Low-temperature synthesis of CaZrTi2O7 zirconolite-type materials using ceramic, coprecipitation, and sol–gel methods†
Received
9th September 2018
, Accepted 3rd December 2018
First published on 4th December 2018
Abstract
Materials adopting the zirconolite-type structure have been investigated previously for use in many applications, including for the sequestration of nuclear waste. However, these materials are often formed using high-temperature methods (e.g., 1400 °C), which are not easy to scale up to produce large quantities. The effects of solution-based synthesis methods (coprecipitation and sol–gel) on the long- and short-range structures of zirconolite-type oxides annealed at various temperatures was investigated in the current study. Powder X-ray diffraction (XRD) was used to study the formation of the zirconolite phase at all temperatures studied (700 to 1400 °C). While the zirconolite structure was observed to be formed over the temperature range of 900 to 1400 °C, a mixture of zirconolite and defect fluorite phases were observed when the annealing temperature was 800 °C and only the defect fluorite structure was observed when the annealing temperature was 700 °C. Changes in the long-range structure of the zirconolite-type materials were evaluated by Rietveld refinement of the diffraction patterns. X-ray absorption near edge spectroscopy (XANES) was used to probe the local structure of Ti and Zr within the materials. Examination of the Ti K- and Zr K-edge XANES spectra showed no change in the local structure of the zirconolite-type materials annealed at temperatures as low as 900 °C while changes were observed when lower annealing temperatures were used owing to the presence of both the zirconolite and defect fluorite phases. It has been shown in this study that zirconolite-type materials can be synthesized at temperatures as low as 900 °C using coprecipitation or sol–gel methods due to the atomic scale mixing of reactants which occurs in solution. The radiation resistance of the materials synthesized using the ceramic, coprecipitation or sol–gel methods was investigated by implanting the materials with high energy Au ions and studying the changes in the local structure of these materials using glancing angle XANES. The resistance of these materials to radiation induced damage was found to be similar regardless of the synthesis method used to form them, which is important if these materials are to be used as nuclear waste forms.
1. Introduction
Nuclear power is a major source of electricity worldwide.1,2 As of 2017, there were 446 operable reactors which were responsible for generating 11.5% of the world's electricity.2 However, a substantial amount of nuclear waste is produced, which needs to be contained and isolated from the environment for the long-term. Ceramic oxide materials have attracted a substantial amount of interest as nuclear waste forms, with many different compositions being proposed, including brannerite-(Ce2Ti2O6), hollandite-(BaAl2Ti6O16), perovskite-(ABO3), zircon-(ZrSiO4), pyrochlore-(A2B2O7), and zirconolite-type (CaZrTi2O7) oxides.3–12 This is partially because of the existence of naturally occurring analogues which have been shown to contain radionuclides and withstand radiation damage over geological timescales.3,4,6,8,9,11,13–19 The ideal mineral formula of zirconolite is CaZrTi2O7 which crystallizes in the C2/c space group. The structure consists of two different cation layers, one composed of 8-coordinate Ca2+ and 7-coordinate Zr4+ polyhedra and the other of corner sharing 6-coordinate and 5-coordinate Ti4+ polyhedra in a 2
:
1 ratio (Fig. 1).4,6,7,17,20–24 Several zirconolite polytypes exist (e.g. 2M, 3T, 3O, 4M, and 6T) that vary according to the layer stacking pattern.6,7,10,20,23–25 The 2M polytype forms for compositions of CaZrxTi3−xO7 where x ranges from 0.7 to 1.3.20,25 Zirconolite-type oxides have been investigated for several decades for many applications including nuclear waste sequestration applications. Actinides and rare-earth elements may substitute into both the Ca and Zr sites, with charge balance being maintained by the substitution of Mg, Al, Fe, Nb, Ta, or W for Ti.4,6,7,9,10,20,21,23,26,27
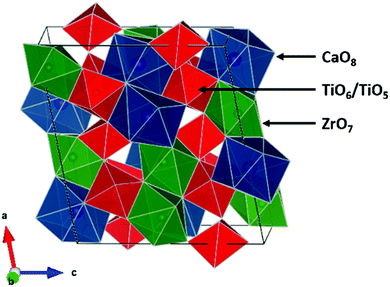 |
| Fig. 1 The 2M zirconolite-type structure (CaZrTi2O7) is shown. The 8-coordinate Ca-site is shown in blue. The 7-coordinate Zr-site is shown in green. The 6- and 5-coordinate Ti-sites are shown in red. The structure was generated using the VESTA software program. | |
The ceramic method is a common method used to synthesize zirconolite-type materials; however, this method often requires the use of high annealing temperatures and relatively long reaction times. It has been demonstrated previously that solution-based methods can be used to form ceramic materials, potentially at lower than expected annealing temperatures.28,29 One of the most widely used solution-based synthesis methods to prepare ceramic materials is the sol–gel method. This technique is broadly defined as the formation of a gel from a solution, resulting in the formation of a three-dimensional solid network.30–32 A relatively small amount of energy is required to arrange the ions into an ordered, crystalline material because the solid structure is already mostly formed. Another solution-based synthesis method, the coprecipitation method, requires the precipitation of all metals in solution, typically as hydroxides.33–35 The diffusion distances required to form the material are shortened due to the very small particle size of the precursors, similarly reducing the energy required to form the product. In contrast to these solution-based synthesis methods, the ceramic method requires the diffusion of ions through many lattice sites and defects, which is a much higher energy process. Sol–gel and coprecipitation methods are commonly used to prepare materials at relatively lower annealing temperatures for this reason, particularly in synthetic applications where high temperatures are not feasible.31,33,36–42
As mentioned above, zirconolite-type oxides have been proposed for the storage of actinide elements commonly present in high level (nuclear) waste (HLW), which typically undergo α-decay and emit an α-particle and a heavy, high energy daughter product (i.e., recoil nucleus).1,11,13–15,21,40,43,44 Radiation induced damage to the structure of a waste form can lead to swelling and cracking of the waste form, which may affect the leaching characteristics of the material. The two most common approaches used to study the effect of radioactive decay within a material are direct incorporation of radionuclides into the material causing in situ radiation damage (i.e., self-radiation) or external irradiation of the material using an ion accelerator (i.e., ion implantation).6,15,43–47 Ion implantation is particularly useful to simulate the interactions of the daughter products with the surrounding material in reasonable time scales (minutes to hours) rather than geologic time scales (thousands of years).6,15,17,40,44,46,48
In terms of nuclear waste sequestration applications, industrial-scale synthesis of waste form materials needs to be as cost-effective as possible. Low-temperature synthesis methods are thus highly desirable, as they reduce equipment and operating costs. In this study, zirconolite-type (CaZrTi2O7) materials were prepared by ceramic, coprecipitation, and sol–gel methods to determine the effect of synthesis method on the long-range and local structures of the materials. The synthesized materials were studied using a combination of powder X-ray diffraction (XRD) and X-ray absorption near-edge spectroscopy (XANES) to determine the lowest temperature at which phase-pure CaZrTi2O7 could be formed. The effect of synthesis method on the radiation resistance of these materials was also investigated by implanting the materials with high energy Au ions and comparing the change in local structure that occurred by use of glancing angle (GA-) XANES.
