectra and color changes of 1o (A) in THF (2.0 × 10−5 mol L−1) with the addition of Cu2+ ion, and (B) 1o′ upon irradiation with UV/vis lights. | |
The photochromism of 1o′ was also investigated by alternating the irradiation between UV and visible lights. As shown in Fig. 2B, upon irradiation with 365 nm light, the absorptions at 320 nm and 413 nm decreased gradually, and two new absorption bands at 356 nm and 584 nm emerged and increased simultaneously. In accordance with the change in the absorption spectrum, the color of the solution changed from yellow to olive due to the formation of the closed-ring isomer 1c-Cu2+ complex (1c′). After irradiation for 3.0 minutes, PPS was achieved, and three isosbestic points were observed at 342 nm, 379 nm, and 442 nm. However, the absorption spectrum of 1o′ could not be completely recovered upon irradiation with visible light (λ > 500 nm). When Cu2+ was gradually added to the solution of 1c, the absorption band centered at 340 nm decreased distinctly, and a new absorption band centered at 420 nm appeared and increased. At the same time, the absorption peak at 563 nm increased slightly and red-shifted to 584 nm, accompanied by the color change of the solution from purple to olive (Fig. S7†). A well-defined isosbestic point was observed at 376 nm, revealing the formation of the complex 1c′. When excess EDTA was added, the absorption spectrum could not recover to that of 1c.
Fluorescence response of 1o to Cd2+
The fluorescence responses of 1o to various metal ions including Cu2+, Sn2+, Ca2+, K+, Ag+, Ni2+, Ba2+, Mg2+, Mn2+, Cd2+, Sr2+, Hg2+, Co2+, Cr3+, Fe3+, Pb2+, Zn2+, and Al3+ were also investigated in THF. As shown in Fig. 3A, 1o displays very weak fluorescence with 340 nm light excitation, and the quantum yield is determined to be 0.002. Upon the addition of 4.0 equiv. of metal ions, only Cd2+ induced prominent fluorescence enhancement at 470 nm, while the other metal ions did not show any significant effects (Fig. 3B). It was noteworthy that Zn2+ could also cause fluorescence enhancement, but the enhancement could be ignored compared to that of Cd2+. Under the 365 nm light, the changes in the fluorescence color of 1o induced by various metal ions were recorded, and only Cd2+ induced an obvious color change that could be seen by the naked eyes (Fig. 3C). These results indicated that 1o can act as a fluorescent sensor for the detection of Cd2+. Competitive experiments were also carried out by adding 4.0 equiv. of Cd2+ to the solutions containing 1o and various metal ions (4.0 equiv. of 1o). As shown in Fig. S8,† the coexistence of ions hardly interferes with the detection of cadmium ions except for Ni2+ and Sn2+. It is noteworthy that Cu2+ could significantly quench the fluorescence of 1o-Cd2+, which may be due to its paramagnetism.58
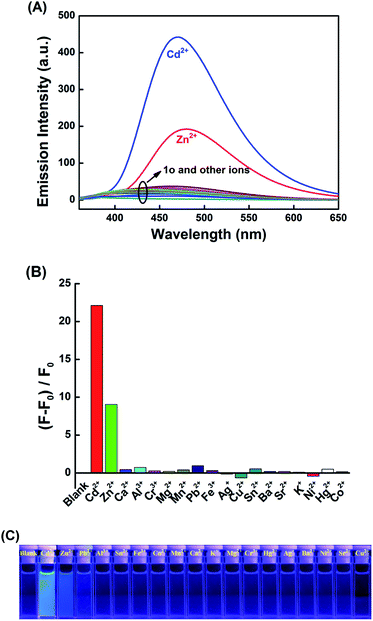 |
| Fig. 3 Changes in the fluorescence of 1o (2.0 × 10−5 mol L−1) induced by various metal ions (4.0 equiv.) in THF: (A) fluorescence emission spectral changes (λex = 340 nm); (B) emission intensity changes; (C) color changes (under 365 nm light). | |
The fluorescence titration of 1o with Cd2+ was performed in THF at room temperature. As shown in Fig. 4A, upon the gradual addition of 0 to 4.0 equiv. Cd2+ to the solution of 1o, the emission intensity of 1o increased gradually and reached saturation (Fig. S9†). At the end of titration, the emission intensity enhanced 22-fold, with the emission peak shifting from 430 nm to 470 nm, which was ascribed to the formation of a new complex 1o-Cd2+ (1o′′). With further titration, the fluorescence intensity remained unchanged. The fluorescence color changed from dark to bright blue, and the fluorescence quantum yield of 1o′′ was determined to be 0.01. The weak fluorescence of 1o was attributed to the isomerization of the C
N bond.39,59 After the addition of Cd2+, a stable complex 1o′′ was formed, which inhibited the isomerization of the C
N bond and increased the rigidity of the molecule, resulting in a chelation enhanced fluorescence (CHEF) effect.60,61 The fluorescence spectrum of 1o could be recovered by adding 60 equiv. of EDTA, indicating that the complexation/decomplexation between 1o and Cd2+ was reversible.
