DOI:
10.1039/C9SC02823D
(Perspective)
Chem. Sci., 2019,
10, 7670-7679
Frontiers and progress in cation-uptake and exchange chemistry of polyoxometalate-based compounds
Received
10th June 2019
, Accepted 24th July 2019
First published on 25th July 2019
Abstract
Cation-uptake and exchange has been an important topic in both basic and applied chemistry relevant to life and materials science. For example, living cells contain appreciable amounts of Na+ and K+, and their concentrations are regulated by the sodium–potassium pump. Solid-state cation-exchangers such as clays and zeolites both natural and synthetic have been used widely in water softening and purification, separation of metal ions and biomolecules, etc. Polyoxometalates (POMs) are robust, discrete, and structurally well-defined metal-oxide cluster anions, and have stimulated research in broad fields of sciences. In this perspective, cation-uptake and exchange in POM and POM-based compounds are categorized and reviewed in three groups: (i) POMs as inorganic crown ethers and cryptands, (ii) POM-based ionic solids as cation-exchangers, and (iii) reduction-induced cation-uptake in POM-based ionic solids, which is based on a feature of POMs that they are redox-active and multi-electron transfer occurs reversibly in multiple steps. This method can be utilized to synthesize mixed-valence metal clusters in metal ion-exchanged POM-based ionic solids.
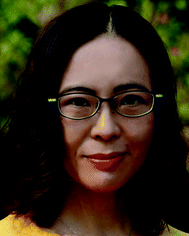 Sayaka Uchida | Sayaka Uchida is an associate professor at the Department of Basic Science, The University of Tokyo (Japan). She received her PhD in applied chemistry from The University of Tokyo (2002). She has worked as a postdoc and assistant professor at the Department of Applied Chemistry, The University of Tokyo (Prof. Noritaka Mizuno group) and moved to the current position (2009 Oct). She has also worked as a JST-PRESTO researcher “Hyper-nanospace design toward innovative functionality” (2013–2017). Her work focuses on the synthesis of functional solid-state materials based on polyoxometalates. |
1. Introduction
Cation-uptake and exchange from aqueous solutions has been an important topic in both basic and applied chemistry relevant to life and materials science.1–3 For example, living cells contain appreciable amounts of Na+ and K+, and their concentrations are regulated by the sodium–potassium pump, which exchanges three Na+ with two K+.3 On the other hand, it is quite difficult to achieve high selectivity towards K+ artificially except for 18-crown-6 ether,4 which is a cyclic oligomer of ethylene oxide, and binds K+ by using all six oxygens as donor atoms. The denticity of the polyether influences the affinity toward various ions: 15-crown-5 and 12-crown-4 show high selectivity toward Na+ and Li+, respectively. Crown ethers have been widely used for cation recognition and separation, and as phase transfer catalysts.5
Solid-state cation-exchangers play an especially important role in chemistry. A classic example is zeolites, which are microporous crystalline aluminosilicates with anionic frameworks due to the substitution of Si4+ by Al3+.6 Cations such as Na+, K+, Ca2+, Mg2+, etc. loosely interact with the anionic framework via Coulomb interaction, which can be exchanged by treating the zeolite in an aqueous solution containing excess amount of foreign cations. It is well known that the pore sizes and adsorption properties of zeolites can be controlled by the types of cations: the effective pore size of Linde Type A (LTA) zeolite with K+ is 3 Å (Molecular Sieves 3A), and the pore size is increased to 4 Å by the exchange of K+ with smaller Na+ (Molecular Sieves 4A).7 Zeolites can adsorb gas and vapor (CH4, H2O, etc.) in the microporous structure, and the amounts of adsorption in alkaline earth metal ion-exchanged and alkali metal ion-exchanged faujasite (FAU) zeolites increase with the increase in the ionic potentials z/r (z and r are the charge and radius of the ion, respectively) of the counter cations.8,9
Recently, because of relatively facile reaction conditions, cation-exchange has also been recognized as a strategy for post-synthesis and discovery of new solid materials.2 Metal–organic frameworks (MOFs), which can be recognized as “inorganic–organic zeolites” have emerged decades ago, but cation-exchange has been reported only recently. A landmark report in this research area is the exchange of guest Mn2+ in as-synthesized Mn3[(Mn4Cl3)(BTT)8(CH3OH)10]2 (BTT = 1,3,5-benzenetristetrazolate) with monovalent or divalent metal ions in methanol solution, which resulted in the formation of isostructural frameworks with a large variation in H2 adsorption enthalpy.10,11 Cation-exchange has been also employed with nanocrystals and nanoparticles to fine-tune their structures and functions systematically.12 For example, in vivo cation-exchange of Ag+ with Hg2+ and Zn2+ in selenide/sulfide quantum dots enhanced the specificity of tumor imaging.13
Polyoxometalates (POMs) are robust, discrete, and structurally well-defined oxide cluster anions that are mainly composed of high-valence transition metals (such as W6+, Mo6+, V5+) and have stimulated research in broad fields of sciences.14–25 For example, α-Keggin-type silicododecatungstate, which is one of the most researched and popular POM, forms according to the following equation:
| SiO44− + 12WO42− + 24H+ → [α-SiW12O40]4− + 12H2O. | (1) |
The oxides of high-valence transition metals dissolve at high pH as an anion (e.g., WO42−), condensation proceeds via loss of water and formation of M–O–M linkages with acidification, and an anionic molecular framework of twelve octahedral tungsten oxoanions surrounding a central silicate is formed. One of the most noteworthy features of POMs are that they are redox-active, and multi-electron transfer occurs reversibly in multiple-steps:16
| [α-SiWVI12O40]4− + e− = [α-SiWVI11WVO40]5− (−0.22 V vs. SHE in 1 M HCl(aq.)), | (2) |
| [α-SiWVI11WVO40]5− + e− = [α-SiWVI10WV2O40]6− (−0.42 V vs. SHE in 1 M HCl(aq.)). | (3) |
In this perspective, cation-uptake and exchange in POM and POM-based compounds are categorized and reviewed in three groups: (i) POMs as inorganic crown ethers and cryptands, (ii) POM-based ionic solids as cation-exchangers, and (iii) reduction-induced cation-uptake in POM-based ionic solids (Fig. 1). Unique functions related to these cation-exchanged POM-based compounds are introduced, and future works arising from these functions are also discussed. For past developments on polyoxometalates as cation-exchangers, the readers are directed to a legendary review article.26
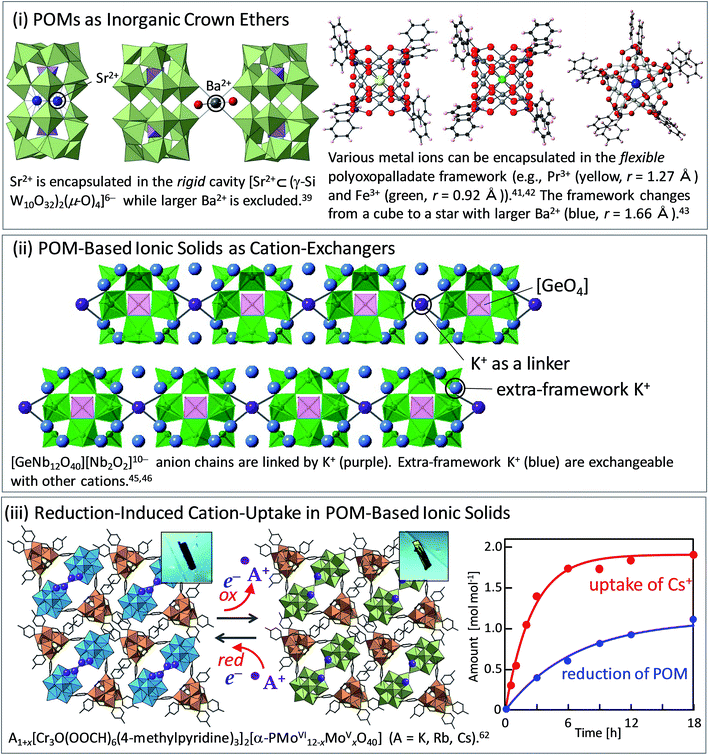 |
| Fig. 1 Cation-uptake and exchange in POM and POM-based compounds categorized in three groups: (i) POMs as inorganic crown ethers, (ii) POM-based ionic solids as cation-exchangers, and (iii) reduction-induced cation-uptake in POM-based ionic solids. | |
2. Polyoxometalates as inorganic crown ethers and cryptands
Crown ethers4,5 and cryptands,27,28 which are a family of synthetic cyclic and polycyclic multidentate organic ligands, have attracted great interest due to their structural topologies and applications especially in selective cation-uptake. Crown ethers can strongly bind alkali and alkali earth metal ions size-selectively with the oxygen donors in gas, solution, or solid phases. Cryptands can bind these cations using both nitrogen and oxygen donors three-dimensionally, often showing higher selectivity and binding constants.
