DOI:
10.1039/C9SC01777A
(Edge Article)
Chem. Sci., 2019,
10, 6785-6790
A remarkably strained cyclopyrenylene trimer that undergoes metal-free direct oxygen insertion into the biaryl C–C σ-bond†
Received
11th April 2019
, Accepted 11th June 2019
First published on 12th June 2019
Abstract
A remarkably strained cyclopyrenylene trimer CP3 was synthesized and it underwent the first biaryl C–C σ-bond cleavage by direct oxygen insertion without the aid of any metal agents. A priori highly strained CP3 exhibits the longest wavelength emission among all pyrene-based fluorophores due to the intensive electronic interactions between pyrenes. The color of the emission drastically changes from orange to light blue upon oxidation. Theoretical studies revealed that the release of ring strain reasonably drives the reaction between two CP3 molecules and O2. This strain-induced transformation could be also applied for sulfur atom insertion into a biaryl σ-bond.
Introduction
Cycloarylenes such as cycloparaphenylenes (CPPs) have attracted interest due to their unique structure, remarkable physical properties, and potential applications in materials science.1–4 The remarkable characteristics of CPPs are often derived from their curved π-conjugated systems. Recently, Yamago and co-workers reported two C–C σ-bond cleavages of [5]- and [6]CPPs with a platinum salt5 driven by their internal strain energy6 (the values are significantly higher than those of cyclopropane).7 In organic chemistry, a molecule experiences strain when the molecule is held in an energetically unfavorable conformation by intramolecular covalent bonds in comparison to a strain-free reference compound.8 After cutting off the particular bonds, the strain energy would be released. Ring strain is a type of instability that results from a combination of angle strain (Baeyer strain), torsional strain (Pitzer strain), and transannular strain known as van der Waals (or Prelog) strain.9 Angle strain occurs to achieve maximum bond strength in a specific conformation in which the bond angles deviate from the ideal bond angles. The potential energy and unique bonding structure present in the molecules with ring strain can be used to drive reactions in organic synthesis. Examples of such reactions are small ring opening reactions, photo-induced ring opening of cyclobutenes and nucleophilic ring-opening of epoxides.10–12 Strain-induced C–C bond activation with transition metals has been long known.13 As another type of example, spherical sp2 carbons in fullerenes tend to react as electrophiles.14 The driving force is the release of strain when double bonds become saturated.
Here we report the synthesis and reactivity of a remarkably strained cyclopyrenylene trimer CP3 (Scheme 1). Since the first synthesis of diphenylethers by Cook in 1901,15 almost all biaryl ether syntheses so far developed have relied on the Williamson-type nucleophilic aromatic substitution. The only exceptions are the metal-mediated oxidation reactions of biphenylenes (Scheme 1).11 In this work, direct oxygen atom insertion into a biaryl C–C σ-bond is achieved for the first time under ambient conditions without any aid of metal agents.
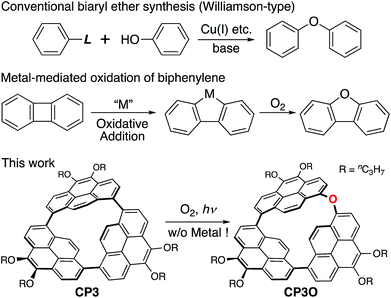 |
| Scheme 1 General biaryl ether synthesis, metal-mediated oxidation of a biphenylene, and direct oxygen insertion into a highly strained C–C σ-bond. | |
Results and discussion
Synthesis and structural characterization
Macrocyclic arylene CP3 was synthesized by a Ni-mediated coupling reaction of 1,8-dibromo-4,5-dipropoxypyrene16 according to reported procedures.17–19 We performed a one-pot macrocyclization reaction of the dibromopyrene with Ni(cod)2/2,2′-bipyridine in a mixture of toluene and DMF (76.0 mM). CP3 was successfully isolated from higher oligomers using silica gel and gel permeation chromatography in 3.5% yield.