2. Experimental
2.1 Synthesis
Zirconolite-type materials (CaZrTi2O7) were synthesized using the ceramic method, a coprecipitation method, and a sol–gel method. For the ceramic method, CaCO3 (EMD, ≥99.0%), ZrO2 (Alfa Aesar, 99.7%), and TiO2 (Alfa Aesar, 99.6%) powders were mixed stoichiometrically and pressed into a pellet uniaxially at 6 MPa. The pellets were placed in an alumina crucible and heated in air at temperatures ranging from 900 to 1450 °C for 6 days with intermediate grinding and pelleting after 3 days of annealing followed by quench cooling in air.
For the coprecipitation method, calcium carbonate, titanium tert-butoxide, and an aqueous zirconium solution were used as reagents. The zirconium solution was prepared by dissolving ZrOCl2·xH2O (Alfa Aesar, 99.9%) in HNO3 (pH ≈ 1). The concentration of zirconium in this solution was determined by ICP-AES analysis. CaCO3 and Ti[OC(CH3)3]4 (Acros Organics, 98%) were added to an appropriate amount of the zirconium solution and stirred until dissolved. CaCO3 was added in 100% stoichiometric excess to account for the partial solubility of Ca(OH)2.49 NH4OH (Fisher Scientific, 28.0–30.0%) was then added to the solution until a pH of 10 was reached to precipitate all metals as hydroxides. The mixtures were then vacuum filtered and the filter paper was burned off at 650 °C, resulting in a dry powder precursor. The white powders were pelletized and annealed at temperatures ranging from 900 to 1400 °C for 6 days and 700 to 800 °C for 12 days followed by quench cooling in air.
A modified Pechini method was used for the sol–gel method due to the compatibility of this method with aqueous reagents. The Pechini method results in gelation by the condensation polymerization of a polyhydroxy alcohol and a carboxylic acid metal complex, which are typically ethylene glycol and citric acid, respectively. An appropriate amount of zirconium solution was first concentrated to a volume of ∼1 mL by heating to evaporate water (to improve the quality of the gel obtained and gelation time) followed by the addition of 2 mL of ethylene glycol (Alfa Aesar, 99%) to prepare 0.25 g of product. Stoichiometric amounts of CaCO3 and Ti[OC(CH3)3]4 were then added along with 1 mL of concentrated HNO3 and 0.5 mL of glacial acetic acid (Fisher, 99.7%). The solution was stirred in a sand bath at 80 ± 5 °C for ∼2 hours until an opaque white gel was obtained. The gel was then dried in a furnace at 250 °C for 1 hour, resulting in a fine black powder. This powder was ground and heated at 650 °C for 6 hours to burn off any remaining carbon-containing components, resulting in a fine white powder. This powder was then pelleted and annealed at 1400 °C for 3 days, 1200 or 900 °C for 6 days, or 800 °C for 9 days with intermediate grinding as previously described followed by quench cooling in air.
2.2 Powder XRD
Powder XRD patterns were collected using a PANalytical Empyrean X-ray diffractometer equipped with a Co Kα1,2 X-ray source for phase analysis in the 2θ range of 10–80° with a step size of 0.017°. Diffraction patterns used for Rietveld refinement were collected using the same instrument but a Cu Kα1,2 X-ray source was used and the 2θ range was 10–110°. Rietveld refinement was performed using the X’Pert HighScore Plus software program. The scale factor, zero shift, lattice constants, atomic positional parameters, profile variables, and the overall isotropic thermal parameter (BOVL) were allowed to vary during the refinement. The XRD patterns are presented with 2θ values representative of a Co X-ray source for consistency.
2.3 SEM
Scanning electron microscopy (SEM) was performed to study the particle size of CaZrTi2O7 materials prepared by the ceramic method (annealed at 1400 °C), the coprecipitation method (annealed at 900 °C), or the sol–gel method (annealed at 900 °C). Imaging was performed using a JEOL 840A scanning electron microscope. Samples were mounted on double-sided carbon tape and sputter coated with approximately 200 Å of gold prior to loading them into the SEM. Images were acquired at 15 kV under a working distance of 15 mm and were analyzed using the Gellar Analytical's dPict image acquisition software program.
2.4 Ion implantation
Zirconolite-type materials were implanted with a high energy (2 MeV) beam of Au− ions to a dose of 5.0 × 1014 ions per cm2 using the 1.7 MeV Tandetron accelerator located at Interface Science Western (ISW), University of Western Ontario. Information on the materials that were ion implanted can be found in Table S1 (ESI†). The ion beam was aligned normal to the surface of the pellet.