In contrast, POMs can serve as inorganic crown ethers and cryptands:29 an early example is a cyclic POM [As4W40O140]28−, which binds alkali and alkaline earth metal ions selectively within the central “cryptand site” (best fit for K+ and Ba2+, which are hard Lewis acids) while transition metals (soft Lewis acids) bind to the “external site” with a different type of coordination.30 A recent work by Kortz and co-workers on a wheel-shaped K+-templated POM [K⊂{(β-AsIIIW8O30)(WO(H2O))}3]14− exhibits high selectivity to Rb+, because the size of the central cavity is relatively large for K+.31 Preyssler–Pope–Jeannin-type POM with a general formula of [Xn+(H2O)P5W30O110](15−n)− is the smallest POM with an internal cavity allowing cation-exchange in aqueous solutions.32,33 Preyssler–Pope–Jeannin-type POM possesses a flexible W5O5 cavity and can capture various cations from Na+, Ca2+, La3+ to tetravalent actinides (e.g., Th4+) (Fig. 2),34 so that they have been considered as a potentially useful material for separation of nuclear wastes. DFT calculations by López, Poblet, and co-workers have revealed that encapsulation of cations with larger charge is difficult (i.e., heating is needed) because energy cost for the cation excapsulation from aqueous solution is dependent on the dehydration enthalpy of the cation.34 A more recent report by Li, Su, Wang and co-workers shows that while Preyssler–Pope–Jeannin-type POMs with phosphorous [P5W30O110]15− exhibit high affinity to Na+, those with sulfur [S5W30O110]10− exhibit high affinity to K+ because of the larger internal cavity.35
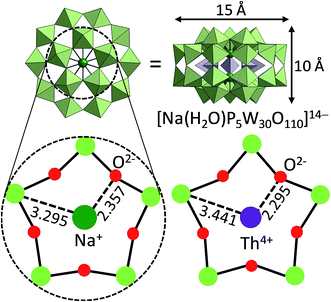 |
| Fig. 2 Preyssler–Pope–Jeannin-type POM [Xn+(H2O)P5W30O110](15−n)− with flexible W5O5 cavity for cation encapsulation.33 Light green and purple polyhedra show the [WO6] and [PO4] units, respectively. | |
Müller and co-workers synthesized a series of nanoporous POM capsules with a general formula of [{(MoVI)MoVI5O21(H2O)6}12{MoV2O4(ligand)}30], and these capsules allow systematic studies of uptake/release of cations in aqueous solutions.36,37 The capsules possess large negative charges, and the affinity and coordination environment for cations depend on the functional groups inside the capsules. For example, various cations can coordinate to SO42− ligands via exchange with NH4+: protonated urea molecules situate close to the pore openings while Ce3+ situates deeply inside the capsule due to the small ionic radius (Fig. 3).36,37 The protonated urea molecules can be removed and the pores open by cation-exchange with Ca2+ in water.38 This system can be recognized as an artificial cell since Ca2+ take an important role in life science.
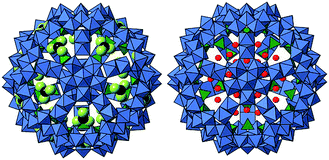 |
| Fig. 3 Coordination environments of protonated urea (left, black: C and yellow: N/O) and Ce3+ (right, red) in the nanoporous POM capsule.36,37 Blue and green polyhedra show the [MO6] and [SO4] units, respectively. | |
Mizuno and co-workers synthesized a dimeric POM [Hn(γ-SiW10O32)2(μ-O)4](8−n)− by dehydrative condensation of silicodecatungstate [γ-SiW10O34(H2O)2]4−.39 The dimeric POM has a rigid cavity between the two silicodecatungstate units, where divalent Pb2+ and Sr2+ and monovalent Ag+, Na+, K+, and Rb+ can be encapsulated, while larger Ba2+ and Cs+ are completely excluded (Fig. 1). Interestingly, this POM, which can be considered as an inorganic cryptand, can capture Sr2+ more strongly than the organic counterpart. Baskar, Winpenny, and co-workers have reported that a ε-Keggin-type POM constructed with [SbO6] units can accommodate d5 and d10 ions such as Mn2+ and Zn2+ at the center cage of the molecule in a tetrahedral fashion.40
Kortz and co-workers have reported that a polyoxopalladate [MPdII12(AsPh)8O32]n− can accommodate various lanthanide ions (M = Pr3+, Nd3+, Sm3+, Eu3+, Gd3+, Tb3+, Dy3+, Ho3+, Er3+, Tm3+, Yb3+, Lu3+) as well as 3d transition metal ions (M = Sc3+, Mn2+, Fe3+, Co2+, Cu2+, Zn2+) at the cuboidal center cage of the molecule.41,42 The [Pd12O32] unit is flexible and can adjust to the coordination requirements of a large variety of metal ions (Fig. 1).41,42 More recently, they have reported that alkaline earth metal ions (Ca2+, Sr2+, Ba2+) with relatively large ionic radii as templates affect the polyoxopalladate framework so that the nanocube (8-coordination) transforms to a nanostar (10-coordination).43
3. Polyoxometalate-based ionic solids as cation-exchangers
Ammonium salt of α-Keggin-type phosphododecamolybdate (NH4)3[α-PMo12O40] has been long investigated as a cation-exchanger in aqueous solutions according to the following equation, | (NH4)3[α-PMo12O40] + 3A+ ⇄ A3[α-PMo12O40] + 3NH4+. | (4) |
The following affinity was derived Cs+ = Tl+ > Rb+ > Ag+ > K+ > H3O+ > Na+ > Li+, which is in line with the trend in hydration radius or dehydration enthalpy of the cations.26,44 This trend means that it is more facile to remove the water of hydration from Cs+ than Li+ because of the large ionic radius (i.e., low ionic potential) of Cs+, so that Cs+ can more easily enter and diffuse through the solid state structure. Besides, [α-PMo12O40]3− shows high affinity towards Tl+ or Ag+, and the bonds between Tl+ or Ag+ and O2− of the POM are supposed to have a covalent character.