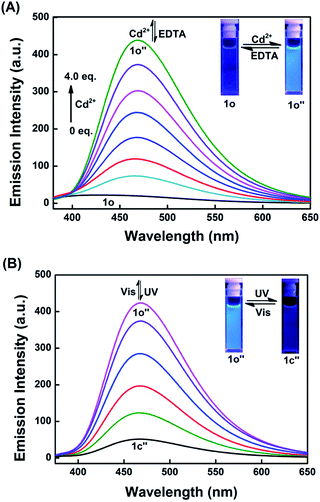 |
| Fig. 4 Fluorescence spectral changes and color responses of (A) 1o in THF (2.0 × 10−5 mol L−1) induced by Cd2+ (0–4.0 equiv.) and (B) 1o′′upon irradiation with UV and visible lights (λex = 340 nm). | |
The 1o′′ complex also exhibited excellent fluorescence switching properties. As shown in Fig. 4B, upon irradiation with 365 nm light, the emission intensity of 1o′′ at 470 nm is quenched by 88% at PPS, indicating that 1o′′ exhibits relatively high fluorescence modulation efficiency. The decrease in the emission intensity was attributed to the formation of the closed-ring isomer 1c-Cd2+ complex (1o′′),62 and the fluorescence resonant energy transfer (FRET) process occurred.63,64 The fluorescence spectrum of 1o′′ could be recovered upon irradiation with visible light (λ > 500 nm). The fluorescence titration experiments of 1c with Cd2+ were also carried out in THF. Upon the addition of 4.0 equiv. of Cd2+ to the solution of 1c, the fluorescence intensity at 470 nm increased by 4-fold due to the formation of 1o′′ (Fig. S10†). Similarly, the fluorescence spectrum of 1c could be recovered by adding 60 equiv. of EDTA, indicating that the complexation between 1c and Cd2+ was also reversible.
Complexation mechanism of 1o with Cu2+ and Cd2+
The stoichiometry between 1o and Cu2+/Cd2+ was determined by Job's plot analysis.65 As shown in Fig. 5A, the maximum absorbance appears at the molar ratio of 0.5, indicating that the binding mode between 1o and Cu2+ is 1
:
1. The mass spectrum peak at m/z = 820.8 corresponding to [1o + Cu2+ + 2NO3− − H+]− (calcd: 821.0) further confirmed the 1
:
1 stoichiometry (Fig. S11†). On the basis of the 1
:
1 binding mode between 1o and Cu2+, the association constant (Ka) was calculated to be 1.25 × 104 L mol−1 (R = 0.994) (Fig. 5B), and the limit of detection (LOD) of 1o towards Cu2+ was calculated to be 8.36 × 10−8 mol L−1 based on 3δ/s (ref. 66 and 67) (Fig. S12†). Similarly, the Job's plot also indicated the formation of a 1
:
1 complex between 1o and Cd2+(Fig. 6A). Additionally, ESI-MS was performed for further verifying the binding stoichiometry; a signal peak at m/z = 871.7 was assigned to [1o + Cd2+ + 2NO3− − H+]− (calcd: 871.9) (Fig. S13†), which was consistent with the stoichiometry of 1
:
1. Based on the 1
:
1 binding mode, the association constant (Ka) was calculated to be 1.28 × 103 L mol−1 (R = 0.991) (Fig. 6B). The detection limit of 1o towards Cd2+ was determined to be 1.71 × 10−7 mol L−1 (Fig. S14†). The detection limit of 1o was much lower than those of the reported sensors for Cu2+ or Cd2+ (Table S1†).32,41,68−74 The results displayed that 1o not only acted as a colorimetric sensor for Cu2+, but also served as a fluorescent sensor for Cd2+ with high sensitivity.