High-resolution matrix-assisted-laser-desorption/ionization spiral time-of-flight mass spectroscopy (HR-MALDI-TOF-MS) detected the parent ion peak at m/z = 948.4384 (calcd for C66H60O6 = 948.4383 [M]+). The 1H NMR spectrum of CP3 in CDCl3 reveals only a single set of signals that consists of one singlet peak at 6.99 ppm due to protons at the 9,10-positions and two doublet peaks at 8.60 and 8.67 ppm due to protons at the 2,7- and 3,6-positions, respectively. These data indicate that the cyclic pyrene CP3 adopts a D3h symmetric structure in solution.
The structure of CP3 has been unambiguously revealed by X-ray diffraction analysis.20 The smoothly curved pyrene 1 and pyrene 3 in Fig. 1a were held with a small torsion angle (19.5(6)°), which stands in marked contrast to conventional perpendicular 1,1′-linked pyrene dimers.21 The π-orbital axis vector (POAV) angles, the degree of the pyramidalization of trigonal carbon atoms,22 were calculated based on the crystal structure (Fig. 1b). Two of the largest POAV values (4.88° and 4.04°) were found at C(1) and C(54) atoms, respectively, but these angle strains are not as high as those of C60 (11.64°) and [6]CPP (6.10°).23 It is noticed that the C(1)–C(54) bond length of the curved bipyrene unit is 1.546(5) Å, being distinctly longer than that of the normal CAr–CAr bond (av. 1.49 Å).24 Surprisingly, the distance between C(13) and C(56) atoms (10,10′-positions) of the coplanar bipyrene is 2.88 Å, much shorter than the sum of van der Waals radii of carbon atoms (ca. 3.40 Å), thus resulting in the negative Gaussian curvature25 of two encountered six-membered rings with transannular strain (Fig. 1b). In total, the ring strain affects the elongation of the C(1)–C(54) σ-bond. The conformation of the coplanar bipyrene unit has the same shape as a rotational transition state of binaphthyl via the syn-Cs form,26 letting us easily imagine its high energy state.
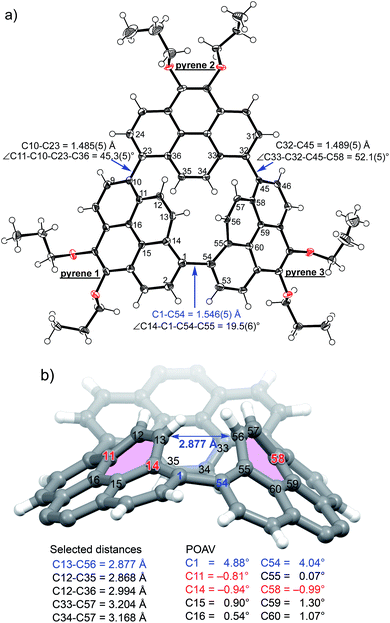 |
| Fig. 1 Single crystal X-ray structure of CP3 and selected distances, angles and POAV values. (a) ORTEP drawing (50% probability) of the top view and (b) ball-and-stick model of the side view. Two six-membered rings with negative Gaussian curvature are shown in pink. | |
Photophysical properties
CP3 absorbs UV-vis light with an absorption maximum at 505 nm, and emits fluorescence at 599 nm with a quantum yield of ΦF = 0.34 at 298 K along with a small short wavelength emission (vide infra) (Fig. 2). Curiously, this red-shifted emission of CP3 is much longer than that of the pyrene excimer (λmax = 475 nm in hexane)27,28 and any fused pyrene dimers (λmax = up to 525 nm; Chart 1b–d).29–33 Most importantly, this is even longer than that of the completely fused corresponding pyrene trimer (λmax = 530–570 nm, Chart 1e).34 To the best of our knowledge, this is the most red-shifted emission among all pyrene-based fluorophores besides acetylene-linked cyclic trimers and tetramers.16 These properties result from the intensive electronic interaction between pyrene units in the highly strained structure.
