DOI:
10.1039/C8SC04464C
(Edge Article)
Chem. Sci., 2019,
10, 1193-1200
Rationally designed pure-inorganic upconversion nanoprobes for ultra-highly selective hydrogen sulfide imaging and elimination in vivo†
Received
8th October 2018
, Accepted 12th November 2018
First published on 12th November 2018
Abstract
Lung injury is a hydrogen sulfide (H2S)-associated complication with high mortality in acute pancreatitis (AP) cases. Herein, we used Prussian Blue (PB) as a H2S-responsive acceptor to develop a novel pure-inorganic upconversion nanoprobe for detecting and eliminating H2S, which can be used for diagnosing AP and alleviating lung injury. Upconversion nanoprobes with 5 nm PB shells were optimized to achieve outstanding in vitro H2S detection capacity (linear range: 0–150 μM, LOD: 50 nM), which met the in vivo serum H2S range, and thus were feasible for imaging H2S in vivo. More importantly, when combined with the traditional H2S synthetase inhibitor DL-PAG, the nanoprobes also served as a therapeutic agent that synergistically alleviated lung injury. As PB is an FDA-approved drug, our work proposes a potential clinical modality for the early diagnosis of AP, which will decrease lung injury-induced mortality and increase the survival rates of AP cases.
Introduction
Hydrogen sulfide (H2S) is a newly discovered gaseous signaling molecule, in addition to nitrogen oxide and carbon monoxide, and is an essential player in various physiological processes.1 In acute pancreatitis (AP) cases, excessive serum H2S levels can cause lung injury, which leads to high early mortality rates due to respiratory failure and hypoxemia.2,3 Therefore, developing a probe that can simultaneously image and eliminate H2S from serum would significantly benefit AP patients.
Luminescence probes can be applied to disease diagnostics due to their strong performance in time-resolution imaging and dynamic bioinformatics monitoring.4–11 Among all phosphors, upconversion nanoparticles emit adjustable luminescence (300–800 nm) under near-infrared (NIR) excitation, and have the advantages of low background luminescence and high stability, which makes them ideal for in vivo luminescence imaging.12–16 Therefore, upconversion nanoparticles are feasible for use as donors in construction of high-performance luminescence nanoprobes.17–21 Using upconversion nanoparticles as donors and H2S-responsive acceptors, some upconversion nanoprobes for H2S detection and imaging have been developed based on Förster resonance energy transfer (FRET) and inner filter effects.22–24 However, the typical H2S-responsive acceptors were organic dyes with obvious shortcomings, including low stability and hydrophobicity. In contrast, inorganic acceptors have no such issues, but are limited by low H2S selectivity and potential toxicity to biosystems, which resulted in less research into inorganic acceptors. Thus, an exploration of biocompatible inorganic H2S-responsive acceptors with good selectivity and high stability should be conducted with the aim of developing nontoxic hydrophilic H2S nanoprobes.
Due to the strong interaction between Fe(II/III) and cyanide, Prussian Blue (PB) is ultra-stable when combined with various biomolecules, which contributes to its outstanding biocompatibility.25 Nevertheless, a synchronous reaction between Fe(III) and H2S may cause the decomposition of PB in a H2S solution, which would result in decreased PB absorbance that can be used to colorimetrically detect H2S. When applying PB as a H2S-responsive acceptor to construct upconversion nanoprobes, the weakened absorbance in the visible and NIR ranges can also lead to upconversion luminescence (UCL) recovery, which can be used for luminescence detection of H2S. Conversely, PB can also eliminate free H2S in fluid by forming stable sulfur and sulfide compounds, which means that these nanoprobes could be a potential drug for eliminating excessive serum H2S. As PB is a US Food and Drug Administration (FDA)-approved drug for clinical use, PB-modified upconversion nanoprobes may be optimal inorganic nanoprobes that simultaneously image and eliminate H2S.
Herein, a novel PB-functionalized upconversion nanoprobe was developed for dual-modal colorimetric/luminescence H2S detection. The thickness of the PB shell was modulated to achieve the optimal detection capacity within the serum H2S concentration range. To ensure safety, the cytotoxicity, biocompatibility, and biobehavior of the as-optimized nanoprobes were carefully studied. Ultimately, the feasibility of the nanoprobes for imaging H2S and alleviating lung injury was confirmed in an AP mouse model.
Results and discussion
Rationally designed upconversion nanoprobes for H2S detection
The PB-functionalized upconversion nanoprobes were rationally designed as a core–shell structure of 20% Er-doped NaLuF4:Yb,Er,Tm@NaLuF4 nanoparticles (UCNPs) coated with a homogeneous PB shell of optimal thickness. Using typical solvothermal methods, uniform core–shell UCNPs were synthesized with a larger size distribution (47.2 ± 4.3 nm) than that of NaLuF4:Yb,Er,Tm cores (37.2 ± 3.8 nm; Fig. 1A and S1A and B†). After inner shell coating, the upconversion luminescence (UCL) of UCNPs was significantly improved in both visible and NIR regions due to inhibition of multi-phonon relaxation between the emitter and the absorbed ligands (Fig. S1C†). Furthermore, X-ray diffraction (XRD) patterns suggested that the UCNPs were in a highly crystallized hexagonal phase, corresponding to the standard reference card of β-NaLuF4 (JCPDS: 027-0726; Fig. S2A†). High-resolution transmission electron microscopy (HR-TEM) images of UCNPs with a defined crystallographic plane supported this finding (Fig. 1B).