The ion implantation depth and the number of vacancies per Au− ion were calculated using the Stopping Range of Ions in Matter (SRIM-2013) software package and are presented in Fig. S1 (ESI†).50 The simulations were performed using 5000 Au− ions with an ion beam energy of 2 MeV. The target displacement energies (the energy required to permanently displace an atom from its lattice site to a defect position) were assumed to be 25 eV for Ca/Ti/Zr and 28 eV for O.50,51 The density of CaZrTi2O7 used in the calculations was 4.44 g cm−3. The X-ray attenuation depth in the materials was calculated at the Ti K-edge XANES absorption energy (4966 eV) using a program from Lawrence Berkeley National Laboratory.52 This program utilizes the Beer–Lambert law to calculate the depth to which X-rays of a given energy will penetrate a material as a function of angle relative to the surface of the material. Two angles were chosen to be studied. The first angle (0.8°) corresponds to a depth of ∼100 nm within the material which is likely to be the most damaged region. The second angle (3.1°) was chosen to probe the entire region in which Au− ions were calculated to be implanted.
2.5 Zr K- and Ti K-edge XANES and Ti K-edge GA-XANES
Zr and Ti K-edge XANES spectra were collected using the Sector 20 bending magnet (20BM) beamline located at the APS.53 Samples were finely ground, spread onto Kapton tape, and layered to maximize absorption. XANES spectra were collected in partial fluorescence mode using a 13-element Ge detector and in transmission mode with 100% N2 gas in the incident ion chamber and a mixture of Ar (Zr K-edge XANES) or He (Ti K-edge XANES) and N2 gases in the transmission and reference chambers. A Si(111) double crystal monochromator was used, providing a spectral resolution of 2.5 eV at 17
998 eV and 0.7 eV at 4966 eV for the Zr and Ti K-edge XANES spectra, respectively.53 Spectra from Zr metal foil (E0 = 17
998 eV) and Ti metal foil (E0 = 4966 eV) were collected concurrently in transmission mode and used for calibration. XANES spectra were collected using a step size of 0.3 eV and 0.15 eV through the absorption edge for Zr and Ti K-edge XANES spectra, respectively.
Ti K-edge GA-XANES spectra were collected from ion-implanted materials using the 20BM beamline in partial fluorescence mode.53 The ion-implanted pellets were mounted on carbon tape and attached to a motor driven Huber goniometer which was used to adjust the glancing angle. The X-ray beam was vertically focused with a height of 2 mm and a beam width of 50 μm. The 0° angle was calibrated by adjusting the angle of the sample to achieve maximum transmission. The glancing angle was set to the desired value with a precision of 0.1° to probe different depths of the ion implanted material. All XANES spectra were calibrated, normalized, and analyzed using the Athena software program.54
3. Results and discussion
3.1 Investigation of the long-range structure and particle size
3.1.1 Powder XRD and Rietveld refinement.
The powder XRD patterns from CaZrTi2O7 zirconolite-type materials prepared by the ceramic method after annealing for 6 days are shown in Fig. 2. CaTiO3, ZrO2, and TiO2 impurities are present in the materials annealed at temperatures below 1400 °C. XRD patterns from the materials prepared by the coprecipitation and sol–gel methods are presented in Fig. 3(a and b). These patterns are compared to the pattern from a zirconolite material prepared by the ceramic method and annealed at 1450 °C. This temperature was chosen for the ceramic method to ensure complete crystallization of the zirconolite phase, which is particularly important for XANES analysis (vide infra). The patterns from the samples prepared by the coprecipitation method (annealed at temperatures ranging from 1300 to 900 °C) and the sol–gel method (annealed at temperatures ranging from 1400 to 900 °C) are indicative of the formation of the zirconolite-type structure. An increase in the width of the diffraction peaks from the materials synthesized using the coprecipitation and sol–gel methods was observed with decreasing annealing temperature as a result of a decrease in crystallite size, which is discussed further in Section 3.1.2. The powder XRD patterns from the coprecipitation and sol–gel synthesized materials that were annealed at 800 °C were observed to contain some diffraction peaks that were not representative of the zirconolite-type structure. To further investigate this unknown phase, a material was prepared by the coprecipitation method and annealed at 700 °C. Fig. 3(c) shows that after annealing at 700 °C, only peaks corresponding to the unknown phase were observed. The peak positions and relative intensities were determined to be indicative of a defect fluorite-type structure, which contains O anion vacancies. Rietveld refinement was performed on the XRD pattern from this material using a (Ca0.25Zr0.25Ti0.5)O1.75 defect fluorite-type structure as the model. The theoretical site occupancy values were used due to the difficulty in refining site occupancies of three cations in one site. The results from this refinement are shown in Fig. 3(d) and a skeletal representation of this structure is presented in Fig. S2 in the ESI.† This analysis indicates the formation of a defect fluorite-type phase when the annealing temperature was 700 °C and a mixture of defect fluorite- and zirconolite-type phases when the annealing temperature was 800 °C.