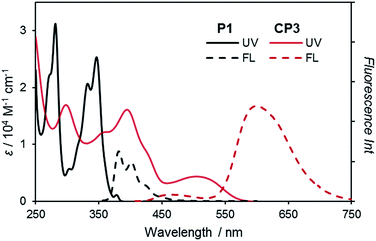 |
| Fig. 2 (a) UV-vis (solid line) and fluorescence (broken line) spectra of CP3 (in red) along with those of 4,5-dipropoxypyrene (P1) (in black) in CH2Cl2, [CP3] = 1.5 × 10−5 M. Fluorescence spectra were recorded for excitation (λex = 346 nm for P1, λex = 395 nm for CP3) with the absorbance adjusted to 0.1. | |
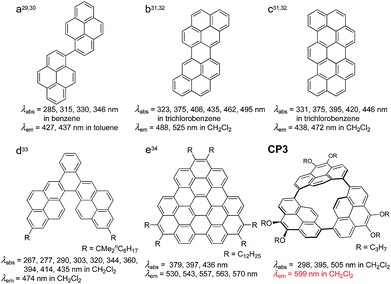 |
| Chart 1 | |
DFT calculations
To further understand these unusual electronic features, density functional theory (DFT) and time-dependent (TD)-DFT calculations both at the B3LYP-D3/6-311G(d,p)//B3LYP-D3/6-31G(d) level including the SMD solvation model with a dielectric constant of dichloromethane were carried out (Fig. 3 and the computational details are provided in the ESI†).35 The highest occupied molecular orbital (HOMO) and lowest unoccupied molecular orbital (LUMO) are non-degenerative and the coefficient distribution in these frontier MOs appears delocalized over pyrenes 1 and 3. The longest band of CP3 at 548 nm mainly comprises the transition from high HOMO (−5.00 eV) to low LUMO (−2.37 eV) relative to a linear pyrene trimer P3 (HOMO: −5.31 eV, LUMO: −1.89 eV, Fig. S20†) with the oscillator strength, f = 0.279. The red-shifted absorption and emission were predominantly achieved by the stabilized LUMO relative to P3, which is caused by the π*–π* conjugation between C(13) and C(56) atoms. The transition energies and oscillator strengths simulated by TD-DFT showed good agreement with the observed absorption spectrum (Fig. S18†).
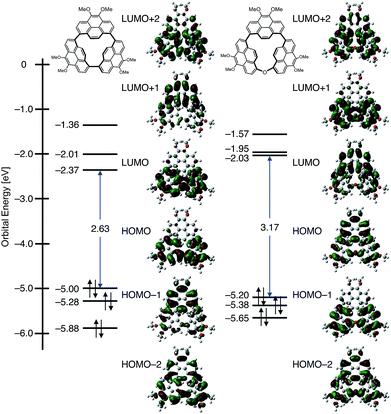 |
| Fig. 3 MO diagrams of CP3 and CP3O based on calculations at the B3LYP-D3/6-311G(d,p)//B3LYP-D3/6-31G(d) including the SMD solvation model. Propyl groups were replaced by Me groups. | |
C–C σ-bond cleavage by oxidation
Meanwhile, we found that the short wavelength emission gradually enhanced under ambient conditions. We measured fluorescence spectral changes under room light in CH2Cl2 (Fig. 4). The fluorescence of CP3 clearly indicates a new peak at 480 nm with the isosbestic point at 553 nm, from which a 1
:
1 transformation was expected. The color of the emission drastically changes from orange to light blue (Fig. 4 inset). From the time profile, we have determined the half lifetime to be 18 h.
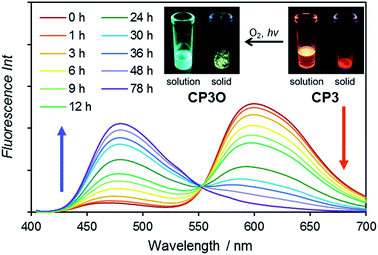 |
| Fig. 4 Fluorescence spectral change of CP3 under room light in CH2Cl2. Insets are photos of the solution (CH2Cl2) and solid of CP3 and CP3O under UV irradiation. | |
After 78 h, CP3 was completely transformed. The structure of the product was initially characterized by 1H NMR spectroscopy. In the 1H NMR spectrum, 9 peaks in the aromatic region appear and the protons at the 10,10′-positions of the coplanar pyrene rings are significantly deshielded from δ = 6.99 ppm to 8.90 ppm as a doublet (d, J = 10.0 Hz). All these spectra are consistent with the product having C2v symmetry, lowering from D3h. HR-MALDI-TOF-MS of the product detected a CP3 + 16 mass unit at m/z = 964.4347 (calcd for C66H60O7 = 964.4334 [M]+), suggesting the insertion of one oxygen atom.