The selectivity, kinetics, and capacity of cation-exchange in POM-based ionic solids are determined both by the framework geometry and the behavior of extra-framework cations. For example, Nyman and co-workers reported that Keggin-type polyoxoniobates [XNb12O40]n− (X = Si, Ge, P) with [Ti2O2]4+ or [Nb2O2]6+ bridges form one-dimensional chains, and these chains have an overall negative charge of −10 or −12.45 Single crystal X-ray diffraction, thermogravimetry, IR, and 1H-MASNMR combined with computational studies could distinguish the states of counter cations (Na+ and K+), and the mobile extra-framework cations can be exchanged with radionuclides (Sr2+, Np(NpO2+), and Pu4+) (Fig. 1).46 Unlike with conventional POMs, polyoxoniobates are stable under basic conditions, and therefore should be less likely to decompose in the highly alkaline conditions of nuclear wastes. More recently, Ueda, Sadakane, and co-workers synthesized microporous solids with ε-Keggin-type POMs and Zn2+ or Mn2+ as linkers (Fig. 4).47 These solids possess 3D-cages and channels with an aperture of ca. 8 Å containing exchangeable cations (NH4+ and Na+). These cations can be exchanged with K+, Rb+, and Cs+ in aqueous solutions, while exchange with H+ and Li+ is insufficient,47 which is in line with the hydration radius and dehydration enthalpy of the cations.44 More recently, the same group has reported the synthesis and structure of a polyoxomolybdotellurate with a one-dimensional molecular structure.48 NH4+ as counter cations surround the molecular wire, and NH4+ is selectively exchanged with Cs+ among alkali metal ions in water, and large alkylammonium cations can also be incorporated due to the flexible solid-state structure.49
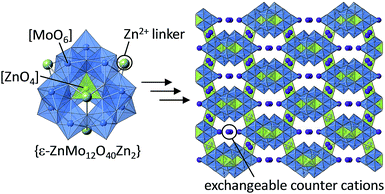 |
| Fig. 4 Microporous solid composed of {ε-ZnMo12O40Zn2} units and exchangeable counter cations.47 | |
Acidic salts of α-Keggin-type POM (H3[α-PW12O40]·nH2O, H4[α-SiW12O40]·nH2O) have been well known as excellent acid catalysts, and partial substitution of protons with Cs+ in aqueous solutions, stabilizes the solid-state structure and increases the surface area.50–52 The cesium hydrogen salt of silicododecatungstate CsxH4−x[α-SiW12O40] adopts a body-centered cubic cell in analogy to the cesium salt of phosphododecatungstate Cs3[α-PW12O40].53 We have shown that the use of [α-SiW12O40]4− instead of [α-PW12O40]3− leads to the formation of POM vacancies to compensate the excess negative charge, which give rise to channels exhibiting cation-exchange of Cs+ with other alkali metal ions in aqueous solutions (Fig. 5a and b).54 Amounts of cation-exchange decreased in the order of Rb+ > K+ > Na+ > Li+, which is in line with the hydration radius and dehydration enthalpy of the alkali metal ions44 (Fig. 5c), and elemental mapping images confirmed the uniform distribution of the exchanged cations (Fig. 5d).54 Recently, Sun and co-workers showed that the cation-exchange of Cs+ in the cesium hydrogen salt of silicododecatungstate with Bi (BiO+ and BiOH2+) having stereoactive 6s2 lone pair as a dopant, leads to near-infrared photoluminescence in the important biological and telecommunication optical windows, due to the asymmetric coordination geometry of the Bi species in the microporous framework.55 This result offers a new strategy for the preparation of POM-based luminescent systems via cation-exchange.
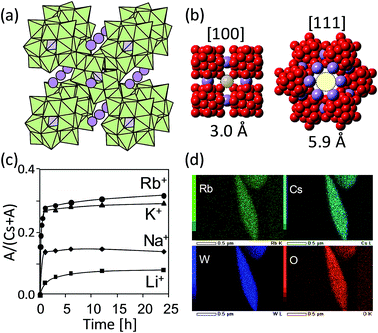 |
| Fig. 5 (a) Crystal structure of CsxH4−x[α-SiW12O40]. Light green and purple polyhedra show the [WO6] and [SiO4] units, respectively. Purple spheres are exchangeable cations (Cs+). (b) Anion vacancies (in light yellow) along the [100] and [111] directions. Red and purple spheres show the O2− of POM and Cs+, respectively. (c) Cation-exchange: time courses of uptakes of alkali metal ions. (d) Elemental mapping images.54 | |
We have reported a porous organic–inorganic ionic crystal K2[Cr3O(OOCH)6(4-methylpyridine)3]2[α-SiW12O40]·nH2O composed of [α-SiW12O40]4− with a molecular cation [Cr3O(OOCH)6(4-methylpyridine)3]+, which possess robust one-dimensional channels due to π–π stacking among the 4-methylpyridine of the neighboring cations.56 Cr(III)-carboxylates with a general formula of [Cr3O(OOCR)6(L)3]+ have been widely considered as building blocks of porous solids because of the versatile selection of bridging (R) and terminal (L) ligands and chemical inertness due to the large crystal field stabilization energy of Cr3+ with d3 configuration.57 The counter cation (K+) can be fully-exchanged with other alkali metal cations in aqueous solutions, and the states of water molecules in the channels can be controlled by the type of alkali metal ions:58 alkali metal ions with high ionic potentials (e.g., Li+) form dense and extensive hydrogen-bonding network of water molecules with mobile protons at the periphery, which leads to high proton conductivities of 10−3 S cm−1 without any acidic functional groups (Fig. 6).58
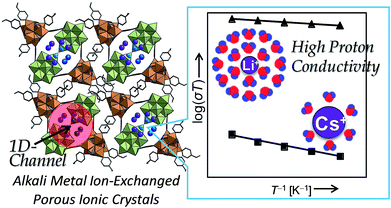 |