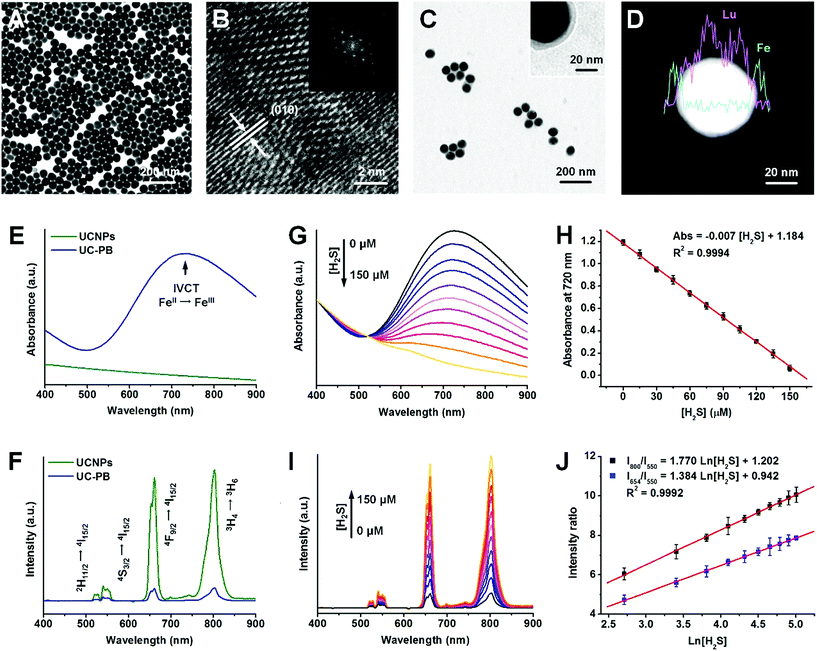 |
| Fig. 1 Characterization and H2S detection capacities of the UC-PB nanoprobe. TEM image (A) and high-resolution lattice image (B) of UCNPs. Inset: the corresponding fast Fourier transform diffractogram. (C) TEM image of the UC-PB nanoprobes. Inset: TEM image of a single UC-PB nanoprobe. (D) STEM image and EDXA line scan on a single UC-PB nanoprobe. UV-vis-NIR absorbance spectra (E) and UCL spectra (F) of UCNPs and UC-PB nanoprobes. UV-vis-NIR absorbance spectra (G) and UCL spectra (I) of UC-PB nanoprobes in response to various concentrations of H2S. Linear relationship between absorbance at 720 nm (H) or between the UCL ratio (J) of UC-PB5 nanoprobes and various concentrations of H2S. Data are represented as mean ± SD. | |
To obtain the as-designed nanoprobes, a PB shell was coated onto UCNPs (UC-PB) by the ligand-exchange and controllable complexation method.26 After successful PB coating, the low contrast shell could be identified in both HR-TEM and scanning transmission electron microscopy (STEM) images (Fig. 1C and D). Energy dispersive X-ray analysis (EDXA) line scanning results found that Fe was well-distributed on the periphery of UCNPs, which further confirmed the existence of the PB shell on UCNP surfaces (Fig. 1D). Furthermore, a new diffraction peak was observed at the 2-theta range of 20–40 degrees, which corresponded to the (2,2,0) and (3,3,1) crystallographic planes of face-centered cubic PB (JCPDS: 52-1907) and indicated PB formation in the as-prepared nanoprobes (Fig. S2B†). The Fe peak in the EDXA spectrum and fabrication of cyanide bonds in the Fourier transform infrared (FTIR) spectrum also illustrated successful PB coating (Fig. S2C and D†). The as-prepared nanoprobes had a strong absorbance peak in the red (600–700 nm) and NIR (700–900 nm) ranges (Fig. S3†), which was attributed to the intervalence charge transfer between Fe(III) and Fe(II) in PB and caused remarkable UCL quenching of UCNPs through both Förster resonance energy transfer (FRET) and inner filter effects (Fig. 1E and F and S4A†). Compared with typical upconversion nanoparticles with relatively low Er dopant concentration (2% Er dopant, UCNPs-L), these UCNPs (20% Er dopant) had stronger UCL in the red and NIR regions, and higher red/green and NIR/green ratios, which were well matched with the absorbance range of PB, and thus were more feasible for constructing PB-functionalized upconversion nanoprobes (Fig. S4B–D†).