The structure was finally determined by single crystal X-ray diffraction analysis (Fig. 5).20 The product has an arched structure with an oxygen atom between two pyrenes, thus revealing that the cyclic ether CP3O was quantitatively formed.36 All the structural parameters suggested that the ring strain is no longer involved, predicted from the atom–atom distances and torsion angles (Fig. 5). The larger dihedral angles make the electronic interactions between pyrenes weak, resulting in a blue-shifted absorption and emission spectra with ΦF = 0.54. The reaction is sensitive to the solvent and proceeded smoothly in CH2Cl2 and CHCl3. We confirmed that the oxygen atom originates from molecular oxygen by reacting in H218O saturated CH2Cl2 to generate CP316O. No C–C bond activation occurred without light, thus indicating that 1O2* (1Δg) reacts with CP3. To confirm the presence of singlet O2 derived from the excitation of CP3, we performed singlet O2 sensitization and measured the emission signal (λem = 1274 nm) upon selective excitation of CP3 at λex = 515 nm in O2-saturated CH2Cl2 (Fig. S17†). The quantum yield of singlet oxygen production (ΦΔ) determined with respect to ΦΔ = 0.60 for tetraphenylporphyrin (TPP)37 is 0.33 at 298 K. Although the pyrene monomer is known as an efficient sensitizer for O2 with high ΦΔ,38 it can absorb only UV light (Fig. 2). The absorption spectrum of CP3 shows red-shifted broad bands up to ca. 600 nm, so the singlet O2 could be generated by room light.
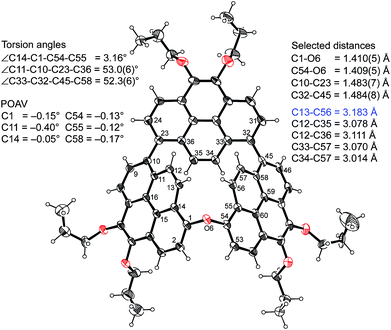 |
| Fig. 5 Single crystal X-ray structure and selected distances, angles and POAV values of CP3O. The thermal ellipsoids are shown at 25% probability. One of the two identical molecules in the crystallographic asymmetric unit of CP3O is shown. | |
The strain energy of CP3 has been estimated on the basis of a homodesmotic reaction using CP3, dimer P2, and trimer P3 (Scheme 2).38 By using the heat of formation (ΔH) of the optimized structures of CP3, P2, and P3, the strain energy of CP3 was obtained as 251 kJ mol−1 (Fig. S20†). This is a higher strain energy per unit in comparison to [7]CPP.39
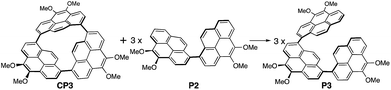 |
| Scheme 2 A hypothetical homodesmotic reaction for the calculation of strain energies of CP3. Propyl groups were replaced by Me groups. | |
Investigation of the reaction mechanism by DFT calculations
To elucidate the mechanism of C–C activation of CP3, the reaction pathway starting from CP3 and a singlet oxygen molecule was explored using an automated reaction path search method, called the artificial force induced reaction (AFIR) method, combined with the DFT level of theory. As shown in Scheme 3, the bond formation between C(1) (or C(54) in Fig. 1) and oxygen took place without a barrier to form intermediates INT1 or INT2, followed by C–O bond formation with another CP3 affording INT3. As shown in Fig. S22 and S23,† the negative charge localized on the peroxide moiety in INT3 caused the partial occupancy of the σ* orbital of the peroxide moiety, which induced the decrease of the O–O bond order, i.e. O–O bond cleavage. After the formation of two neutral intermediates, INT4, the intramolecular rearrangement proceeded via a three-center transition state, which afforded the final product CP3O. The high exothermicity could be attributed to the release of strain upon transformation from INT4 to CP3O.