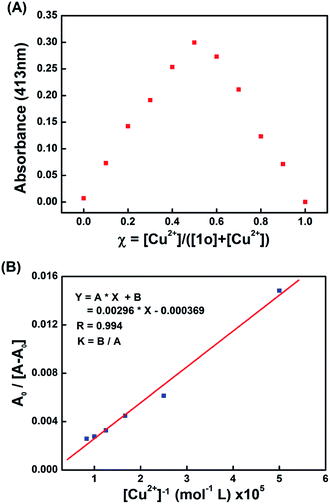 |
| Fig. 5 (A) Job's plot showing the 1 : 1 complex of 1o and Cu2+, and (B) Benesi–Hildebrand plot based on the 1 : 1 binding stoichiometry. The binding constant of 1o with Cu2+ was calculated to be 1.25 × 104 L mol−1. | |
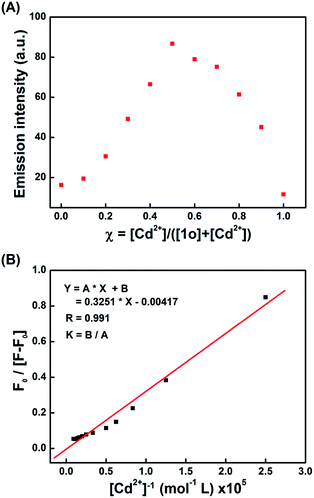 |
| Fig. 6 Job's plot showing the 1 : 1 complex of 1o and Cd2+ (A) and (B) Benesi–Hildebrand plot based on the 1 : 1 binding stoichiometry; the binding constant of 1o with Cd2+ was calculated to be 1.28 × 103 L mol−1. | |
1H NMR titration and IR spectral results were recorded to further explore the interaction between 1o and Cu2+/Cd2+. First, the binding mode between 1o and Cu2+ was investigated, and the 1H NMR titration did not provide useful information due to the paramagnetic nature of Cu2+.57 In the IR spectrum of 1o, the stretching vibration absorption peaks at 1603, 1548, 1497, and 1444 cm−1 are assigned to the pyridine unit; the absorption peaks at 1752 and 1617 cm−1 are assigned to the stretching vibrations of C
O and C
N, respectively (Fig. S15†). When Cu2+ was added, the stretching vibration peaks of the pyridine unit remained almost unaffected, indicating that the N atom of the pyridine unit did not participate in the coordination process. It could be clearly seen that the absorption peaks at 1617 and 1752 cm−1 shifted severally to 1657 and 1725 cm−1, suggesting that the N atom of the Schiff base unit and the O atom in C
O are more likely the binding sites for Cu2+ coordination. Then, the interacting mode between 1o and Cd2+ was further investigated. As depicted in Fig. 7, with the addition of Cd2+, the signals (Hb, Hc, Hd) assigned to the pyridine ring and Schiff base displayed downfield shifts from 8.77, 8.02 and 7.97 ppm to 8.85, 8.27 and 8.17 ppm, respectively. However, the signal (He) at 7.97 ppm exhibited an upfield shift to 7.77 ppm. These shifts may be attributed to the coordinate bond between Cd2+ and the N atom on the pyridine unit or the Schiff base unit. Notably, the signal at 5.20 ppm ascribed to methylene displayed a 0.13 ppm downfield shift to 5.36 ppm, and the proton signal at 10.45 ppm (Ha) assigned to –NH had a large downfield shift to 12.14 ppm, which may be caused by the isomerization of the amide unit (NH–C
O) and the formation of a new N
C–OH structure. The coordination of the O atom with Cd2+ led to the decrease in the electron cloud density of the O atom and resulted in a large downfield shift of the proton signal (Ha).75 In the IR spectra, upon the addition of Cd2+, the stretching vibration absorption peaks of the pyridine unit changed slightly, while it could be seen that the absorption peak of C
N at 1617 cm−1 shifted to 1649 cm−1. The results further confirmed that the N atoms of the Schiff base unit were involved in coordination with Cd2+. It was worth noting that the peak at 1752 cm−1 assigned to C
O disappeared, and a new absorption peak appeared at 1694 cm−1, indicating that the isomerization of the amide unit (NH–C
O) occurred and resulted in the formation of a new N
C–OH structure. The results further confirmed that the N atom of the Schiff base unit and the O atom of the N
C–OH unit participated in coordination. The appearance of absorption peaks at 2397 and 2428 cm−1 was attributed to the formation of the large conjugated system (C
N–N