| Fig. 6 (Left) Crystal structure of K2[Cr3O(OOCH)6(4-methylpyridine)3]2[α-SiW12O40]·nH2O. Light green and orange polyhedra show the [WO6] and [CrO5N] units, respectively. Purple and blue spheres show the alkali metal ions and oxygen atoms of the water of crystallization, respectively. (Right) Arrhenius plots of the temperature dependent proton conductivities at RH 95% (303–323 K). Proton conductivities of the compound with Li+ and Cs+ as counter cations were 1.9 × 10−3 and 1.2 × 10−7 S cm−1, respectively (323 K).58 | |
4. Reduction-induced cation-uptake in polyoxometalate-based ionic solids
Redox property of solids is a key for selective cation-uptake and exchange relevant to material science. For example, Yoshikawa, Awaga, and co-workers have reported that α-Keggin-type phosphododecamolybdate [α-PMo12O40]3− exhibits reversible 24-electron redox during charging/discharging due to the twelve molybdenum atoms (MoIV/VI) coupled with Li+ uptake/release, as a component of molecular cluster battery.59 Therefore, it can be suggested that one of the best way to engineer redox-active porous solids would be to incorporate redox-active components. A landmark example was reported by Cronin and co-workers: a porous solid composed of silicodecatungstate [γ-SiW10O36]8− and Mn3+ was synthesized, and the oxidation states of MnII/III can be switched by the addition of reducing/oxidizing reagents.60 They have later synthesized another redox-active porous solid with cyclic POM [P8W48O148]40− units and MnII/III (Fig. 7), and the cation-exchange rate and capacity can be controlled by the oxidation states of Mn.61
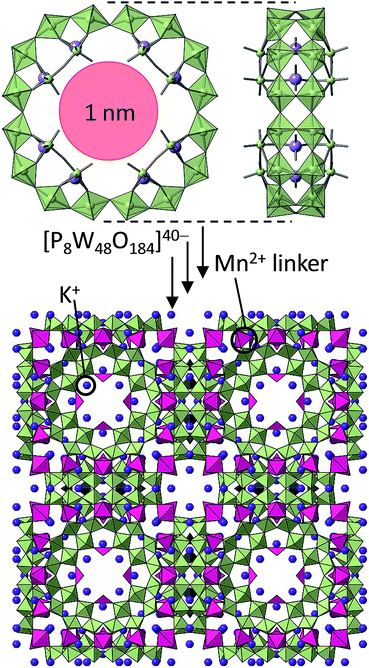 |
| Fig. 7 (Upper) Molecular structure of [P8W48O184]40− comprising a nanometer-size cavity. (Lower) The molecular unit is linked by Mn2+ resulting in a 3D-POM framework.61 | |
We have reported a redox-active porous ionic crystal A[Cr3O(OOCH)6(4-methylpyridine)3]2[α-PMo12O40]·nH2O (A = alkali metal ions) possessing one-dimensional channels, and the treatment of the crystal with reducing (ascorbic acid) or oxidizing (chlorine water) reagents results in one-electron redox of the POM (MoV/VI) coupled with uptake/release of alkali metal ions, and the reaction rate depended on the type of alkali metal ions.62 The reaction rate increased in the order of K+ < Rb+ < Cs+, which is in line with the order of hydration radius and dehydration enthalpy of the cations (Fig. 1).44,62 This work was extended by the utilization of [α-SiMo12O40]4−, which resulted in the formation of an ionic crystal with isolated pores instead of continuous one-dimensional ones (Fig. 8).63 The compound selectively adsorbed Cs+ among alkali and alkaline earth metal ions via reduction of the POM in the compound with ascorbic acid, showing potential applicability as an adsorbent for radioactive Cs+ removal from environmental water. Despite the high selectivity to Cs+, there were several tasks to solve: requirement of heating (343 K) and slow adsorption kinetics (12 h to reach equilibrium). In order to solve these tasks, large-molecular size and easily reducible Wells–Dawson-type POMs [α-P2M18O62]6− (M = Mo, W) were utilized to increase the pore volume and to facilitate the reduction-induced Cs+ uptake.64 As expected, Cs+-uptake capacity and rate increased largely (only 1 h to reach equilibrium at room temperature).
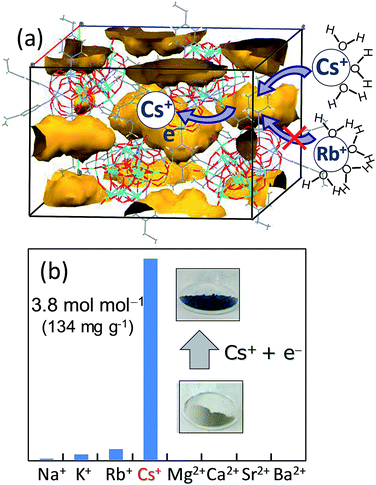 |
| Fig. 8 (a) Crystal structure of (etpyH)2[Cr3O(OOCH)6(etpy)3]2[α-SiMo12O40]·nH2O (etpy = 4-ethylpyridine). Each void (in yellow-brown) has a size of ca. 6.5 Å × 12.5 Å. (b) Amounts of cations incorporated by the reduction-induced method. Note that there is a color change due to the reduction of POM upon Cs+ uptake.63 | |
Metal clusters are a topic of great interest in materials science and have found numerous applications especially in catalysis and electro-optics.65 Microporous compounds offer versatile scaffolds for the formation and stabilization of metal clusters from metal ions. For example, small mixed-valence silver clusters have been synthesized in zeolites by calcination of Ag+-exchanged zeolites at high temperature: Ag42+ in MFI-zeolite66 is active for the selective reduction of NO by propane with O2 and H2, and Ag42+ in LTA-zeolite67 shows on–off switching of yellow-green photoluminescence (PL) by hydration–dehydration. A landmark report on formation of metal clusters in redox-active MOFs has been carried out by reducing Pd2+via electron transfer from nitrilotrisbenzoate, which is a redox-active organic linker of the porous framework.68 However, redox of MOFs is mostly limited to the utilization of redox-active organic ligands because redox of the metal center ion induces large change in the coordination geometry causing to collapse the porous framework. Therefore, metal clusters in MOFs have been synthesized by adding reducing reagents such as H2, NaBH4, DMF, etc.69–71 to the MOF comprising metal ions, and homogeneous formation and distribution of metal clusters become a problem.