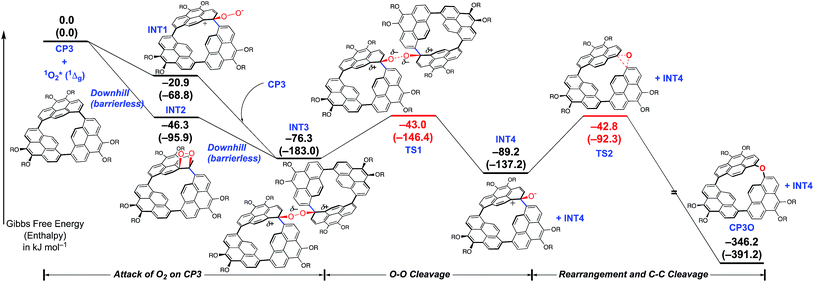 |
| Scheme 3 Gibbs free energy profile (ΔG in kJ mol−1) of the formation of CP3O starting from CP3 and a singlet oxygen molecule. The enthalpy differences are shown in parentheses. Propyl groups were replaced by Me groups. All the calculations were carried out at the B3LYP-D3/6-311G(d,p)//B3LYP-D3/6-31G(d) level including the SMD solvation model. Computational details and geometries are shown in the ESI.† | |
C–C σ-bond cleavage by sulfidation
Finally, the transformation of CP3 into a cyclic thioether was also examined by using Na2S·9H2O as a reagent (Scheme 4). Treatment of CP3 with Na2S·9H2O in DMF at 80 °C for 72 h gave a sulfur inserted cyclic trimer CP3S in 67% conversion yield. CP3S exhibits light green emission with ΦF = 0.44. This is also the first direct sulfidation of a biaryl C–C σ-bond.36 Synthesis of biaryl thioethers is considered as a valuable C–C bond activation process because transformations of diaryl sulfides and sulfones to the corresponding biaryl compounds have been developed.40
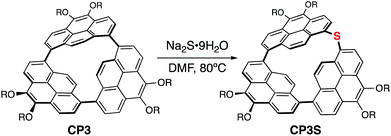 |
| Scheme 4 Sulfur atom insertion into the C–C σ-bond of CP3. R = nC3H7. | |
Conclusions
In summary, we could isolate a highly strained cyclic pyrene trimer that underwent direct etherification at room temperature. This is the first example of biaryl C–C σ-bond cleavage under metal-free conditions. Because CP3 is the most bathochromic pyrene, a vivid color change of fluorescence is observed upon oxidation, being advantageous for the use of CP3 as a fluorescence probe for the imaging of singlet O2 with relatively high ΦF.41 We revealed that this unusual reactivity originates from the intense intramolecular energy in the highly strained structure. Besides, recently, O-doping of polycyclic aromatic hydrocarbons (PAHs) is emerging as a powerful strategy to tailor the optoelectronic properties of π-conjugated molecular modules.42 This study presents a new concept for the preparation of potential sensing agents and O-doped PAHs. We would insert other elements into CP3 by which the optical and electronic properties can be controlled. Such possibilities are now under investigation and will be reported in due course.
Conflicts of interest
There are no conflicts to declare.
Acknowledgements
This work was partly supported by the Japan Society for the Promotion of Science (JSPS) KAKENHI Grant No. JP18K14190 (H. H.), JP18K14298 (M. S.), JP17H06445 (M. H.), JP17H03042 (N. A.) and JP26105004 (H. Y.). We thank Prof. A. Osuka (Kyoto Univ.) and Prof. K. Mizuno (NAIST) for fruitful discussion and, Profs. T. Kawai and T. Nakashima (NAIST) for measurements of fluorescence quantum yields and Ms Y. Nishikawa for MS measurements. We also acknowledge the computer resources provided by the Academic Center for Computing and Media Studies (ACCMS) at Kyoto University.