C) (Fig. S15†). Therefore, in the 1H NMR titration, the changes in signals (Ha, Hf) were ascribed to the isomerization of amides. Based on these results, a reasonable binding mode between 1o and Cu2+/Cd2+ is exhibited in Scheme 2, and the optimized structures of 1o-Cu2+ and 1o-Cd2+ are shown in Fig. S16.†
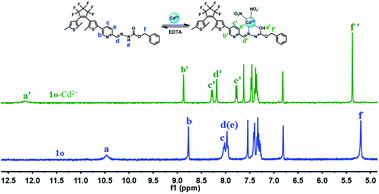 |
| Fig. 7 1H NMR spectra of 1o and 1o-Cd2+ in THF-d8 (inset shows the proposed binding mode of the complex 1o′′). | |
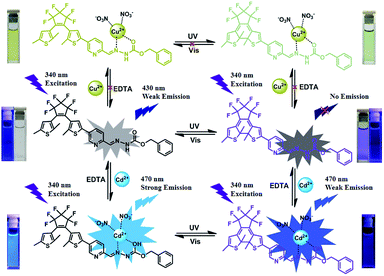 |
| Scheme 2 Changes in the photochromism, color and absorption of 1o induced by Cu2+/EDTA and UV-vis lights, and the multi-controlled fluorescence-switching behaviors of 1o induced by UV/vis lights and Cd2+/EDTA. | |
Application in real water samples
To further explore the potential application of the sensor 1o in actual water samples, real samples including those obtained from the Ganjiang River and tap water were collected from Nanchang City and analyzed according to the methods reported previously.76,77 For better results and analysis of Cu2+ and Cd2+ in the water samples, the collected water samples were pretreated with a 0.2 μm membrane to remove suspended substances; then, different amounts of Cu2+ and Cd2+ were spiked separately into the real water samples to evaluate their recovery. The recoveries of Cu2+ and Cd2+ added in the real samples were calculated, and the results are summed up in Tables 1 and 2, respectively. It could be seen that the recoveries for Cu2+ ranged from 98.0% to 104.7%, while those for Cd2+ ranged between 97.2% and 103.9%, revealing the high accuracy of the sensor 1o and its potential applications for the determination of Cu2+ and Cd2+ in practical water samples.
Table 1 Application in practical samples for the detection of Cu2+
Sample |
Cu2+ added (μmol L−1) |
Cu2+ determined (μmol L−1) |
Recovery (%) |
Ganjiang River |
4 |
4.10 |
102.5 |
6 |
6.28 |
104.7 |
8 |
7.96 |
99.5 |
10 |
10.35 |
103.5 |
Tap water |
4 |
3.92 |
98.0 |
6 |
6.08 |
101.3 |
8 |
7.89 |
98.6 |
10 |
10.08 |
100.8 |
Table 2 Application in practical samples for the detection of Cd2+
Sample |
Cd2+ added (μmol L−1) |
Cd2+ determined (μmol L−1) |
Recovery (%) |
Ganjiang River |
4 |
4.08 |
102.0 |
8 |
7.77 |
97.2 |
12 |
11.80 |
98.3 |
16 |
16.07 |
100.4 |
Tap water |
4 |
4.06 |
101.5 |
8 |
8.31 |
103.9 |
12 |
12.34 |
102.8 |
16 |
15.91 |
99.4 |
Conclusions
In summary, a novel bifunctional sensor for the colorimetric detection of Cu2+ and fluorescence detection of Cd2+ based on diarylethene with a benzyl carbazate unit was synthesized successfully. The detection limits for Cu2+ and Cd2+ were 8.36 × 10−8 mol L−1 and 1.71 × 10−7 mol L−1, respectively, which were much lower than the values allowed by the WHO and EPA in drinking water. The results from the applications in real samples indicated that the sensor could be used to detect Cu2+ and Cd2+ in practical samples with high accuracy. The results will provide us a new thought for the design and synthesis of multi-functional sensors.
Conflicts of interest
There are no conflicts of interest to declare.
Acknowledgements
The authors are grateful for the financial support from the National Natural Science Foundation of China (41867053), the “5511” Science and Technology Innovation Talent project of Jiangxi (2016BCB18015), the key project of Natural Science Foundation of Jiangxi Province (20171ACB20025), the Young Talents Project of Jiangxi Science and Technology Normal University (2015QNBJRC004), and the Master Graduate Innovation Special Foundation of Jiangxi Province (YC2018-S407).