As the H2S concentration increased, the absorbance of PB decreased and the UCL of UCNPs recovered accordingly, owing to the decomposition of the PB shells in response to H2S (Fig. 1G and I). To study and optimize the influence of PB thickness on H2S detection performance, nanoprobes with 5 nm (UC-PB5), 8 nm (UC-PB8), and 11 nm (UC-PB11) PB shells were prepared by modulating the reactant ratio (Fig. S5A and B†). As the concentration of serum H2S is within the micromolar range (reported previously as 0–150 μM), the nanoprobes were optimized to be within this range. It was found that all three nanoprobes responded to H2S, while the UCNP itself had no response (Fig. S5C–E†), which highlighted the essential role of PB in the H2S-responsive nanoprobes. As changes in absorbance and UCL were highly correlated with the reacted PB ratio, nanoprobes with thinner PB shells showed higher H2S sensitivity. However, low levels of PB limited the detection range due to limiting amounts of the H2S responsive agent. When the PB shell was <5 nm, serum H2S concentrations were beyond the detection range of the nanoprobes (Fig. S5C–E†). Meanwhile, compared with nanoprobes with thicker shells, UC-PB5 had larger signal changes and was significantly more sensitive in the same H2S concentration range. Therefore, to be within the serum H2S concentration ranges while achieving the balance between a wide detection range and high sensitivity, UC-PB5 nanoprobes were selected. These results also demonstrated that the range and sensitivity of H2S detection could be easily modulated by changing the PB shell thickness, which demonstrated the great adaptability of the PB-functionalized nanoprobes.
Since UC-PB5 nanoprobes were optimized for H2S detection in clinical applications, a detailed evaluation of their performance was conducted. The concentration and absorbance at 720 nm of UC-PB5 nanoprobe suspensions were quantified using ultraviolet-visible-near-infrared (UV-vis-NIR) spectra before being used for detection. We found that the absorbance at 720 nm of a UC-PB5 nanoprobe suspension was negatively correlated with H2S concentration at 0–150 μM (Abs. = 1.184 − 0.007 × [H2S]/μM, R2 = 0.9994, LOD: 10 nM, Fig. 1H). Moreover, using the 550 nm UCL intensity as a reference, the relative 654 nm (abbreviated I654/I550) and 800 nm (abbreviated I800/I550) intensities were positively linearly correlated with Ln[H2S] within the same range (I654/I550 = 0.942 + 1.384 × Ln[H2S]/μM, I800/I550 = 1.202 + 1.770 × Ln[H2S]/μM, R2 = 0.9992, LOD: 10 nM, Fig. 1J). Compared with all previously reported upconversion nanoprobes for H2S detection, UC-PB5 had a favorable detection capacity (Table S1†).23,24,27,28 It is noteworthy that the reaction between UC-PB5 and H2S reached equilibrium within a very short period (approximately 350 s for the spectra and 20 s for the naked eye, Fig. S6 and Video S1†), which indicated that UC-PB5 was feasible for dynamic H2S detection. More importantly, UC-PB5 nanoprobes were well-dispersed in various biological fluids and showed high stability after long-term storage (Fig. S7†). Therefore, UC-PB5 can serve as a dual-modal colorimetric/luminescence probe with favorable biostability and H2S detection capacity (Scheme 1).
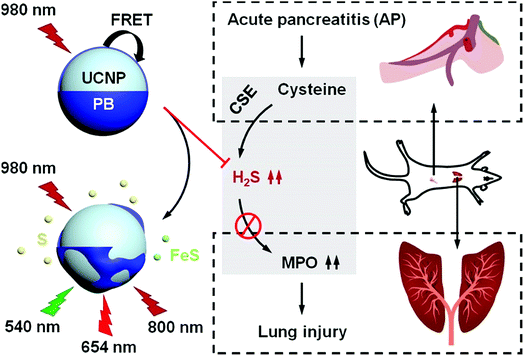 |
| Scheme 1 Schematic illustrations of ultra-highly selective colorimetric/luminescence dual-modal H2S detection and acute pancreatitis (AP)-associated lung injury alleviation using UC-PB5 nanoprobes. CSE: cystathionine-γ-lyase; MPO: myeloperoxidase. | |
Increased H2S selectivity from cooperative redox and combination reactions
Apart from its outstanding H2S detection capacity and high stability, we also noticed that UC-PB5 had ultra-high selectivity for H2S among other biomolecules, including typical bio-reductants and bio-complexes (Fig. S8 and 9†). Moreover, UC-PB5 also showed no response to other bio-sulfur species (typically biothiols, S2O32−, and SO32−), even at high concentrations (15 mM, 100-fold higher than [H2S]; Fig. 2A and B). To uncover the mechanism of this ultra-highly selective H2S detection, the reaction was monitored and products were characterized. After adding UC-PB5 into a H2S solution, the free S2− concentration significantly decreased as a function of time, indicating that H2S may have reacted with UC-PB5 (Fig. 2C). However, because ferrocyanide (also known as Prussian White, reduced PB) can be rapidly oxidized by dissolved oxygen in water, the single redox reaction between UC-PB5 and H2S failed to achieve permanent PB decomposition according to dynamic absorbance spectra (Fig. 2D). Therefore, we concluded that the decomposition of PB by H2S involved cooperation of both redox and combination reactions. To confirm this hypothesis, the products were further characterized. TEM images showed that small nanostructures were formed after adding H2S, while PB shells were rugged and detached from UCNP surfaces (Fig. 2E). Square-wave voltammetry (SWV) curves and Fe2p X-ray photoelectron spectroscopy (XPS) spectra suggested that the Fe(III) in PB was the oxidation reaction center (Fig. 2F and G), while the S2p XPS spectra confirmed the existence of both sulfur and ferrous sulfide, demonstrating the simultaneous redox and combination reactions between UC-PB5 and H2S (Fig. 2H). As there were two coordinated Fe(II) in the reduced form of PB (Fe(II)–C
N– and Fe(II)–N
C–), the combination reaction center was further identified by studying the reaction between H2S and other iron complexes. This showed that only sulfur was produced in the reaction between ferricyanide (containing Fe(II)–C
N– in its reduced form) and H2S, while both sulfur and ferrous sulfide were produced between ferric isothiocyanate (containing Fe(II)–N
C– in its reduced form) and H2S, which was attributed to the stronger coordinative interaction between Fe(II) and C than N in the –C
N– structure (Fig. S11†). Above all, the ultra-highly selective H2S detection using UC-PB5 contributed to the permanent PB decomposition by the cooperation of redox and combination reactions between H2S and PB. The nitrogen-coordinated Fe was either the oxidation or combination reaction center in PB that reacted with H2S, resulting in the H2S-responsiveness of PB.