Notes and references
- S. Toyota and E. Tsurumaki, Chem.–Eur. J., 2019, 25, 6878–6890 CrossRef CAS PubMed
.
- M. Iyoda and H. Shimizu, Chem. Soc. Rev., 2015, 44, 6411–6424 RSC
.
- X. Lu, S. Lee, Y. Hong, H. Phan, T. Y. Gopalakrishna, T. S. Herng, T. Tanaka, M. E. Sandoval, W. Zeng, J. Ding, D. Casanova, A. Osuka, D. Kim and J. Wu, J. Am. Chem. Soc., 2017, 139, 13173–13183 CrossRef CAS PubMed
.
- M. A. Majewski and M. Stępień, Angew. Chem., Int. Ed., 2019, 58, 86–116 CrossRef CAS PubMed
.
- E. Kayahara, T. Hayashi, K. Takeuchi, F. Ozawa, K. Ashida, S. Ogoshi and S. Yamago, Angew. Chem., Int. Ed., 2018, 57, 11418–11421 CrossRef CAS
.
- T. Iwamoto, Y. Watanabe, Y. Sakamoto, T. Suzuki and S. Yamago, J. Am. Chem. Soc., 2011, 133, 8354–8361 CrossRef CAS PubMed
.
- S. W. Benson, F. R. Cruickshank, D. M. Golden, G. R. Haugen, H. E. O'Neal, A. S. Rodgers, R. Shaw and R. Walsh, Chem. Rev., 1969, 69, 279–324 CrossRef CAS
.
- K. B. Wiberg, Angew. Chem., Int. Ed. Engl., 1986, 25, 312–322 CrossRef
.
-
E. V. Anslyn and D. A. Dougherty, in Modern Physical Organic Chemistry, Chapter 2: Strain and Stability, University Science Books, Sausalito, California, 2006, pp. 100–109 Search PubMed
.
-
A. Greenberg and J. F. Liebman, in Strained Organic Molecules, ed. H. H. Wasserman, Academic Press, 1st edn, 1978, vol. 38 Search PubMed
.
- L. Souillart and N. Cramer, Chem. Rev., 2015, 115, 9410–9464 CrossRef CAS PubMed
.
- G. Fumagalli, S. Stanton and J. F. Bower, Chem. Rev., 2017, 117, 9404–9432 CrossRef CAS PubMed
.
-
M. Murakami and N. Chatani, in Cleavage of Carbon-Carbon Single Bonds by Transition Metals, Wiley-VCH, Weinheim, 2015 Search PubMed
.
- M. Prato, V. Lucchini, M. Maggini, E. Stimpfl, G. Scorrano, M. Eiermann, T. Suzuki and F. Wudl, J. Am. Chem. Soc., 1993, 115, 8479–8480 CrossRef CAS
.
- A. N. Cook, J. Am. Chem. Soc., 1901, 23, 806–813 CrossRef
.
- G. Venkataramana, P. Dongare, L. N. Dawe, D. W. Thompson, Y. Zhao and G. J. Bodwell, Org. Lett., 2011, 13, 2240–2243 CrossRef CAS PubMed
.
- T. Yamamoto, Prog. Polym. Sci., 1992, 17, 1153–1205 CrossRef CAS
; T. Yamamoto, Y. Hayashi and A. Yamamoto, Bull. Chem. Soc. Jpn., 1978, 51, 2091–2097 CrossRef
.
- J. Y. Xue, K. Ikemoto, N. Takahashi, T. Izumi, H. Taka, H. Kita, S. Sato and H. Isobe, J. Org. Chem., 2014, 79, 9735–9739 CrossRef CAS
.
- Y. Yamamoto, E. Tsurumaki, K. Wakamatsu and S. Toyota, Angew. Chem., Int. Ed., 2018, 57, 8199–8202 CrossRef CAS PubMed
.