Notes and references
- P. Milindanuth and P. Pisitsak, Mater. Chem. Phys., 2018, 216, 325–331 CrossRef CAS.
- R. Martínez, A. Espinosa, A. Tárraga and P. Molina, Tetrahedron, 2008, 64, 2184–2191 CrossRef.
- Y. Guo, Z. Wang, W. Qu, H. Shao and X. Jiang, Biosens. Bioelectron., 2011, 26, 4064–4069 CrossRef CAS PubMed.
- J. Fan, P. Zhan, M. Hu, W. Sun, J. Tang, J. Wang, S. Sun, F. Song and X. Peng, Org. Lett., 2013, 15, 492–495 CrossRef CAS PubMed.
- T. R. Halfdanarson, N. Kumar, C. Y. Li, R. L. Phyliky and W. J. Hogan, Eur. J. Haematol., 2008, 80, 523–531 CrossRef CAS PubMed.
- Y. Zhou, J. Zhang, H. Zhou, Q. Zhang, T. Ma and J. Niu, J. Lumin., 2012, 132, 1837–1841 CrossRef CAS.
- L. M. Gaetke and C. K. Chow, Toxicology, 2003, 189, 147–163 CrossRef CAS PubMed.
- J. S. Bains and C. A. Shaw, Brain Res. Rev., 1997, 25, 335–358 CrossRef CAS PubMed.
- G. J. Brewer, Am. J. Clin. Nutr., 1998, 67, 1087S–1090S CrossRef CAS PubMed.
- D. G. Barceloux, J. Toxicol., Clin. Toxicol., 1999, 37, 217–230 CrossRef CAS PubMed.
- N. Kumar, Mayo Clin. Proc., 2006, 81, 1371–1384 CrossRef CAS PubMed.
- B. Sarkar, Metal Ions in Biological Systems, ed. H. Sigel, Marcel Dekker, New York, 1981, vol. 12, pp. 233–281 Search PubMed.
- J. Liu and Y. Lu, J. Am. Chem. Soc., 2007, 129, 9838–9839 CrossRef CAS PubMed.
- G. R. You, G. J. Park, J. J. Lee and C. Kim, Dalton Trans., 2015, 44, 9120–9129 RSC.
- F. Beck and P. Rüetschi, Electrochim. Acta, 2000, 45, 2467–2482 CrossRef CAS.
- C. L. Han, L. H. Wu, W. N. Tan, D. X. Zhong, Y. J. Huang, Y. M. Luo and P. Christie, Environ. Geochem. Health, 2012, 34, 481–492 CrossRef CAS PubMed.
- R. L. Chaney, J. A. Ryan, Y. M. Li and S. L. Brown, Soil Cadmium as a Threat to Human Health, Cadmium in Soils and Plants, Springer Netherlands, 1999, pp. 219–256 Search PubMed.
- World Health Organization (WHO), Guidelines for Drinking-Water Quality, World Health Organization, Geneva, 4th edn, 2011, p. 327 Search PubMed.
- USA Environmental Protection Agency, Aquatic Life, Ambient Water QualityCriteria, Cadmium-2016, EPA 820-R-16-002, Office of Water, Office of Science and Technology, Health and Ecological Criteria Division, Washington D.C., USA, 2016 March Search PubMed.
- Y. Li, T. Xia, J. Zhang, Y. Cui, B. Li, Y. Yang and G. Qian, J. Solid State Chem., 2019, 275, 38–42 CrossRef CAS.
- A. Åkesson, B. Julin and A. Wolk, Cancer Res., 2008, 68, 6435–6441 CrossRef PubMed.
- J. A. McElroy, M. M. Shafer, A. T. Dietz, J. M. Hampton and P. A. Newcomb, J. Natl. Cancer Inst., 2006, 98, 869–873 CrossRef CAS PubMed.
- M. P. Waalkes, J. Inorg. Biochem., 2000, 79, 241–244 CrossRef CAS PubMed.
- M. Waisberg, P. Joseph, B. Hale and D. Beyersmann, Toxicology, 2003, 192, 95–117 CrossRef CAS PubMed.
- S. M. Z. Hossain and J. D. Brennan, Anal. Chem., 2011, 83, 8772–8778 CrossRef CAS PubMed.