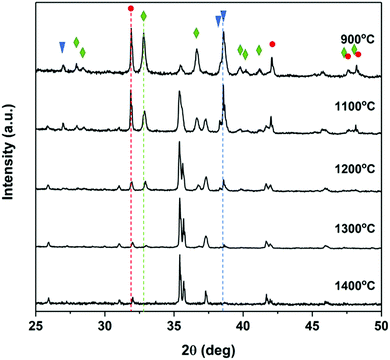 |
| Fig. 2 Powder XRD patterns from CaZrTi2O7 materials prepared by the ceramic method and annealed at various temperatures for 6 days. Dotted lines mark the major peak from impurity phases. Impurities are marked by blue triangles (CaTiO3), green diamonds (ZrO2), and red circles (TiO2; rutile). | |
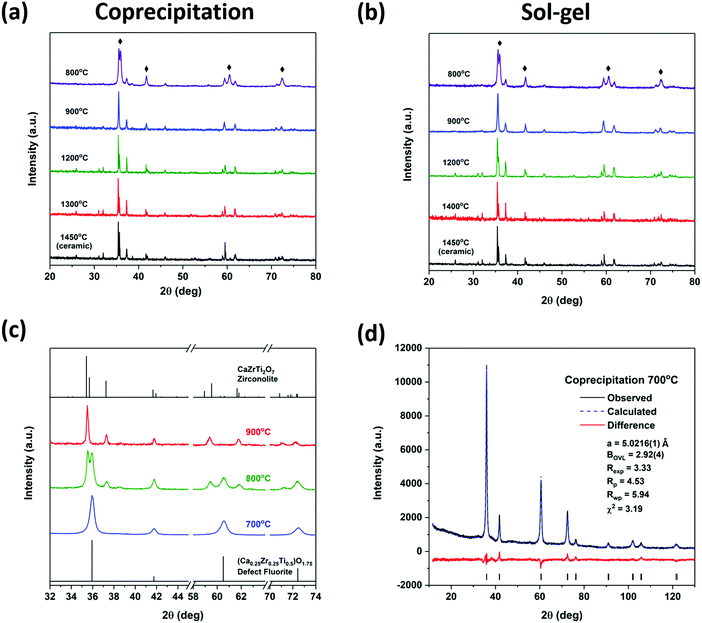 |
| Fig. 3 Powder XRD patterns from CaZrTi2O7 zirconolite-type materials prepared by the (a) coprecipitation and (b) sol–gel methods. All patterns are compared to CaZrTi2O7 prepared by the ceramic method and annealed at 1450 °C. Diamonds indicate peaks from a secondary phase. (c) Enlarged XRD patterns from CaZrTi2O7 materials prepared by the coprecipitation method and annealed at 900, 800, and 700 °C. (d) Rietveld refinement of the diffraction pattern from the material prepared by the coprecipitation method and annealed at 700 °C. The pattern was fitted to a (Ca0.25Zr0.25Ti0.5)O1.75 defect fluorite-type model and the expected major reflections for this structure-type are indicated in the plot. The calculated diffraction patterns from materials adopting the zirconolite- or defect fluorite-type structures are included in (c) for comparison. | |
The long-range order of the zirconolite materials prepared by the coprecipitation and sol–gel methods followed by annealing at high (1300/1400 °C) and low (900 °C) temperatures was examined by Rietveld refinement of the powder XRD patterns. The diffraction patterns from materials annealed at 800 °C were not refined as these materials were not phase-pure zirconolite. The refined patterns show good fits to the experimental data (Fig. 4) and a skeletal representation of this structure is presented in Fig. S3 in the ESI.† Small changes in the lattice parameters and atomic coordinates (Table 1) were observed depending on the annealing temperature used. Generally, a, c, and β increased and b decreased with decreasing annealing temperature. Despite these changes in lattice parameters, there was <0.1% change in the cell volume. Therefore, the materials prepared at high and low temperatures using either synthesis method (coprecipitation or sol–gel) show very little long-range structural changes.
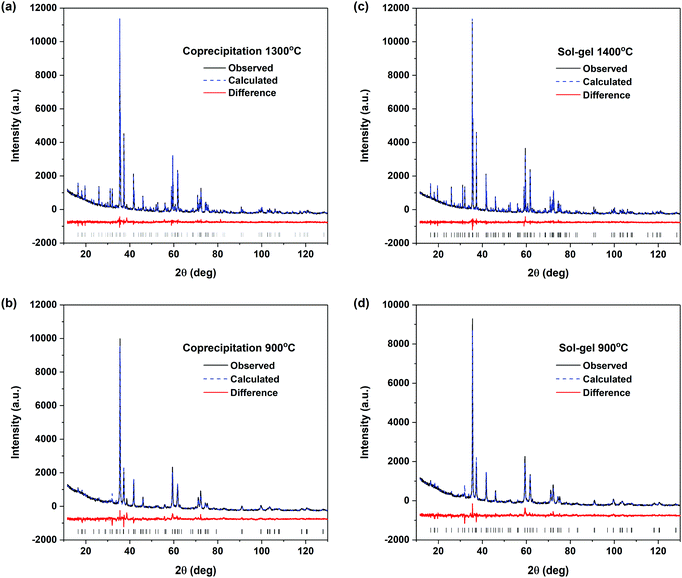 |
| Fig. 4 Rietveld refined powder XRD patterns from the CaZrTi2O7 materials prepared by the coprecipitation method and annealed at (a) 1300 °C and (b) 900 °C, and prepared by the sol–gel method and annealed at (c) 1400 °C and (d) 900 °C. The expected major reflections for the zirconolite-type structure are indicated in the plots. | |
Table 1 Results of the Rietveld refinements of the XRD patterns from the CaZrTi2O7 materials prepared by the coprecipitation (P) and sol–gel (S) methods and annealed at high and low temperatures. The starting model for the refinement was taken from ref. 24. Oxygen positional parameters were not refined further