-
CP3: C66H60O6, Mw = 3493.9, triclinic, space group P
(no. 2), a = 8.9771(10), b = 17.626(2), c = 17.763(2) Å, α = 116.5169(19), β = 93.784(2), γ = 102.495(2)°, V = 2412.5(5) Å3, Z = 2, T = 90(2) K, Dcalcd = 1.307 g cm−3, R1 = 0.0738 (I > 2σ(I)), Rw = 0.2024 (all data), GOF = 1.061. CP3O: C66H60O7 (methanol)0.59 (dichloromethane)0.39, Mw = 1012.83, triclinic, space group P
(no. 2), a = 17.7176(19), b = 18.088(2), c = 18.855(2) Å, α = 109.8071(19), β = 114.3435(19), γ = 91.189(2)°, V = 5088.2(10) Å3, Z = 4, T = 90(2) K, Dcalcd = 1.322 g cm−3, R1 = 0.0829 (I > 2σ(I)), Rw = 0.2715 (all data), GOF = 1.025. CCDC 1907392 and 1907393 contain the supplementary crystallographic data for this paper.
- A. Matsumoto, M. Suzuki, H. Hayashi, D. Kuzuhara, J. Yuasa, T. Kawai, N. Aratani and H. Yamada, Bull. Chem. Soc. Jpn., 2017, 90, 667–677 CrossRef CAS
.
- R. C. Haddon and L. T. Scott, Pure Appl. Chem., 1986, 58, 137–142 Search PubMed
; R. C. Haddon, J. Am. Chem. Soc., 1987, 109, 1676–1685 CrossRef CAS
; R. C. Haddon, J. Phys. Chem., 1987, 91, 3719–3720 CrossRef
; R. C. Haddon, Science, 1993, 261, 1545–1550 CrossRef PubMed
.
- Y. Segawa, A. Yagi, H. Ito and K. Itami, Org. Lett., 2016, 18, 1430–1433 CrossRef CAS PubMed
.
-
F. H. Allen, D. G. Watson, L. Brammer, A. G. Orpen and R. Taylor, in International Tables for Crystallography, ed. E. Prince, International Union of Crystallography, 2006, vol. C, ch. 9.5, pp. 790–811 Search PubMed
.
- S. H. Pun and Q. Miao, Acc. Chem. Res., 2018, 51, 1630–1642 CrossRef CAS PubMed
.
- L. Meca, D. Řeha and Z. Havlas, J. Org. Chem., 2003, 68, 5677–5680 CrossRef CAS PubMed
.
- Th. Förster and K. Kasper, Z. Phys. Chem., 1954, 1, 275–277 CrossRef
.
-
J. B. Birks, in Photophysics of Aromatic Molecules, Wiley, London, 1970, pp. 300–371 Search PubMed
.
- V. A. Nefedov, Russ. J. Org. Chem., 2007, 43, 1163–1166 CrossRef CAS
.
- R. A. Velapoldi, J. Res. Natl. Bur. Stand., Sect. A, 1972, 76, 641–654 CrossRef CAS
.
- E. Clar and O. Kühn, Liebigs Ann., 1956, 601, 181–192 CrossRef CAS
.
- S. A. Tucker, A. I. Zvaigzne, W. E. Acree Jr, J. C. Fetzer and M. Zander, Appl. Spectrosc., 1991, 45, 424–428 CrossRef CAS
.
- K.-i. Yamashita, A. Nakamura, Md. A. Hossain, K. Hirabayashi, T. Shimizu and K.-i. Sugiura, Bull. Chem. Soc. Jpn., 2015, 88, 1083–1085 CrossRef CAS
, Sugiura’s dimer has π-conjugative planar bipyrene and emits at 474 nm, so the long wavelength emission of CP3 originates from its highly strained structure. See text.
- M. Kastler, J. Schmidt, W. Pisula, D. Sebastiani and K. Müllen, J. Am. Chem. Soc., 2006, 128, 9526–9534 CrossRef CAS PubMed
.