- A. Lafaye, C. Junot, B. R. L. Gall, P. Fritsch, J. C. Tabet and E. Ezan, Rapid Commun. Mass Spectrom., 2003, 17, 2541–2549 CrossRef CAS PubMed.
- C. Liu, S. He, K. Shen, X. Feng, G. Fang and S. Wang, Food Anal. Methods, 2015, 8, 1785–1793 CrossRef.
- N. Li, D. Zhang, Q. Zhang, Y. Lu, J. Jiang, G. L. Liu and Q. Liu, Sens. Actuators, B, 2016, 231, 349–356 CrossRef CAS.
- R. V. Rathod, S. Bera, M. Singh and D. Mondal, RSC Adv., 2016, 6, 34608–34615 RSC.
- L. Yan, R. Li, F. Ma and Z. Qi, Anal. Methods, 2017, 9, 1119–1124 RSC.
- N. Chakraborty, A. Chakraborty and S. Das, J. Lumin., 2018, 199, 302–309 CrossRef CAS.
- Q. Huang, Y. T. Chen, Y. W. Ren, Z. Y. Wang, Y. X. Zhu and Y. Zhang, Anal. Methods, 2018, 10, 5731–5737 RSC.
- P. Sengupta, A. Ganguly and A. Bose, Spectrochim. Acta, Part A, 2018, 198, 204–211 CrossRef CAS PubMed.
- L. Wang, Q. Bing, J. Li and G. Wang, J. Photochem. Photobiol., A, 2018, 360, 86–94 CrossRef CAS.
- L. Zhang, R. Wang, R. Liu, X. Du, R. Meng, L. Liu and J. Yao, Cellulose, 2018, 25, 6947–6961 CrossRef CAS.
- S. Guo, G. Liu, C. Fan and S. Pu, Sens. Actuators, B, 2018, 266, 603–613 CrossRef CAS.
- Y. Pan, J. Wang, X. Guo, X. Liu, X. Tang and H. Zhang, J. Colloid Interface Sci., 2018, 513, 418–426 CrossRef CAS PubMed.
- Z. Wang, S. Cui, S. Qiu and S. Pu, Tetrahedron, 2018, 74, 7431–7437 CrossRef CAS.
- X. Zhang, R. Wang, C. Fan, G. Liu and S. Pu, Dyes Pigm., 2017, 139, 208–217 CrossRef CAS.
- Z. Wang, S. Cui, S. Qiu and S. Pu, J. Photochem. Photobiol., A, 2018, 367, 212–218 CrossRef CAS.
- H. L. Liu, S. Q. Cui, F. Shi and S. Z. Pu, Dyes Pigm., 2019, 161, 34–43 CrossRef CAS.
- S. Kawai, T. Nakashima, K. Atsumi, T. Sakai, M. Harigai, Y. Imamoto and T. Kawai, Chem. Mater., 2007, 19, 3479–3483 CrossRef CAS.
- X. Li and H. Tian, Tetrahedron Lett., 2005, 46, 5409–5412 CrossRef CAS.
- H. Samachetty and N. Branda, Pure Appl. Chem., 2006, 78, 2351–2359 CAS.
- Q. Luo, H. Cheng and H. Tian, Polym. Chem., 2011, 2, 2435–2443 RSC.
- P. Ren, R. Wang, S. Pu, G. Liu and C. Fan, J. Phys. Org. Chem., 2014, 27, 183–190 CrossRef CAS.
- W. D. Gao, H. Li and S. Z. Pu, J. Photochem. Photobiol., A, 2018, 364, 208–218 CrossRef CAS.
- S. Z. Pu, G. Liu, L. Shen and J. K. Xu, Org. Lett., 2007, 9, 2139–2142 CrossRef CAS PubMed.
- G. Liu, S. Z. Pu and X. M. Wang, J. Photochem. Photobiol., A, 2010, 214, 230–240 CrossRef CAS.
- T. Nakashima, K. Miyamura, T. Sakai and T. Kawai, Chem. - Eur. J., 2009, 15, 1977–1984 CrossRef CAS PubMed.
- S. Q. Cui, S. Z. Pu and G. Liu, Spectrochim. Acta, Part A, 2014, 132, 339–344 CrossRef CAS PubMed.
- H. C. Ding, G. Liu and S. Z. Pu, Dyes Pigm., 2014, 103, 82–88 CrossRef CAS.
- H. Zhang, X. X. Kou, Q. Zhang, D. H. Qu and H. Tian, Org. Biomol. Chem., 2011, 9, 4051–4056 RSC.