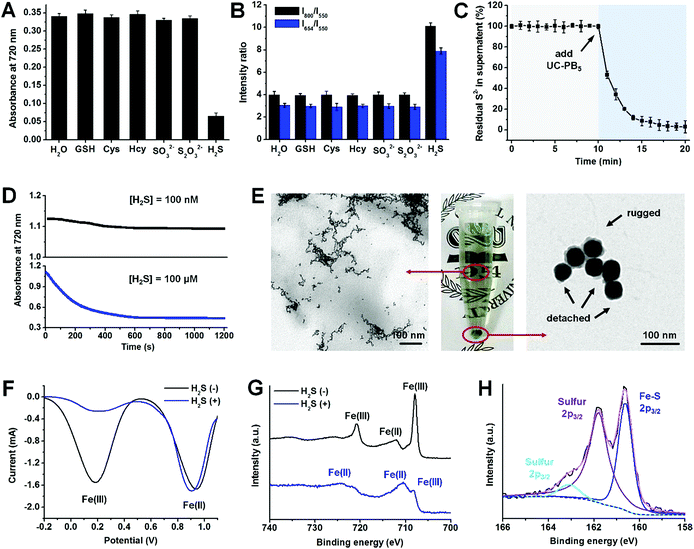 |
| Fig. 2 Mechanism of ultra-high H2S selectivity. Absorbance at 720 nm (A) and UCL ratio (B) of UC-PB5 nanoprobes in response to various biogenic sulfur species. (C) Dynamic free S2− amount in aqueous solution before and after addition of UC-PB5 nanoprobes. (D) Dynamic absorbance of UC-PB5 nanoprobes at 720 nm in response to relatively low (100 nM) and high (100 μM) concentrations of H2S. (E) Photograph of the UC-PB5 nanoprobe suspension after H2S addition and centrifugation. TEM images of the supernatant (left) and precipitate (right). SWV curve (F) and Fe2p XPS spectra (G) of UC-PB5 nanoprobes with/without H2S addition. (H) S2p XPS spectra of UC-PB5 nanoprobes with H2S addition. Data are represented as mean ± SD. | |
Ultra-highly selective ratiometric imaging of H2S
By virtue of the fact that luminescence signals are essential resources for bioimaging, UC-PB5 was a potential H2S-responsive nanophosphor for imaging H2S in cells and in vivo. The signal intensity obtained from luminescence imaging was also useful for evaluating the concentration of H2S in regions or samples of interest. Before applying UC-PB5 to further experimentation, its cytotoxicity and biocompatibility were carefully studied though the PB and upconversion nanoparticles were previously reported to be safe for bioapplications.29,30 Both cell viability assays and SYTO-9/propidium iodide staining suggested that UC-PB5 had no obvious cytotoxicity. It had relatively high IC50 values of 4.05 mg kg−1 and 3.44 mg kg−1 at 24 and 48 h, respectively (Fig. S12†). Furthermore, serological tests and tissue sections also suggested that UC-PB5 had no negative influence on organ function or morphology, demonstrating its good in vivo biocompatibility (Fig. S13 and 14†). These results ensured the safety of UC-PB5 in the subsequent studies.
It is known that H2S can be produced from thiol-containing amino acids under the enzymatic catalysis of cystathionine-γ-lyase (CSE).31 However, DL-propargylglycine (DL-PAG) can irreversibly inhibit CSE by attacking the active-site Tyr114 with its deprotonated β-position, resulting in reduced H2S production.32 In contrast, L-cysteine (L-Cys) and NaHS, which can serve as the substrate and product of this reaction, respectively, can correspondingly alter intracellular H2S levels (Fig. S15A†). Thus, these biomolecules can modulate intracellular H2S concentrations by simulating the environment and regulatory processes. Due to the correlation between the UCL ratio of nanoprobes and H2S concentration, UC-PB5 was feasible for evaluating changes in intracellular H2S levels.