-
M. J. Frisch, G. W. Trucks, H. B. Schlegel, G. E. Scuseria, M. A. Robb, J. R. Cheeseman, G. Scalmani, V. Barone, B. Mennucci, G. A. Petersson, H. Nakatsuji, M. Caricato, X. Li, H. P. Hratchian, A. F. Izmaylov, J. Bloino, G. Zheng, J. L. Sonnenberg, M. Hada, M. Ehara, K. Toyota, R. Fukuda, J. Hasegawa, M. Ishida, T. Nakajima, Y. Honda, O. Kitao, H. Nakai, T. Vreven, J. A. Montgomery Jr, J. E. Peralta, F. Ogliaro, M. Bearpark, J. J. Heyd, E. Brothers, K. N. Kudin, V. N. Staroverov, R. Kobayashi, J. Normand, K. Raghavachari, A. Rendell, J. C. Burant, S. S. Iyengar, J. Tomasi, M. Cossi, N. Rega, J. M. Millam, M. Klene, J. E. Knox, J. B. Cross, V. Bakken, C. Adamo, J. Jaramillo, R. Gomperts, R. E. Stratmann, O. Yazyev, A. J. Austin, R. Cammi, C. Pomelli, J. W. Ochterski, R. L. Martin, K. Morokuma, V. G. Zakrzewski, G. A. Voth, P. Salvador, J. J. Dannenberg, S. Dapprich, A. D. Daniels, O. Farkas, J. B. Foresman, J. V. Ortiz, J. Cioslowski and D. J. Fox, Gaussian 09, Revision E.1, Gaussian, Inc., Wallingford CT, 2009 Search PubMed
.
- Aromatization-driven oxygen and sulfur insertion reactions into a norcorrole (cyclic dipyrrin dimer) were reported. T. Ito, Y. Hayashi, S. Shimizu, J.-Y. Shin, N. Kobayashi and H. Shinokubo, Angew. Chem., Int. Ed., 2012, 51, 8542–8545 CrossRef CAS PubMed
.
- D. Yao, V. Hugues, M. Blanchard-Desce, O. Mongin, C. O. Paul-Roth and F. Paul, New J. Chem., 2015, 39, 7730–7733 RSC
.
- K. L. Marsh and B. Stevens, J. Phys. Chem., 1983, 87, 1765–1768 CrossRef CAS
.
- Y. Segawa, H. Omachi and K. Itami, Org. Lett., 2010, 12, 2262–2265 CrossRef CAS
.
- K. Nogi and H. Yorimitsu, Chem. Commun., 2017, 53, 4055–4065 RSC
.
- S. Kim, T. Tachikawa, M. Fujitsuka and T. Majima, J. Am. Chem. Soc., 2014, 136, 11707–11715 CrossRef CAS PubMed
.
- Y. Wang, S. Qiu, S. Xie, L. Zhou, Y. Hong, J. Chang, J. Wu and Z. Zeng, J. Am. Chem. Soc., 2019, 141, 2169–2176 CrossRef CAS PubMed
; A. Berezin, N. Biot, T. Battisti and D. Bonifazi, Angew. Chem., Int. Ed., 2018, 57, 8942–8946 CrossRef
; D. Stassen, N. Demitri and D. Bonifazi, Angew. Chem., Int. Ed., 2016, 55, 5947–5951 CrossRef PubMed
; K. Nakanishi, D. Fukatsu, K. Takaishi, T. Tsuji, K. Uenaka, K. Kuramochi, T. Kawabata and K. Tsubaki, J. Am. Chem. Soc., 2014, 136, 7101–7109 CrossRef PubMed
; K. Nakanishi, T. Sasamori, K. Kuramochi, N. Tokitoh, T. Kawabata and K. Tsubaki, J. Org. Chem., 2014, 79, 2625–2631 CrossRef
.
Footnote |
† Electronic supplementary information (ESI) available: Detailed experimental procedures, characterization of molecules and additional spectroscopic computational data. CCDC 1907392 and 1907393. For ESI and crystallographic data in CIF or other electronic format see DOI: 10.1039/c9sc01777a |
|
This journal is © The Royal Society of Chemistry 2019 |
Click here to see how this site uses Cookies. View our privacy policy here.