|
Ref. 24
|
P-1300 °C |
P-900 °C |
S-1400 °C |
S-900 °C |
a (Å) |
12.4458(7) |
12.4531(2) |
12.508(1) |
12.4462(1) |
12.510(1) |
b (Å) |
7.2734(4) |
7.2755(1) |
7.2294(8) |
7.27174(8) |
7.2314(7) |
c (Å) |
11.3942(9) |
11.3814(2) |
11.4064(9) |
11.3813(8) |
11.3987(7) |
β (°) |
100.533(7) |
100.570(1) |
100.69(1) |
100.5587(8) |
100.669(9) |
B
OVL
|
— |
0.54(3) |
1.21(5) |
0.27(3) |
0.81(4) |
Cell volume (Å3) |
1014.1 |
1013.7 |
1013.5 |
1012.6 |
1012.0 |
Ca1 |
8f |
x
|
0.373(1) |
0.3727(5) |
0.377(1) |
0.3725(5) |
0.384(1) |
y
|
0.123(2) |
0.127(1) |
0.097(3) |
0.125(1) |
0.136(2) |
z
|
0.4949(8) |
0.4979(4) |
0.503(1) |
0.4967(4) |
0.505(1) |
Zr1 |
8f |
x
|
0.1222(5) |
0.1236(2) |
0.1288(6) |
0.1238(2) |
0.1314(7) |
y
|
0.1222(9) |
0.1247(5) |
0.108(1) |
0.1239(5) |
0.113(1) |
z
|
0.9744(5) |
0.9748(2) |
0.9900(7) |
0.9749(2) |
0.9894(5) |
Ti1 |
8f |
x
|
0.2497(9) |
0.2525(5) |
0.244(1) |
0.2505(5) |
0.247(1) |
y
|
0.122(2) |
0.126(1) |
0.138(3) |
0.124(1) |
0.138(2) |
z
|
0.7467(8) |
0.7451(4) |
0.741(1) |
0.7436(4) |
0.7437(8) |
Ti2 |
8f |
x
|
0.474(2) |
0.4721(8) |
0.517(4) |
0.4720(9) |
0.492(4) |
y
|
0.056(2) |
0.0573(9) |
0.097(3) |
0.0500(9) |
0.095(3) |
z
|
0.250(3) |
0.249(1) |
0.275(2) |
0.256(1) |
0.222(2) |
Ti3 |
4e |
y
|
0.127(2) |
0.125(1) |
0.124(3) |
0.126(1) |
0.114(3) |
R
exp
|
— |
4.44 |
4.33 |
4.69 |
4.38 |
R
p
|
— |
4.64 |
6.47 |
4.87 |
5.64 |
R
wp
|
— |
6.04 |
8.80 |
6.32 |
7.51 |
χ
2
|
— |
1.85 |
4.12 |
1.82 |
2.94 |
3.1.2 SEM.
SEM images were obtained to compare the particle sizes of materials prepared by the three synthesis methods at high and low annealing temperatures. Fig. 5 shows the SEM images from the powdered materials prepared by the ceramic method and annealed at 1400 °C and the samples prepared by the coprecipitation and sol–gel methods followed by annealing at 900 °C. The particle sizes of the materials were observed to be significantly larger (∼10–20 μm) when prepared by the ceramic method and annealed at high temperatures compared to the materials prepared by either the coprecipitation or sol–gel methods (∼200–500 nm) that were annealed at lower temperatures (i.e., 900 °C). The materials prepared by the coprecipitation and sol–gel methods followed by annealing at 900 °C were similar in particle size and distribution (Fig. 5(b and c)). This trend in particle size is also reflected in the crystallite size of these materials that was determined by analysis of the XRD patterns (Fig. 3) and using the Scherrer equation. The crystallite sizes of the materials prepared by the coprecipitation method were calculated to be 290 ± 30 and 70 ± 20 nm for materials annealed at 1300 and 900 °C, respectively. Similarly, the crystallite sizes of the materials prepared by the sol–gel method were calculated to be 600 ± 100 and 70 ± 10 nm for materials annealed at 1400 and 900 °C, respectively.
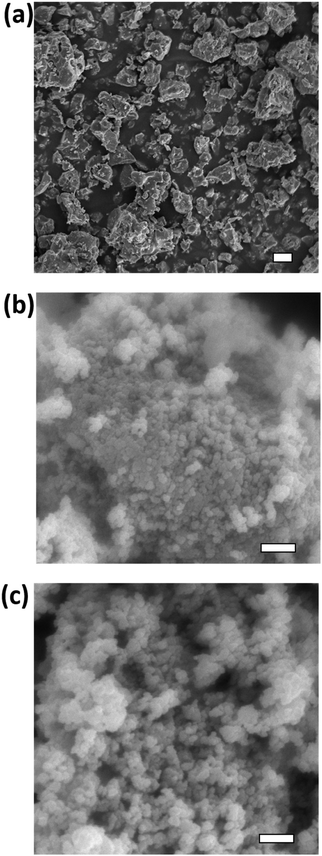 |
| Fig. 5 SEM images of CaZrTi2O7 materials (a) prepared by the ceramic method and annealed at 1400 °C (the scale bar is 10 μm); (b) prepared by the coprecipitation method and annealed at 900 °C (the scale bar is 1 μm); (c) prepared by the sol–gel method and annealed at 900 °C (the scale bar is 1 μm). | |
3.2 Investigation of the local structure of the synthesized materials
Zirconium and Ti K-edge XANES spectra were collected to determine the effect of decreasing annealing temperature on the short-range order of the zirconolite-type structure. XANES is a powerful technique for investigating the structural and electronic properties of materials. The line shape and intensity of the pre-edge and main-edge features found in the K-edge XANES spectra from transition-metals provide information on the local coordination number (CN), oxidation state, and bonding environment of the absorbing atom, allowing for changes in the local structure resulting from changes in synthesis conditions to be detected.3,55 The pre-edge region in K-edge spectra from transition-metals results (primarily) from 1s → (n − 1)d transitions, which are forbidden by the dipolar selection rule that governs XANES resulting in weak pre-edge peaks being observed; however, the intensity of these features can increase as a result of the overlap of np and (n − 1)d orbitals because of a loss of inversion symmetry.55 The main-edge features, which result from 1s → np transitions, are also sensitive to changes in the local structure of the absorbing atom because of changes in the coordination environment.
3.2.1 Zr K-edge XANES.
Zr K-edge XANES spectra show pre-edge and main-edge features that are a result of 1s → 4d (≤18
005 eV) and 1s → 5p (≥18
005 eV) transitions, respectively.56,57 A distinct pre-edge peak (1s → 4d) is not observed in the Zr K-edge XANES spectra because of the low resolution of the spectra and the decreased separation between Zr 4d and 5p orbitals when compared to first row transition metals like Ti (cf., Fig. 5 and 6). Although the resolution of the pre-edge peak in the Zr K-edge XANES spectra makes it difficult to investigate changes in Zr CN, the main-edge features are sensitive to CN changes. The Zr K-edge XANES spectra were collected from the zirconolite materials made by the ceramic method (annealed at 1450 °C), the coprecipitation method, and the sol–gel method, and are presented in Fig. 6(a and b). No differences in the line shape of the spectra from any of the materials prepared by the coprecipitation or sol–gel methods followed by annealing at 900 or 1400 °C were observed when compared to the spectrum from the material prepared by the ceramic method. The spectra from both materials annealed at 800 °C show very small changes in the line shape of the spectra. The XRD patterns from the materials prepared by the coprecipitation and sol–gel methods annealed at 800 °C showed that Zr is present in both CaZrTi2O7 and a defect fluorite-type phase. Zr is 7-coordinate in the zirconolite structure and 7-coordinate in the defect fluorite structure. The rounded feature above the absorption edge is typical of either 7-coordinate Zr or 8-coordinate Zr with longer than average bond lengths, such as in zirconolite (7-coordinate; average bond length = 2.166 Å) or zirconium sulphate tetrahydrate (8-coordinate; average bond length = 2.180 Å).58 This feature can be split into two peaks if Zr is octahedrally coordinated, as in perovskite, or if the environment is 8-coordinate but highly distorted, as in zircon, as shown in Fig. 6(c).58 The changes in the line shape of the spectrum from the material prepared by the coprecipitation method and annealed at 800 °C resemble the shape of the zircon spectrum where Zr is in a highly distorted 8-coordinate environment. These changes are very small, which is due to the similarity in the local environment of Zr in zirconolite- and defect fluorite-type structures and the energy resolution of the spectra (∼2.5 eV).