After incubation with UC-PB5, strong UCL was observed in the cytoplasm of living cells, which demonstrated that UC-PB5 could be taken up by cells, which then accumulated H2S-generated electrolytes (Fig. 3A). Dynamic imaging of intracellular H2S also demonstrated that UC-PB5 could rapidly and sensitively respond to changes in the H2S concentration (Fig. 3B). By obtaining UCL intensities from the green (550 ± 20 nm, Abbr. G) and red (650 ± 15 nm, Abbr. R) channels of cells without pretreatment, a standard R/G ratio was calculated and set as the normal intracellular H2S concentration (R/G = 6.28 ± 0.36, Fig. S15B and C†), which corresponded to previous findings. Compared with cells without pretreatment, the R/G ratio (5.49 ± 0.13) in DL-PAG-pretreated cells was significantly lower. In contrast, L-Cys and NaHS pretreated cells had higher R/G ratios (6.92 ± 0.35 for L-Cys and 7.38 ± 0.59 for NaHS) than non-pretreated cells (Fig. S15B†). Importantly, we also found that co-pretreated cells also had a lower H2S concentration (R/G = 5.97 ± 0.28, **p < 0.01) than non-pretreated cells. These results confirmed that UC-PB5 was applicable for the ratiometric imaging of intracellular H2S and could evaluate intracellular H2S concentrations via UCL ratios.
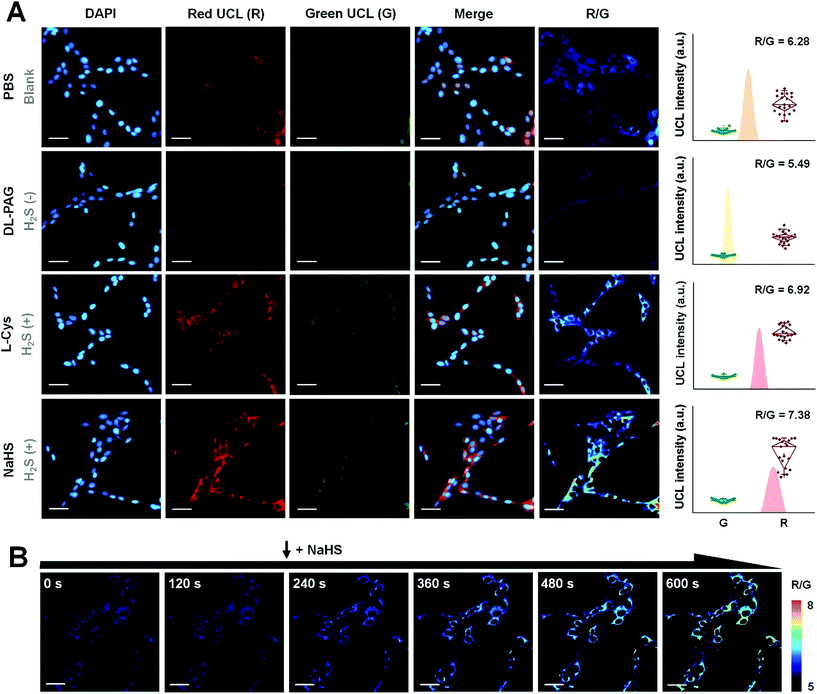 |
| Fig. 3 Evaluation of the intracellular H2S level by ultra-highly selective ratiometric imaging. (A) Luminescence images of A549 cells after various pre-treatments and incubation with UC-PB5 nanoprobes. The nucleus was stained with commercial DAPI staining solution. The ratiometric luminescence images and R/G ratios were calculated from signals collected from the red channel (R) and green channel (G). The corresponding statistics of UCL signal intensity and calculated R/G ratios are also presented. (B) Dynamic ratiometric luminescence images of A549 cells incubated with UC-PB5 nanoprobes before and after NaHS addition. The scale bar was 20 μm. | |
As excessive serum H2S levels can be a potential factor for diagnosing AP, an AP mouse model was established and ratiometric UCL imaging was conducted to evaluate serum H2S concentrations in vivo to verify the feasibility of the developed nanoprobes (Fig. 4A and B and S16†).33 A strong UCL signal was observed in mice that received intravenous injections of UC-PB5 (Fig. 4C). The UCL images of dissected organs suggested that UC-PB5 primarily accumulated in the reticuloendothelial system of mice (Fig. S17†), which corresponded to the biodistribution and metabolic pathway of UC-PB5 (Fig. S18†). It was clear that the UCL signals collected in both the NIR (800 ± 12 nm, Abbr. N) and green (550 ± 20 nm, Abbr. G) ranges from AP mice were higher than those from normal mice. The N/G ratio of AP mice was also significantly higher than that of normal mice (N/G = 9.28 ± 0.69 vs. 7.26 ± 0.75, ***p < 0.001; Fig. 4D). The strong UCL signal and high N/G ratio found in AP mice were attributed to the UCL recovery caused by the reaction between UC-PB5 and high serum H2S levels, which suggested that UC-PB5 was a potential tool for diagnosing AP. More importantly, the CSE-inhibition effect of DL-PAG was also observed by UCL imaging in vivo. At 12 h post-injection of DL-PAG, the N/G ratio was decreased (N/G = 7.89 ± 0.86, ***p < 0.001) and significantly different compared with that of AP mice that received PBS. These results were in accordance with the studies of cultured cells and indicated that CES activity in AP mice could be effectively inhibited by DL-PAG. These results demonstrated the feasibility of using UC-PB5 nanoprobes to evaluate H2S concentration in cells and in vivo by ratiometric imaging, as well as their potential for diagnosing AP.