As explained above, POMs can store multiple electrons in the molecular framework and have been utilized as constituents of redox-active porous frameworks. Some compounds show cooperative migration of electrons with metal ions,61–64 so called cation-coupled electron-transfer (CCET) in relation to proton-coupled electron-transfer72 (PCET). Quite recently, we have utilized redox-active porous ionic crystals Cs[Cr3O(OOCH)6(4-methylpyridine)3]2[α-PMoVI12O40]·nH2O (Cs-ox) and Cs2[Cr3O(OOCH)6(4-methylpyridine)3]2[α-PMoVI11MoVO40]·nH2O (Cs2-red) (the abbreviations Cs-ox and Cs2-red are based on the types and numbers of counter cations and the oxidation state of POM), to form and stabilize small mixed-valence luminescent silver clusters in the one-dimensional channel (Fig. 9). According to elemental analysis of cesium and silver in the compounds by atomic absorption spectrometry (AAS), we have found that reduction-induced ion-exchange of Cs+ in Cs2-red with Ag+ from AgNO3(aq.), and subsequent formation of a mixed-valence luminescent silver cluster Ag42+ took <1 min (eqn (5)), while the simple ion-exchange with Cs+ in Cs-ox with Ag+ from AgNO3(aq.) took >24 h (eqn (6)):73
| Cs2[Cr3O(OOCH)6(4-methylpyridine)3]2[α-PMoVI11MoVO40] + 2Ag+ → AgIAg0[Cr3O(OOCH)6(4-methylpyridine)3]2[α-PMoVI12O40] + 2Cs+, | (5) |
| Cs[Cr3O(OOCH)6(4-methylpyridine)3]2[α-PMoVI12O40] + Ag+ → AgI[Cr3O(OOCH)6(4-methylpyridine)3]2[α-PMoVI12O40] + Cs+. | (6) |
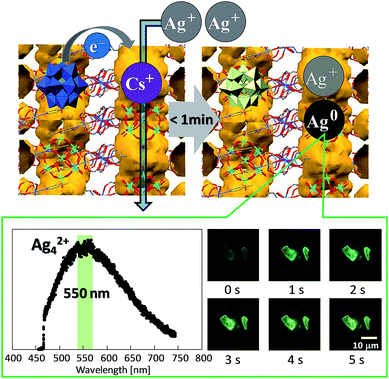 |
| Fig. 9 Schematic illustration of the reduction-induced ion-exchange of Cs+ with Ag+ in the one-dimensional channel (in yellow-brown) to form small mixed-valence luminescent silver clusters. PL spectrum and time course of PL images of a single crystal of Cs2-red in AgNO3(aq.) (excitation at 405 nm).73 | |
Note that the formation of Ag42+ in the redox-active porous ionic crystals was assumed according to the report that Ag42+ in LTA-zeolite emits yellow-green light giving a broad emission band around 550 nm.67 According to the PL images in Fig. 9, Ag42+ is formed within few seconds. A model fitting of the time course of PL intensity has shown that the reduction-induced ion-exchange consists of two steps: electron transfer from the reduced POM ([α-PMoVI11MoVO40]4−) to Ag+ and subsequent formation of a silver cluster Ag42+, and diffusion of the silver cluster and exchange with Cs+. The compound containing the silver cluster showed high affinity toward unsaturated hydrocarbon guests (acetylene and ethylene). Ag/Al2O3 has been commercialized as a catalyst for the epoxidation of ethylene and propylene, and small mixed-valence silver clusters have been suggested as the activation site of this reaction.74 Our next aim is to form and stabilize these clusters in redox-active ionic crystals with mesopores, and to apply them as solid catalysts. In addition, synthesis of metal clusters with different elements, sizes, and charges by controlling the pore size and degree of reduction of the POM-based redox-active scaffold is currently under investigation.
5. Summary and outlook
In this perspective, cation-uptake and exchange in POMs and POM-based compounds were categorized and reviewed in three groups. (i) POMs as inorganic crown ethers and cryptands: POMs can offer versatile platforms for cation coordination, which are different from those of the organic counterparts, and one of the next targets would be to substitute oxygen (O2−) of POMs with sulfur (S2−), selenium (Se2−), etc.75 to tune the coordination environment. (ii) POM-based ionic solids as cation-exchangers: the next target would be to explore cooperative effects of the selectively adsorbed cations and POMs,55 especially as optical materials, magnetic materials, solid catalysts, etc. (iii) Reduction-induced cation-uptake in POM-based ionic solids: while it is difficult for conventional porous compounds such as zeolites and MOFs to support the geometry change in the framework that often accompany the redox processes, POM-based solids show great potential for the multiple and reversible uptake/release of cations with electrons.60–64,73 Such CCET reactions in solids can be applied not only to selective cation-uptake and sensing but also to the next-generation rechargeable batteries,76 solid catalysts for water splitting, chemical fixation of CO2, ammonia synthesis, etc.
Another challenge is anion-exchange in POM-based compounds. This notion includes substitution of O2− in POMs with S2−, Se2−, N3− or halide ions as well as incorporation of multiple types of anions in the ionic solid. A recent review on metal oxyfluorides and oxynitrides show that incorporation of multiple anions in metal oxide-based compounds can finely modulate physicochemical properties such as catalysis, optics, conduction, magnetism, etc.77 Some MOFs with cationic frameworks show anion-exchange properties,78 according to the Hofmeister series79 (citrate (trivalent) > sulfate (divalent) > acetate (monovalent) > HCO3− > Cl− > Br− > I− > NO3−, which is in line with the degree of hydration) or non-Hofmeister selectivity due to the utilization of Lewis acid and/or multidentate donors.80 We have recently reported the synthesis of cesium salts of α-Keggin-type [α-BW12O40]5− (BW) and [α-SiW12O40]4− (SiW) blends, and the porosity is finely controlled by the BW/SiW ratio.81 The next aim would be to synthesize these mixed-POM compounds post-synthetically or by anion-exchange.
Conflicts of interest
There are no conflicts to declare.
Acknowledgements
This work was supported by JST-PRESTO (JPMJPR1312) and grant-in-aids for scientific research JP16K05742, JP19H04564 (Coordination Asymmetry), and JP19H04686 (Mixed Anion) from MEXT of Japan. Prof. N. Mizuno (Univ. of Tokyo) is acknowledged for his continuous encouragement and support on this research. Collaborators, and former and current students of Uchida group are acknowledged for their contribution on this research.
Notes and references
- A. Clearfield, Role of ion exchange in solid-state chemistry, Chem. Rev., 1988, 88, 125–148 CrossRef CAS.
- J. B. Rivest and P. K. Jain, Cation exchange on the nanoscale: an emerging technique for new material synthesis, device fabrication, and chemical sensing, Chem. Soc. Rev., 2013, 42, 89–96 RSC.
- J. P. Morth, B. P. Pedersen, M. S. Toustrup-Jensen, T. L. M. Sørensen, J. Petersen, J. P. Andersen, B. Vilsen and P. Nissen, Crystal structure of the sodium–potassium pump, Nature, 2007, 450, 1043–1049 CrossRef CAS PubMed.
- C. J. Pedersen, Cyclic polyethers and their complexes with metal salts, J. Am. Chem. Soc., 1967, 89, 7017–7036 CrossRef CAS.
- G. W. Gokel, W. M. Leevy and M. E. Weber, Crown ethers: Sensors for ions and molecular scaffolds for materials and biological models, Chem. Rev., 2004, 104, 2723–2750 CrossRef CAS PubMed.
- M. E. Davis and R. F. Lobo, Zeolite and Molecular Sieve Synthesis, Chem. Mater., 1992, 4, 756–768 CrossRef CAS.
- D. W. Breck, W. G. Eversole, R. M. Milton, T. B. Reed and T. L. Thomas, Crystalline zeolites. I. The properties of a new synthetic zeolite, type A, J. Am. Chem. Soc., 1956, 78, 5963–5972 CrossRef CAS.
- O. Talu, S. Y. Zhang and D. T. Hayhurst, Effect of cations on methane adsorption by NaY, MgY, CaY, SrY, and BaY zeolites, J. Phys. Chem., 1993, 97, 12894–12898 CrossRef CAS.
- O. M. Dzhigit, A. V. Kiselev, K. N. Mikos, G. G. Muttik and T. A. Rahmanova, Heats of adsorption of water vapour on X-zeolites containing Li+, Na+, K+, Rb+, and Cs+ cations, Trans. Faraday Soc., 1971, 67, 458–467 RSC.
- M. Dincă and J. R. Long, High-enthalpy hydrogen adsorption in cation-exchanged variants of the microporous metal–organic framework Mn3[(Mn4Cl)3(BTT)8(CH3OH)10]2, J. Am. Chem. Soc., 2007, 129, 11172–11176 CrossRef PubMed.