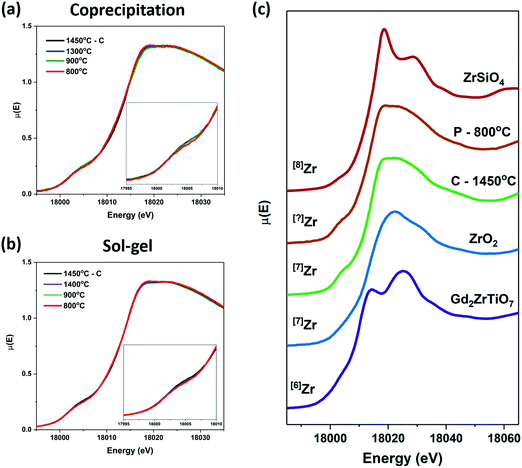 |
| Fig. 6 Zr K-edge XANES spectra from CaZrTi2O7 materials prepared by (a) the coprecipitation method and (b) by the sol–gel method, compared to the spectrum from the material prepared by the ceramic method (black spectrum). The pre-edge region is highlighted in the inset of each figure. (c) Zr K-edge spectra from reference compounds with different Zr coordination numbers. | |
3.2.2 Ti K-edge XANES.
Ti K-edge XANES spectra were also collected to further study changes in the local structure that occur as a result of synthesis method and annealing temperature used to form these zirconolite-type materials. As was the case for the Zr K-edge XANES spectra discussed above, the Ti K-edge XANES spectra contain transitions resulting from both quadrupolar (i.e., 1s → 3d; pre-edge) and dipolar (i.e., 1s → 4p; main-edge) transitions. Unlike the Zr K-edge XANES spectra, the higher resolution (0.7 eV vs. 2.5 eV) of the Ti K-edge XANES spectra results in a defined separation between the pre-edge and main-edge features resulting in the pre- and main-edge regions of Ti K-edge XANES spectra being very sensitive to changes in the local structural environment of Ti.
Ti K-edge XANES spectra were collected from the zirconolite materials made by the ceramic method (annealed at 1450 °C), the coprecipitation method, and the sol–gel method. These spectra are presented in Fig. 7(a and b). The line shapes of the spectra from the materials prepared by the coprecipitation and sol–gel methods annealed at 1400, 1300 °C, and 900 °C are identical to the line shape of the spectrum from the material prepared by the ceramic method and annealed at 1450 °C. The spectra from both the coprecipitation and sol–gel materials annealed at 800 °C show small changes in the main edge feature. Fig. 7(c) shows the spectra from the material prepared by the ceramic method and annealed at 1450 °C and the material prepared by the coprecipitation method and annealed at 800 °C compared to reference spectra from materials with different Ti coordination numbers. The local environment of Ti in zirconolite is a mixture of 5- and 6-coordinate. An increase in the intensity of the pre-edge peak was not observed, which would indicate that there was not an increase in the amount of 4- or 5-coordinate Ti in the materials prepared at 800 °C. The narrowing of the main edge feature appears to mimic the shape of the Gd2Ti2O7 pyrochlore spectrum, indicating that the amount of 6- or (potentially) 7-coordinate Ti relative to 5-coordinate is higher in the material prepared at 800 °C than at higher temperatures. This is supported by the presence of peaks corresponding to a defect fluorite-type phase in the diffraction pattern of the material annealed at 800 °C.
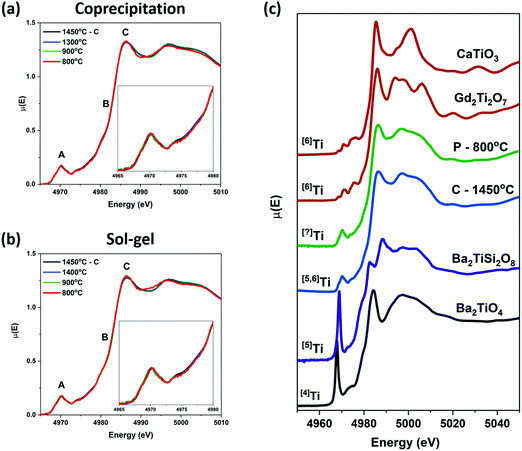 |
| Fig. 7 Ti K-edge XANES spectra from the CaZrTi2O7 materials prepared by (a) the coprecipitation method and (b) by the sol–gel method, compared to the spectrum from the material prepared by the ceramic method (black spectrum). The pre-edge (A) and main edge (B and C) regions are indicated. (c) Ti K-edge XANES spectra from the CaZrTi2O7 materials prepared by the ceramic (C) and coprecipitation (P) methods annealed at 1450 and 800 °C, respectively, and reference compounds with different Ti coordination numbers. | |
3.3 Investigation of the effect of ion implantation on the local structure of the materials depending on synthesis method
Ti K-edge GA-XANES spectra were collected from ion-implanted CaZrTi2O7 materials prepared by the ceramic, coprecipitation, and sol–gel methods to determine the effect of the synthesis method on the resistance of these materials to radiation-induced structural damage (Fig. 8). Spectra were collected at two angles from each material, one low angle to probe the most damaged region and another higher angle to probe the entire damaged region of the material, as determined by calculations using the SRIM program (see Fig. S1, ESI†). These spectra were then compared to the spectrum from the as-synthesized material to show the change in the structure of the material after ion implantation.