- F. Hu, M. Cao, X. Ma, S. H. Liu and J. Yin, J. Org. Chem., 2015, 80, 7830–7835 CrossRef CAS PubMed.
- Z. Li, Y. Wang, M. Li, H. Zhang, H. Guo, H. Ya and J. Yin, Org. Biomol. Chem., 2018, 16, 6988–6997 RSC.
- M. Irie, T. Lifka, S. Kobatake and N. Kato, J. Am. Chem. Soc., 2000, 122, 4871–4876 CrossRef CAS.
- S. Q. Cui, S. Z. Pu and Y. F. Dai, Anal. Methods, 2015, 7, 3593–3599 RSC.
- R. J. Wang, L. Diao, Q. Ren, G. Liu and S. Z. Pu, ACS Omega, 2019, 4, 309–319 CrossRef CAS.
- W. K. Dong, X. L. Li, L. Wang, Y. Zhang and Y. J. Ding, Sens. Actuators, B, 2016, 229, 370–378 CrossRef CAS.
- S. Patil, R. Patil, U. Fegade, B. Bondhopadhyay, U. Pete, S. K. Sahoo, N. Singh, A. Basu, R. Bendre and A. Kuwar, Photochem. Photobiol. Sci., 2015, 14, 439–443 RSC.
- S. Patil, U. Fegade, S. K. Sahoo, A. Singh, J. Marek, N. Singh, R. Bendre and A. Kuwar, ChemPhysChem, 2014, 15, 2230–2235 CrossRef CAS PubMed.
- Y. M. Xue, R. J. Wang and C. H. Zheng, Tetrahedron Lett., 2016, 57, 1877–1881 CrossRef CAS.
- S. Z. Pu, L. L. Ma, G. Liu, H. C. Ding and B. Chen, Dyes Pigm., 2015, 113, 70–77 CrossRef CAS.
- M. Bossi, V. Belov, S. Polyakova and S. W. Hell, Angew. Chem., Int. Ed., 2006, 45, 7462–7465 CrossRef CAS PubMed.
- P. Job, Ann. Chim., 1928, 9, 113–203 CAS.
- M. Shortreed, R. Kopelman, M. Kuhn and B. Hoyland, Anal. Chem., 1996, 68, 1414–1418 CrossRef CAS PubMed.
- W. Lin, L. Yuan, Z. Cao, Y. Feng and L. Long, Chem. - Eur. J., 2009, 15, 5096–5103 CrossRef CAS PubMed.
- C. H. Min, S. Na, J. E. Shin, J. K. Kim, T. G. Jo and C. Kim, New J. Chem., 2017, 41, 3991–3999 RSC.
- G. R. You, H. J. Jang, T. G. Jo and C. Kim, RSC Adv., 2016, 6, 74400–74408 RSC.
- G. R. You, G. J. Park, J. J. Lee and C. Kim, Dalton Trans., 2015, 44, 9120–9129 RSC.
- X. J. Jiang, M. Li, H. L. Lu, L. H. Xu, H. Xu and S. Q. Zang, Inorg. Chem., 2014, 53, 12665–12667 CrossRef CAS PubMed.
- G. Li, D. Zhang, G. Liu and S. Z. Pu, Tetrahedron Lett., 2016, 57, 5205–5210 CrossRef CAS.
- Z. Xu, G. Li, Y. Y. Ren, H. Huang, X. Wen, Q. Xu and L. Xu, Dalton Trans., 2016, 45, 12087–12093 RSC.
- R. Khani, E. Ghiamati, R. Boroujerdi, A. Rezaeifard and M. H. Zaryabi, Spectrochim. Acta, Part A, 2016, 163, 120–126 CrossRef CAS PubMed.
- A. Dhara, N. Guchhait, I. Mukherjee, A. Mukherjee and S. C. Bhattacharya, RSC Adv., 2016, 6, 105930–105939 RSC.
- Y. T. Chen, Y. S. Mi and Q. F. Xie, Anal. Methods, 2013, 5, 4818–4823 RSC.
- X. Wu, J. Chen and J. X. Zhao, Analyst, 2013, 138, 5281–5287 RSC.
Footnote |
† Electronic supplementary information (ESI) available. See DOI: 10.1039/c9ra04965g |
|
This journal is © The Royal Society of Chemistry 2019 |
Click here to see how this site uses Cookies. View our privacy policy here.