- C. K. Brozek and M. Dincă, Cation exchange at the secondary building units of metal–organic frameworks, Chem. Soc. Rev., 2014, 43, 5456–5467 RSC.
- B. J. Beberwyck, Y. Surendranath and A. P. Alivisatos, Cation Exchange: A versatile tool for nanomaterials synthesis, J. Phys. Chem. C, 2013, 117, 19759–19770 CrossRef CAS.
- X. Liu, G. B. Braun, M. Qin, E. Ruoslahti and K. N. Sugahara, In vivo cation exchange in quantum dots for tumor-specific imaging, Nat. Commun., 2017, 8, 343 CrossRef PubMed.
-
M. T. Pope, Heteropoly and Isopoly Oxometalates, Springer-Verlag, New York, 1983 Search PubMed.
- M. T. Pope and A. Müller, Polyoxometalate chemistry: An old field with new dimensions in several disciplines, Angew. Chem., Int. Ed. Engl., 1991, 30, 34–48 CrossRef.
- C. L. Hill, Thematic issue on polyoxometalate, Chem. Rev., 1998, 98, 1–390 CrossRef CAS PubMed.
- L. Cronin and A. Müller, From serendipity to design of polyoxometalates at the nanoscale, aesthetic beauty and applications, Chem. Soc. Rev., 2012, 41, 7325–7648 RSC.
- E. Coronado, C. Giménez-Saiz and C. J. Gómez-García, Recent advances in polyoxometalate-containing molecular conductors, Coord. Chem. Rev., 2005, 249, 1776–1796 CrossRef CAS.
- A. Proust, R. Thouvenot and P. Gouzerh, Functionalization of polyoxometalates: towards advanced applications in catalysis and materials science, Chem. Commun., 2008, 1837–1852 RSC.
- D. Li, P. Yin and T. Liu, Supramolecular architectures assembled from amphiphilic hybrid polyoxometalates, Dalton Trans., 2012, 41, 2853–2861 RSC.
- K. Y. Monakhov, W. Bensch and P. Kögerler, Semimetal-functionalized polyoxovanadates, Chem. Soc. Rev., 2015, 44, 8443–8483 RSC.
- K. Suzuki, N. Mizuno and K. Yamaguchi, Polyoxometalate photocatalysis for liquid-phase selective organic functional group transformations, ACS Catal., 2018, 8, 10809–10825 CrossRef CAS.
- P. Yang and U. Kortz, Discovery and evolution of polyoxopalladates, Acc. Chem. Res., 2018, 51, 1599–1608 CrossRef CAS.
- I. A. Weinstock, R. E. Schreiber and R. Neumann, Dioxygen in polyoxometalate mediated reactions, Chem. Rev., 2018, 118, 2680–2717 CrossRef CAS PubMed.
- L. Chen, W.-L. Chen, X.-L. Wang, Y.-G. Li, Z.-M. Su and E.-B. Wang, Polyoxometalates in dye-sensitized solar cells, Chem. Soc. Rev., 2019, 48, 260–284 RSC.
- V. Pekárek and V. Veselý, Synthetic inorganic ion exchangers-II: Salts of heteropolyacids, insoluble ferrocyanides, synthetic aluminosilicates and miscellaneous exchangers, Talanta, 1972, 19, 1245–1283 CrossRef.
- B. Dietrich, J. M. Lehn and J. P. Sauvage, Diaza-polyoxa-macrocycles et macrobicycles, Tetrahedron Lett., 1969, 34, 2885–2889 CrossRef.
- B. Dietrich, J. M. Lehn and J. P. Sauvage, Les cryptates, Tetrahedron Lett., 1969, 34, 2889–2892 CrossRef.
- W. G. Klemperer and G. Westwood, Traps for cations, Nat. Mater., 2003, 2, 780–781 CrossRef CAS PubMed.
- F. Robert, M. Leyrie, G. Hervé, A. Tézé and Y. Jeannin, Crystal structure of ammonium dicobalto(II)-40-tungstotetraarsenate(III). Allosteric effects in the ligand, Inorg. Chem., 1980, 19, 1746–1752 CrossRef CAS.
- B. Kandasamy, T. Sudmeier, W. W. Ayass, Z. Lin, Q. Feng, B. S. Bassil and U. Kortz, Selective Rb+ vs. K+ guest incorporation in wheel-shaped 27-tungsto-3-arsenate(III) host, [M⊂{(β-AsIIIW8O30)(WO(H2O))}3]14− (M = K, Rb), Eur. J. Inorg. Chem., 2019, 502–505 CrossRef CAS.
- M. H. Alizadeh, S. P. Harmalker, Y. Jeannin, J. Martin-Frere and M. T. Pope, A heteropolyanion with fivefold molecular symmetry that contains a nonlabile encapsulated sodium ion. The structure and chemistry of [NaP5W30O110]14−, J. Am. Chem. Soc., 1985, 107, 2662–2669 CrossRef CAS.
- I. Greaser, M. C. Heckel, R. J. Neitz and M. T. Pope, Rigid nonlabile polyoxometalate cryptates [ZP5W30O110](15−n)− that exhibit unprecedented selectivity for certain lanthanide and other multivalent cations, Inorg. Chem., 1993, 32, 1573–1578 CrossRef.
- J. A. Fernández, X. López, C. Bo, C. de Graaf, E. J. Baerends and J. M. Poblet, Polyoxometalates with internal cavities: redox activity, basicity and cation encapsulation in [Xn+P5W30O110](15−n)− Preyssler Complexes, with X = Na+, Ca2+, Y3+, La3+, Ce3+, and Th4+, J. Am. Chem. Soc., 2007, 129, 12244–12253 CrossRef.
- Z.-M. Zhang, S. Yao, Y.-G. Li, X.-B. Han, Z.-M. Su, Z.-S. Wang and E.-B. Wang, Inorganic crown ethers: Sulfate-based Preyssler polyoxometalates, Chem.–Eur. J., 2012, 18, 9184–9188 CrossRef CAS.
- A. Müller, S. K. Das, S. Talismanov, S. Roy, E. Beckmann, H. Bögge, M. Schmidtmann, A. Merca, A. Berkle, L. Allouche, Y. Zhou and L. Zhang, Trapping cations in specific positions in tunable “artificial cell” channels: New nanochemistry perspectives, Angew. Chem., Int. Ed., 2003, 42, 5039–5044 CrossRef PubMed.
- A. Müller, D. Rehder, E. T. K. Haupt, A. Merca, H. Bögge, M. Schmidtmann and G. Heinze-Brückner, Artificial cells: Temperature-dependent, reversible Li+-ion uptake/release equilibrium at metal oxide nanocontainer pores, Angew. Chem., Int. Ed., 2004, 43, 4466–4470 CrossRef PubMed.
- A. Müller, L. Toma, H. Bögge, C. Schäffer and A. Stammler, Porous capsules
allow pore opening and closing that results in cation uptake, Angew. Chem., Int. Ed., 2005, 44, 7757–7761 CrossRef PubMed.
- A. Yoshida, Y. Nakagawa, K. Uehara, S. Hikichi and N. Mizuno, Inorganic cryptand: Size-selective strong metallic cation encapsulation by a disilicoicosatungstate (Si2W20) polyoxometalate, Angew. Chem., Int. Ed., 2009, 48, 7055–7058 CrossRef CAS PubMed.