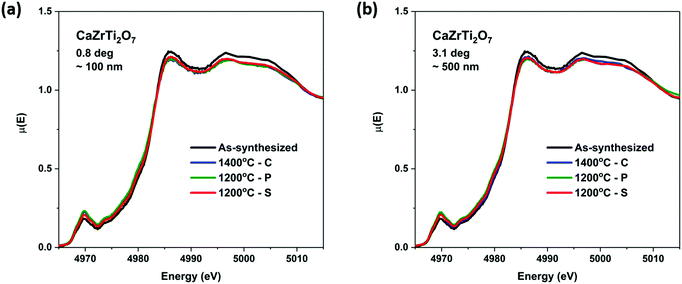 |
| Fig. 8 Ti K-edge GA-XANES spectra from ion-implanted CaZrTi2O7 materials prepared by the ceramic (c), coprecipitation (p), and sol–gel (s) methods collected at (a) 0.8°/∼100 nm and (b) 3.1°/∼500 nm. The “As-synthesized” materials were prepared by the ceramic method and annealed at 1400 °C. | |
As can be seen in Fig. 8 and suggested previously, zirconolite-type materials are relatively resistant to radiation-induced structural damage.4 A small decrease in the intensity of the main-edge feature and an increase in the intensity of the pre-edge feature was observed when the Ti K-edge GA-XANES spectra from the ion implanted materials are compared to the spectrum from the as-synthesized material. This can be attributed to a decrease in the coordination number of Ti in the material because of partial amorphization.21,59 The degree of amorphization can be concluded to be very small because the change in the spectrum after implantation is minor.21 The spectra from the ion implanted CaZrTi2O7 materials prepared by the coprecipitation and sol–gel methods overlap with the spectrum from the ion implanted material made by the ceramic method. This indicates that preparation of CaZrTi2O7 zirconolite-type materials by solution-based synthesis methods (i.e., coprecipitation and sol–gel) does not appear to affect the radiation resistance properties of the materials, even when lower annealing temperatures are used.
4. Conclusions
Zirconolite-type oxides (CaZrTi2O7) have been successfully synthesized using a coprecipitation method and a sol–gel method with annealing temperatures ranging from 900 to 1400 °C. Materials synthesized using these methods followed by annealing at 800 °C contained a defect fluorite-type material, (Ca0.25Zr0.25Ti0.5)O1.75, along with zirconolite while only the defect fluorite-type phase was observed when an annealing temperature of 700 °C was used. The diffraction patterns showed that 900 °C is the lowest temperature at which zirconolite can be formed phase-pure by these methods. Rietveld refinement of zirconolite materials prepared by the coprecipitation and sol–gel methods showed very small structural changes when the material was annealed at high or low temperatures. Examination of the Zr K-edge spectra from these materials showed a very small change in the line shape of the spectra from materials prepared at 800 °C due to the similarity in the local environment of Zr in zirconolite and defect fluorite. A small change in the shape of the main edge feature of the Ti K-edge XANES spectra was observed for the materials annealed at 800 °C which can be attributed to the presence of Ti in a defect fluorite-type material. Ti K-edge GA-XANES spectra collected from the ion-implanted zirconolite materials showed that a small change in the structure of these materials occurred as a result of irradiation. The materials prepared by the coprecipitation and sol–gel methods (annealed at 1200 °C) showed nearly identical resistances to radiation damage compared to the material prepared by the ceramic method (annealed at 1400 °C). It has been shown in this study that zirconolite-type oxides can be synthesized at temperatures as low as 900 °C using either a coprecipitation or sol–gel method with very little change in the short- or long-range structure of the material and that the synthesis method used does not affect the radiation resistance of these materials. This work contributes towards the development of economically viable processes for the synthesis of zirconolite-type oxides for a range of applications, including as nuclear waste form materials.
Conflicts of interest
There are no conflicts to declare.
Acknowledgements
The Natural Sciences and Engineering Research Council (NSERC) of Canada funded this project through a discovery grant awarded to APG. SM thanks the University of Saskatchewan for financial support. SM acknowledges the receipt of support from the CLS Graduate and Post-Doctoral Student Travel Support Program. The Canadian Foundation for Innovation (CFI) is thanked for providing funds to purchase the PANalytical Empyrean powder X-ray diffractometer that was used in this project. Mr T. Bonli is thanked for collecting the SEM images. Dr J. Beam, Dr X. Guo, and Mr D. Holzscherer from the Department of Chemistry at the University of Saskatchewan are thanked for their help in the collection of the XANES spectra presented in this study. Dr Zou Finfrock and Dr Matthew Ward are thanked for their support in carrying out XANES experiments at 20 BM (CLS@APS). This research used resources of the Advanced Photon Source, an Office of Science User Facility operated for the U.S. Department of Energy (DOE) Office of Science by Argonne National Laboratory and was supported by the U.S. DOE under Contract No. DE-AC02-06CH11357, and the Canadian Light Source and its funding partners.
References
- R. C. Ewing, The Nuclear Fuel Cycle versus The Carbon Cycle, Can. Mineral., 2005, 43, 2099–2116 CrossRef CAS
.
-
Canadian Nuclear Association, The Canadian Nuclear Factbook, 2017 Search PubMed
.
-
E. R. Aluri and A. P. Grosvenor, A Review of X-Ray Absorption Near-Edge Spectroscopic Studies of Pyrochlore-Type Oxides Proposed for Nuclear Materials Applications, Elsevier Inc., 2016 Search PubMed
.
- G. R. Lumpkin, Alpha-Decay Damage and Aqueous Durability of Actinide Host Phases in Natural Systems, J. Nucl. Mater., 2001, 289, 136–166 CrossRef CAS
.
- R. C. Ewing, Ceramic Matrices for Plutonium Disposition, Prog. Nucl. Energy, 2007, 49, 635–643 CrossRef CAS
.
- G. R. Lumpkin, Ceramic Waste Forms for Actinides, Elements, 2006, 2, 365–372 CrossRef CAS
.
- L. Wang and T. Liang, Ceramics for High Level Radioactive Waste Solidification, J. Adv. Ceram., 2012, 1, 194–203 CrossRef CAS
.