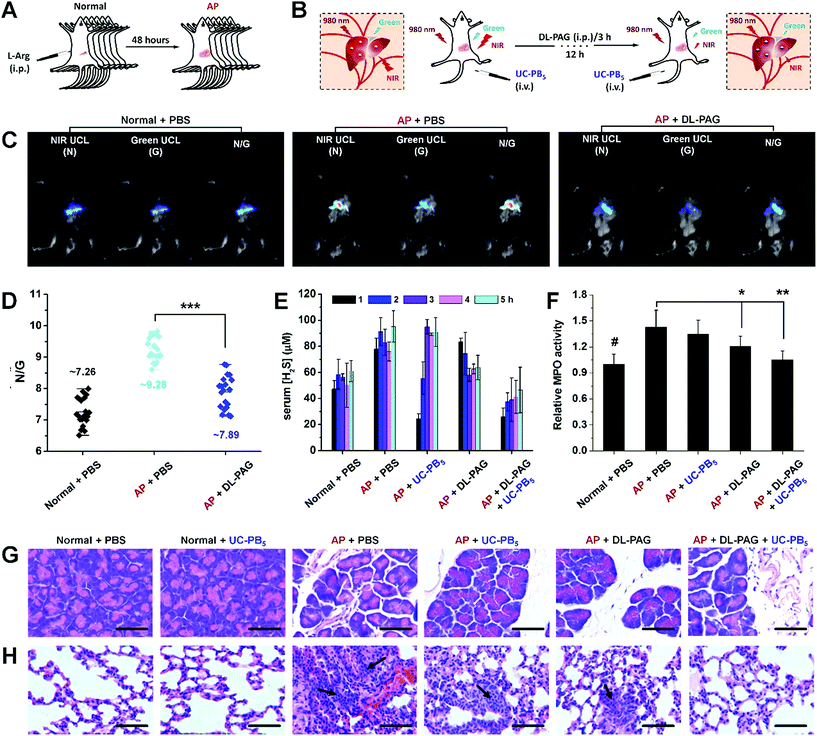 |
| Fig. 4 Evaluation of the serum H2S level and alleviation of AP-associated lung injury in vivo. Schematic illustrations of AP model establishment (A) and theranostics experiments (B). Luminescence images (C) and UCL ratio (D) obtained from mice after various pre-treatments and intravenous injection of UC-PB5 nanoprobes. (E) H2S concentration in serum of mice that received various treatments in the course of 5 h. (F) Relative MPO activity in the lungs of mice that received various treatments. H&E-stained sections of pancreases (G) and lungs (H) harvested from mice that received various treatments. Statistical significance was determined from one-way t tests. *p < 0.05, **p < 0.01, and ***p < 0.001. The scale bar was 50 μm. L-Arg: L-arginine; #: the group as the standard of normalization. Data are represented as mean ± SD. | |
Alleviating AP-associated lung injury in vivo by H2S elimination
Excessive serum H2S levels in AP lead to various complications that can be even worse than AP itself.34 Among these complications, H2S-induced lung injury, including pneumonitis and acute respiratory distress syndrome, are the most serious and are directly responsible for the high early mortality rates in AP cases.35 Therefore, simultaneously inhibiting H2S production and eliminating H2S serum could improve early survival rates.36 However, traditional H2S synthetase inhibitors like DL-PAG are slow-acting and cannot eliminate H2S from serum.37 To meet the requirements of clinical practice and overcome the limitations of H2S synthetase inhibitors, it is essential to develop drugs with rapid H2S elimination capacity. According to the results obtained from H2S detection in vitro and H2S imaging in vivo, the as-designed UC-PB5 nanoprobes could rapidly react with H2S, and thus are expected to eliminate serum H2S within a short period with high efficiency, which would be complementary to inhibitor-based drugs (Fig. S19A†). To test the H2S-elimination effect of UC-PB5, serum H2S concentrations were determined in AP mice that received UC-PB5 nanoprobe injections. These data showed that serum H2S levels in AP mice that received DL-PAG decreased continuously over the first 3 h and remained lower than those in untreated AP mice for the next 2 h. Furthermore, serum H2S levels in AP mice that received UC-PB5 were significantly reduced over the first 2 h of post-injection, which suggested that UC-PB5 rapidly affected serum H2S levels after intravenous injection (Fig. 4E). To test for synergistic effects of UC-PB5 and DL-PAG on H2S levels, AP mice were co-injected with the two agents. Interestingly, serum H2S levels were close to the levels in control mice over the study period, which demonstrated that UC-PB5 and DL-PAG synergistically eliminated H2S from serum.
In most cases, H2S-induced lung injury is associated with abnormal myeloperoxidase (MPO) activation in the lungs, which results in the overproduction of hypochlorous acids and oxidative damage.38 Thus, pulmonary MPO activity was also determined to further illustrate the synergy between UC-PB5 and DL-PAG in alleviating lung injury (Fig. 4F). These data demonstrated that MPO activity was significantly decreased in the lungs of AP mice that received both treatments compared with those that received no or single treatment (UC-PB5 nanoprobes or DL-PAG), and was correlated with the decreased serum H2S levels; hematoxylin and eosin-stained sections also supported these findings (Fig. 4G and H). Lungs harvested from mice that received both treatments had reduced inflammatory reactions and milder pulmonary interstitial edema at the same time points compared with the other mice (Fig. S19B†). These results suggested that co-treatment with UC-PB5 and DL-PAG synergistically alleviated AP-associated lung injury by eliminating H2S from serum and inhibiting H2S production, respectively.