- V. Baskar, M. Shanmugam, M. Helliwell, S. J. Teat and R. E. P. Winpenny, Reverse-Keggin ions: Polycondensation of antimonate ligands give inorganic cryptand, J. Am. Chem. Soc., 2007, 129, 3042–3043 CrossRef CAS PubMed.
- M. Barsukova, N. V. Izarova, R. N. Biboum, B. Keita, L. Nadjo, V. Ramachandran, N. S. Dalal, N. S. Antonova, J. J. Carbó, J. M. Poblet and U. Kortz, Polyoxopalladates encapsulating yttrium and lanthanide ions [XIIIPd12II(AsPh)8O32]5− (X = Y, Pr, Nd, Sm, Eu, Gd, Tb, Dy, Ho, Er, Tm, Yb, Lu), Chem.–Eur. J., 2010, 16, 9076–9085 CrossRef CAS PubMed.
- M. Barsukova-Stuckart, N. V. Izarova, R. A. Barrett, Z. Wang, J. van Tol, H. W. Kroto, N. S. Dalal, P. Jiménez-Lozano, J. J. Carbó, J. M. Poblet, M. S. von Gernler, T. Drewello, P. de Oliveira, B. Keita and U. Kortz, Polyoxopalladates encapsulating 8-coordinated metal ions, [MO8Pd12IIL8]n− (M = Sc3+, Mn2+, Fe3+, Co2+, Ni2+, Cu2+, Zn2+, Lu3+; L = PhAsO32−, PhPO32−, SeO32−), Inorg. Chem., 2012, 51, 13214–13228 CrossRef CAS PubMed.
- P. Yang, Y. Xiang, Z. Lin, B. S. Bassil, J. Cao, L. Fan, Y. Fan, M.-X. Li, P. Jiménez-Lozano, J. J. Carb, J. M. Poblet and U. Kortz, Alkaline earth guests in polyoxopalladate chemistry: From nanocube to nanostar via an open-shell structure, Angew. Chem., Int. Ed., 2014, 53, 11974–11978 CrossRef CAS.
- W. R. Fawcett, Thermodynamic parameters for the solvation of monatomic ions in water, J. Phys. Chem. B, 1999, 103, 11181–11185 CrossRef CAS.
- M. Nyman, J. P. Larentzos, E. J. Maginn, M. E. Welk, D. Ingersoll, H. Park, J. B. Parise, I. Bull and F. Bonhomme, Experimental and theoretical methods to investigate extraframework species in a layered material of dodecaniobate anions, Inorg. Chem., 2007, 46, 2067–2079 CrossRef CAS PubMed.
- M. Nyman, C. R. Powers, F. Bonhomme, T. M. Alam, E. J. Maginn and D. T. Hobbs, Ion-exchange behavior of one-dimensional linked dodecaniobate Keggin ion materials, Chem. Mater., 2008, 20, 2513–2521 CrossRef CAS.
- Z. Zhang, M. Sadakane, T. Murayama, N. Sakaguchi and W. Ueda, Preparation, structural characterization, and ion-exchange properties of two new zeolite-like 3D frameworks constructed by ε-Keggin-type polyoxometalates with binding metal ions, H11.4[ZnMo12O40Zn2]1.5− and H7.5[Mn0.2Mo12O40Mn2]2.1−, Inorg. Chem., 2014, 53, 7309–7318 CrossRef CAS PubMed.
- Z. Zhang, T. Murayama, M. Sadakane, H. Ariga, N. Yasuda, N. Sakaguchi, K. Asakura and W. Ueda, Nat. Commun., 2015, 6, 7731 CrossRef PubMed.
- Q. Zhu, Z. Zhang, M. Sadakane, A. Yoshida, M. Hara and W. Ueda, Synthesis of crystalline molybdenum oxides based on a 1D molecular structure and their ion-exchange properties, New J. Chem., 2017, 41, 4503–4509 RSC.
- T. Okuhara, H. Watanabe, T. Nishimura, K. Inumaru and M. Misono, Microstructure of cesium hydrogen salts of 12-tungstophosphoric acid relevant to novel acid catalysis, Chem. Mater., 2000, 12, 2230–2238 CrossRef CAS.
- T. Okuhara, Water-tolerant solid acid catalysts, Chem. Rev., 2002, 102, 3641–3666 CrossRef CAS PubMed.
- Y. Iwase, S. Sano, L. Mahardiani, R. Abe and Y. Kamiya, Bimodal cesium hydrogen salts of 12-tungstosilicic acid, CsxH4−xSiW12O40, as highly active solid acid catalysts for transesterification of glycerol tributyrate with methanol, J. Catal., 2014, 318, 34–42 CrossRef CAS.
- K. Okamoto, S. Uchida, T. Ito and N. Mizuno, Self-organization of all-inorganic dodecatungstophosphate nanocrystallites, J. Am. Chem. Soc., 2007, 129, 7378–7384 CrossRef CAS PubMed.
- Y. Ogasawara, S. Uchida, T. Maruichi, R. Ishikawa, N. Shibata, Y. Ikuhara and N. Mizuno, Cubic cesium hydrogen silicododecatungstate with anisotropic morphology and polyoxometalate vacancies exhibiting selective water sorption and cation-exchange properties, Chem. Mater., 2013, 25, 905–911 CrossRef CAS.
- D.-D. Zhou, Q. Zhao, F.-P. Zhu, Z.-G. Zhang, Y. Zhou, Z.-J. Yong, J.-P. Ma, Y. Kuroiwa, C. Moriyoshi, Y.-Z. Fang, J.-L. Gu, J. Shu, Z.-Y. Li, J.-M. Chen, L.-R. Zheng and H.-T. Sun, Ion-exchangeable microporous polyoxometalate compounds with off-center dopants exhibiting unconventional luminescence, Chem.–Eur. J., 2018, 24, 9976–9982 CrossRef CAS PubMed.
- S. Uchida, R. Eguchi and N. Mizuno, Zeotype organic–inorganic ionic crystals: Facile cation exchange and controllable sorption properties, Angew. Chem., Int. Ed., 2010, 49, 9930–9934 CrossRef CAS PubMed.
- T. Fujihara, J. Aonahata, S. Kumakura, A. Nagasawa, K. Murakami and T. Ito, Kinetic study on the substitution of dimethylacetamide for the terminal aqua ligands in the trinuclear chromium(III) complexes [Cr3(μ3-O)(μ-RCO2)6(H2O)3]+ (R = H, CH3, CH3CH2, CH2Cl, CHCl2, CH3OCH2, (CH3)3C, CH2ClCH2, (CH3CH2)2CH). Elucidation of the mechanism from the activation volumes and the substituent effects of bridging carboxylate ligands, Inorg. Chem., 1998, 37, 3779–3784 CrossRef CAS.
- S. Uchida, R. Hosono, R. Eguchi, R. Kawahara, R. Osuga, J. N. Kondo, M. Hibino and N. Mizuno, Proton conduction in alkali metal ion-exchanged porous ionic crystals, Phys. Chem. Chem. Phys., 2017, 19, 29077–29083 RSC.