Conclusion
In summary, rationally designed pure-inorganic upconversion nanoprobes using PB as a H2S-responsive acceptor were developed for sensitive and ultra-highly selective H2S detection, imaging, and elimination. To meet the serum H2S concentration range, upconversion nanoprobes with 5 nm PB shells (UC-PB5) were optimized for in vivo studies. The UC-PB5 nanoprobes not only possessed outstanding H2S detection capacity in vitro (linear range: 0–150 μM, LOD: 50 nM), but were also feasible for H2S imaging in cells and in an AP mouse model. Moreover, the rapid H2S elimination by UC-PB5, in cooperation with the long-term inhibition of H2S production by DL-PAG, effectively inhibited H2S-associated MPO activation, which further reduced the oxidative stress in the lungs, alleviating AP-associated lung injury. As PB is an approved drug, our work highlights its great potential for clinical AP treatment by alleviating lung injury, which could reduce the early mortality rate of AP.
Author contributions
Y. L. and J. Z. conceived the project, analyzed the results, and wrote the original manuscript. All authors contributed to the preparation of the original and revised manuscripts. Y. L., Q. J., and X. Z. synthesized the UC-PB nanoprobes. Y. L., A. J., and F. M. investigated the characteristics of the UC-PB nanoprobes. Y. L. and X. Z. performed UCL imaging in vitro and in vivo. Y. L. and Q. J. conducted the other experiments.
Conflicts of interest
There are no conflicts to declare.
Acknowledgements
The authors are thankful for the funding from the Beijing Talent Foundation Outstanding Young Individual Project (2015000026833ZK02), The Joint Foundation Program of Beijing Municipal Natural Science Foundation and Beijing Municipal Education Commission (KZ201810028045), Capacity Building for Sci-Tech Innovation-Fundamental Scientific Research Funds (025185305000/195), Project of High-level Teachers in Beijing Municipal Universities in the Period of the 13th Five-year Plan (IDHT20180517), Project of Construction of Scientific Research Base by the Beijing Municipal Education Commission, Yanjing Young Scholar Development Program of Capital Normal University, and Youth Innovative Research Team of Capital Normal University.
References
- P. Rose, P. K. Moore and Y.-Z. Zhu, Trends Pharmacol. Sci., 2018, 39, 624–634 CrossRef CAS PubMed.
- M. Bhatia, F. L. Wong, D. Fu, H. Y. Lau, S. M. Moochhala and P. K. Moore, FASEB J., 2005, 19, 623–625 CrossRef CAS PubMed.
- P. G. Lankisch, M. Apte and P. A. Banks, Lancet, 2015, 386, 85–96 CrossRef.
- S. Chen, A. Z. Weitemier, X. Zeng, L. He, X. Wang, Y. Tao, A. J. Y. Huang, Y. Hashimotodani, M. Kano, H. Iwasaki, L. K. Parajuli, S. Okabe, D. B. L. Teh, A. H. All, I. Tsutsui-Kimura, K. F. Tanaka, X. Liu and T. J. McHugh, Science, 2018, 359, 679–684 CrossRef CAS PubMed.
- M.-H. Chan, Y.-T. Pan, Y.-C. Chan, M. Hsiao, C.-H. Chen, L. Sun and R.-S. Liu, Chem. Sci., 2018, 9, 3141–3151 RSC.
- C. Li, Y. Zhang, G. Chen, F. Hu, K. Zhao and Q. Wang, Adv. Mater., 2017, 29, 1605754 CrossRef PubMed.
- X. Li, X. Tong, Y. Yin, H. Yan, C. Lu, W. Huang and Q. Zhao, Chem. Sci., 2017, 8, 5930–5940 RSC.
- P. Zhang, H. Wang, Y. Hong, M. Yu, R. Zeng, Y. Long and J. Chen, Biosens. Bioelectron., 2018, 99, 318–324 CrossRef CAS PubMed.
- P. Zhang, X.-f. Jiang, X. Nie, Y. Huang, F. Zeng, X. Xia and S. Wu, Biomaterials, 2016, 80, 46–56 CrossRef CAS PubMed.
- P. Zhang, X. Nie, M. Gao, F. Zeng, A. Qin, S. Wu and B. Z. Tang, Mater. Chem. Front., 2017, 1, 838–845 RSC.
- P. Zhang, Y. Tian, H. Liu, J. Ren, H. Wang, R. Zeng, Y. Long and J. Chen, Chem. Commun., 2018, 54, 7231–7234 RSC.
- X. Zhu, Q. Su, W. Feng and F. Li, Chem. Soc. Rev., 2017, 46, 1025–1039 RSC.
- L. Sun, R. Wei, J. Feng and H. Zhang, Coord. Chem. Rev., 2018, 364, 10–32 CrossRef CAS.
- W. Wei, G. Chen, A. Baev, G. S. He, W. Shao, J. Damasco and P. N. Prasad, J. Am. Chem. Soc., 2016, 138, 15130–15133 CrossRef CAS PubMed.