- H. Wang, S. Hamanaka, Y. Nishimoto, S. Irle, T. Yokoyama, H. Yoshikawa and K. Awaga, In operando X-ray absorption fine structure studies of polyoxometalate molecular cluster batteries: Polyoxometalates as electron sponges, J. Am. Chem. Soc., 2012, 134, 4918–4924 CrossRef CAS PubMed.
- C. Ritchie, C. Streb, J. Thiel, S. G. Mitchell, H. N. Miras, D.-L. Long, T. Boyd, R. D. Peacock, T. McGlone and L. Cronin, Reversible redox reactions in and extended polyoxometalate framework solid, Angew. Chem., Int. Ed., 2008, 47, 6881–6884 CrossRef CAS PubMed.
- S. G. Mitchell, C. Streb, H. N. Miras, T. Boyd, D.-L. Long and L. Cronin, Face-directed self-assembly of an electronically active Archimedean polyoxometalate architecture, Nat. Chem., 2010, 2, 308–312 CrossRef CAS PubMed.
- R. Kawahara, S. Uchida and N. Mizuno, Redox-induced reversible uptake-release of cations in porous ionic crystals based on polyoxometalate: Cooperative migration of electrons with alkali metal ions, Chem. Mater., 2015, 27, 2092–2099 CrossRef CAS.
- S. Seino, R. Kawahara, Y. Ogasawara, N. Mizuno and S. Uchida, Reduction-induced highly selective uptake of Cs+ by an ionic crystal based on silicododecamolybdate, Angew. Chem., Int. Ed., 2016, 55, 3987–3991 CrossRef CAS PubMed.
- S. Hitose and S. Uchida, Rapid uptake/release of Cs+ in isostructural redox-active porous ionic crystals with large molecular size and easily reducible Dawson-type polyoxometalates as building blocks, Inorg. Chem., 2018, 57, 4833–4836 CrossRef CAS PubMed.
- E. C. Tyo and S. Vajda, Catalysis by clusters with precise numbers of atoms, Nat. Nanotechnol., 2015, 10, 577–588 CrossRef CAS PubMed.
- J. Shibata, K. Shimizu, Y. Takada, A. Shichi, H. Yoshida, S. Satokawa, A. Satsuma and T. Hattori, Structure of active Ag clusters in Ag zeolites for SCR of NO by propane in the presence of hydrogen, J. Catal., 2004, 227, 367–374 CrossRef CAS.
- S. Aghakhani, D. Grandjean, W. Baekelant, E. Coutiño-Gonzalez, E. Fron, K. Kvashnina, M. B. J. Roeffaers, J. Hofkens, B. F. Sels and P. Lievens, Nanoscale, 2018, 10, 11467–11476 RSC.
- Y. E. Cheon and M. P. Suh, Enhanced hydrogen storage by palladium nanoparticles fabricated in a redox-active metal–organic framework, Angew. Chem., Int. Ed., 2009, 48, 2899–2903 CrossRef CAS PubMed.
- H. L. Jiang, T. Akita, T. Ishida, M. Haruta and Q. Xu, Synergistic catalysis of Au@Ag core–shell nanoparticles stabilized on metal–organic framework, J. Am. Chem. Soc., 2011, 133, 1304–1306 CrossRef CAS PubMed.
- H. Liu, L. Chang, L. Chen and Y. Li, In situ one-step synthesis of metal–organic framework encapsulated naked Pt nanoparticles without additional reductants, J. Mater. Chem. A, 2015, 3, 8028–8033 RSC.
- H. Liu, L. Chang, C. Bai, L. Chen, R. Luque and Y. Li, Controllable encapsulation of “clean” metal clusters within MOFs through kinetic modulation: towards advanced heterogeneous nanocatalysts, Angew. Chem., Int. Ed., 2016, 55, 5019–5023 CrossRef CAS PubMed.
- J. J. Warren, T. A. Tronic and J. M. Mayer, Thermochemistry of proton-coupled electron transfer reagents and its implications, Chem. Rev., 2010, 110, 6961–7001 CrossRef CAS.
- S. Uchida, T. Okunaga, Y. Harada, S. Magira, Y. Noda, T. Mizuno and T. Tachikawa, Rapid formation of small mixed-valence luminescent silver clusters via cation-coupled electron-transfer in redox-active porous ionic crystal based on dodecamolybdophosphate, Nanoscale, 2019, 11, 5460–5466 RSC.
- Y. Lei, F. Mehmood, J. Greeley, B. Lee, S. Seifert, R. E. Winans, J. W. Elam, R. J. Meyer, P. C. Redfern, D. Teschner, R. Schlögl, M. J. Pellin, L. A. Curtiss and S. Vajda, Increased silver activity for direct propylene epoxidation via subnanometer size effects, Science, 2010, 328, 224–228 CrossRef CAS PubMed.
- E. Cadot, B. Salignac, T. Loiseau, A. Dolbecq and F. Sécheresse, Syntheses and 31P NMR studies of cyclic oxothiomolybdate(V) molecular rings: Exchange properties and crystal structures of the monophosphate decamer [(H2PO4)Mo10S10O10(OH)11(H2O)2]2− and the diphosphate dodecamer [(HPO4)2Mo12S12O12(OH)12(H2O)2]4−, Chem.–Eur. J., 1999, 5, 3390–3398 CrossRef CAS.
- Y. Ji, L. Huang, J. Hu, C. Streb and Y.-F. Song, Polyoxometalate-functionalized nanocarbon materials for energy conversion, energy storage and sensor systems, Energy Environ. Sci., 2015, 8, 776–789 RSC.
- H. Kageyama, K. Hayashi, K. Maeda, J. P. Attfield, Z. Hiroi, J. M. Rondinelli and K. R. Poeppelmeier, Expanding frontiers in materials chemistry and physics with multiple anions, Nat. Commun., 2018, 9, 772 CrossRef PubMed.
- B. F. Hoskins and R. Robson, Design and construction of a new class of scaffolding-like materials comprising infinite polymeric frameworks of 3D-linked molecular rods. A reappraisal of the zinc cyanide and cadmium cyanide structures and the synthesis and structure of the diamond-related frameworks [N(CH3)4][CuIZnII(CN)4] and CuI[4,4′,4′′,4′′′-tetracyanotetraphenylmethane]BF4·xC6H5NO2, J. Am. Chem. Soc., 1990, 112, 1546–1554 CrossRef CAS.
- B. Hribar, N. T. Southall, V. Vlachy and K. A. Dill, How ions affect the structure of water, J. Am. Chem. Soc., 2002, 124, 12302–12311 CrossRef CAS PubMed.
- R. Custelcean and B. A. Moyer, Anion separation with metal–organic frameworks, Eur. J. Inorg. Chem., 2007, 1321–1340 CrossRef CAS.
- T. Kotabe, Y. Ogasawara, K. Suzuki, S. Uchida, N. Mizuno and K. Yamaguchi, Porous cubic cesium salts of silicododecatungstate(molybdate)/borododecatungstate blends: Synthesis and molecular adsorption properties, Inorg. Chem., 2018, 57, 8821–8830 CrossRef CAS PubMed.
|
This journal is © The Royal Society of Chemistry 2019 |
Click here to see how this site uses Cookies. View our privacy policy here.