- W. Fan, W. Bu and J. Shi, Adv. Mater., 2016, 28, 3987–4011 CrossRef CAS PubMed.
- J. Xu, W. Han, Z. Cheng, P. Yang, H. Bi, D. Yang, N. Niu, F. He, S. Gai and J. Lin, Chem. Sci., 2018, 9, 3233–3247 RSC.
- L. Zhou, Y. Fan, R. Wang, X. Li, L. Fan and F. Zhang, Angew. Chem., Int. Ed., 2018, 57, 12824–12829 CrossRef CAS PubMed.
- Y. Liu, A. Jiang, Q. Jia, X. Zhai, L. Liu, L. Ma and J. Zhou, Chem. Sci., 2018, 9, 5242–5251 RSC.
- X. Hu, Y. Wang, H. Liu, J. Wang, Y. Tan, F. Wang, Q. Yuan and W. Tan, Chem. Sci., 2017, 8, 466–472 RSC.
- P. Huang, W. Zheng, S. Zhou, D. Tu, Z. Chen, H. Zhu, R. Li, E. Ma, M. Huang and X. Chen, Angew. Chem., Int. Ed., 2014, 53, 1252–1257 CrossRef CAS PubMed.
- Q. Su, W. Feng, D. Yang and F. Li, Acc. Chem. Res., 2017, 50, 32–40 CrossRef CAS PubMed.
- B. Gu and Q. Zhang, Adv. Sci., 2018, 5, 1700609 CrossRef PubMed.
- J. Peng, C. L. Teoh, X. Zeng, A. Samanta, L. Wang, W. Xu, D. Su, L. Yuan, X. Liu and Y.-T. Chang, Adv. Funct. Mater., 2015, 26, 191–199 CrossRef.
- Y. Zhou, W. Chen, J. Zhu, W. Pei, C. Wang, L. Huang, C. Yao, Q. Yan, W. Huang, J. S. C. Loo and Q. Zhang, Small, 2014, 10, 4874–4885 CrossRef CAS PubMed.
- B. Kong, C. Selomulya, G. Zheng and D. Zhao, Chem. Soc. Rev., 2015, 44, 7997–8018 RSC.
- Y. Liu, Q. Guo, X. Zhu, W. Feng, L. Wang, L. Ma, G. Zhang, J. Zhou and F. Li, Adv. Funct. Mater., 2016, 26, 5120–5130 CrossRef CAS.
- S. Liu, L. Zhang, T. Yang, H. Yang, K. Y. Zhang, X. Zhao, W. Lv, Q. Yu, X. Zhang, Q. Zhao, X. Liu and W. Huang, ACS Appl. Mater. Interfaces, 2014, 6, 11013–11017 CrossRef CAS PubMed.
- Z. Gao, L. Wang, L. Wang and H. Chen, Anal. Methods, 2017, 9, 835–840 RSC.
- L. Cheng, H. Gong, W. Zhu, J. Liu, X. Wang, G. Liu and Z. Liu, Biomaterials, 2014, 35, 9844–9852 CrossRef CAS PubMed.
- A. Gnach, T. Lipinski, A. Bednarkiewicz, J. Rybka and J. A. Capobianco, Chem. Soc. Rev., 2015, 44, 1561–1584 RSC.
- G. Yang, L. Wu, B. Jiang, W. Yang, J. Qi, K. Cao, Q. Meng, A. K. Mustafa, W. Mu, S. Zhang, S. H. Snyder and R. Wang, Science, 2008, 322, 587–590 CrossRef CAS PubMed.
- D. Ezeriņa, Y. Takano, K. Hanaoka, Y. Urano and T. P. Dick, Cell Chem. Biol., 2018, 25, 447–459 CrossRef PubMed.
-
M. Bhatia, in Methods Enzymol., ed. E. Cadenas and L. Packer, 2015, vol. 555, pp. 195–205 Search PubMed.
- M. Bhatia, A. K. Saluja, B. Hofbauer, J.-L. Frossard, H. S. Lee, I. Castagliuolo, C.-C. Wang, N. Gerard, C. Pothoulakis and M. L. Steer, Proc. Natl. Acad. Sci. U. S. A., 1998, 95, 4760–4765 CrossRef CAS.
- T. Dombernowsky, M. Ø. Kristensen, S. Rysgaard, L. L. Gluud and S. Novovic, Pancreatology, 2016, 16, 756–760 CrossRef PubMed.
- R. K. Velusamy and R. Tamizhselvi, J. Pharm. Pharmacol., 2018, 70, 1188–1199 CrossRef CAS PubMed.
- Z. Qu, Y. Jiang, B.-Q. Wu, Y.-F. Duan, Z.-D. Sun and G.-H. Luo, Arch. Med. Sci., 2014, 10, 825–829 CrossRef CAS PubMed.
- A. Warris and E. R. Ballou, Semin. Cell Dev. Biol., 2018 DOI:10.1016/j.semcdb.2018.1003.1004.
Footnote |
† Electronic supplementary information (ESI) available. See DOI: 10.1039/c8sc04464c |
|
This journal is © The Royal Society of Chemistry 2019 |
Click here to see how this site uses Cookies. View our privacy policy here.