- R. C. Ewing and W. Lutze, High-Level Nuclear Waste Immobilization with Ceramics, Ceram. Int., 1991, 17, 287–293 CrossRef CAS
.
- W. E. Lee, M. I. Ojovan, M. C. Stennett and N. C. Hyatt, Immobilisation of Radioactive Waste in Glasses, Glass Composite Materials and Ceramics, Adv. Appl. Ceram., 2006, 105, 3–12 CrossRef CAS
.
- H.-j Matzke, I. L. F. Ray, B. W. Seatonberry, H. Thiele, C. Trisoglio, C. T. Walker and T. J. White, Incorporation of Transuranic Elements in Titanate Nuclear Waste Ceramics, J. Am. Ceram. Soc., 1990, 73, 370–378 CrossRef CAS
.
- W. J. Weber, A. Navrotsky, S. Stefanovsky, E. R. Vance and E. Vernaz, Materials Science of High-Level Nuclear Waste Immobilization, MRS Bull., 2009, 34, 46–53 CrossRef CAS
.
- S. V. Stefanovsky, S. V. Yudintsev and T. S. Livshits, New Cubic Structure Compounds as Actinide Host Phases, IOP Conf. Ser.: Mater. Sci. Eng., 2010, 9, 1–12 Search PubMed
.
- I. Farnan, H. Cho and W. J. Weber, Quantification of Actinide A-Radiation Damage in Minerals and Ceramics, Nature, 2007, 445, 190–193 CrossRef CAS PubMed
.
- R. Devanathan and W. J. Weber, Radiation Effects in a Model Ceramic for Nuclear Waste Disposal, JOM, 2007, 59, 32–35 CrossRef CAS
.
- W. J. Weber, R. C. Ewing, C. R. A. Catlow, T. D. de la Rubia, L. W. Hobbs, C. Kinoshita, H. Matzke, A. T. Motta, M. Nastasi and E. K. H. Salje,
et al., Radiation Effects in Crystalline Ceramics for the Immobilization of High-Level Nuclear Waste and Plutonium, J. Mater. Res., 1998, 13, 1434–1484 CrossRef CAS
.
- C. Ewing and I. Headley, Alpha-Recoil Damage in Natural Zirconolite (CaZrTi2O7), J. Nucl. Mater., 1983, 119, 102–109 CrossRef
.
- W. J. Weber, J. W. Wald and H. Matzke, Effects of Self-Radiation Damage in Cm-Doped Gd2Ti2O7 and CaZrTi2O7, J. Nucl. Mater., 1986, 138, 196–209 CrossRef CAS
.
- M. W. A. Stewart, E. R. Vance, S. A. Moricca, D. R. Brew, C. Cheung, T. Eddowes and W. Bermudez, Immobilisation of Higher Activity Wastes from Nuclear Reactor Production of 99Mo, Sci. Technol. Nucl. Install., 2013, 1–16 Search PubMed
.
- J. V. Crum, L. Turo, B. Riley, M. Tang and A. Kossoy, Multi-Phase Glass-Ceramics as a Waste Form for Combined Fission Products: Alkalis, Alkaline Earths, Lanthanides, and Transition Metals, J. Am. Ceram. Soc., 2012, 95, 1297–1303 CrossRef CAS
.
- R. W. Cheary and A. A. Coelho, A Site Occupancy Analysis of Zirconolite CaZrxTi3−xO7, Phys. Chem. Miner., 1997, 24, 447–454 CrossRef CAS
.
- G. R. Lumpkin, R. C. Ewing, B. C. Chakoumakos, R. B. Greegor, F. W. Lytle, E. M. Foltyn, F. W. Clinard, L. A. Boatner and M. M. Abraham, Alpha-Recoil Damage in Zirconolite (CaZrTi2O7), J. Mater. Res. Technol., 1986, 1, 564–576 CrossRef CAS
.
- E. Paknahad and A. P. Grosvenor, Investigation of the Stability of Glass-Ceramic Composites Containing CeTi2O6 and CaZrTi2O7 after Ion Implantation, Solid State Sci., 2017, 74, 109–117 CrossRef CAS
.
- C. Meng, X. Ding, W. Li, J. Zhao and H. Yang, Phase Structure Evolution and Chemical Durability Studies of Ce-Doped Zirconolite – Pyrochlore Synroc for Radioactive Waste Storage, J. Mater. Sci., 2016, 51, 5207–5215 CrossRef CAS
.
- H. J. Rossell, Zirconolite-a Fluorite-Related Superstructure, Nature, 1980, 283, 282–283 CrossRef CAS
.
- E. R. Vance, G. R. Lumpkin, M. L. Carter, D. J. Cassidy, C. J. Ball, R. A. Day and B. D. Begg, Incorporation of Uranium in Zirconolite (CaZrTi2O7), J. Am. Ceram. Soc., 2004, 85, 1853–1859 CrossRef
.
- E. R. Vance, C. J. Ball, R. A. Day, K. L. Smith, M. G. Blackford, B. D. Begg and P. J. Angel, Actinide and Rare Earth Incorporation into Zirconolite, J. Alloys Compd., 1994, 213–214, 406–409 CrossRef
.
- B. S. K. Roberts, W. L. Bourcier and H. F. Shaw, Aqueous Dissolution Kinetics of Pyrochlore, Zirconolite and Brannerite at 25, 50, and 75 °C, Radiochim. Acta, 2000, 88, 539–543 Search PubMed
.
- I. Ebinumoliseh and A. P. Grosvenor, Effect of Synthetic Method and Annealing Temperature on the Structure of Hollandite-Type Oxides, Inorg. Chem., 2018, 57, 14353–14361 CrossRef CAS PubMed
.
- R. N. Bhowmik, Dielectric and Magnetic Study of BaTi0.5Mn0.5O3 Ceramics, Synthesized by Solid State Sintering, Mechanical Alloying and Chemical Routes, Ceram. Int., 2012, 38(6), 5069–5080 CrossRef CAS
.
-
M. Niederberger and N. Pinna, Aqueous and Nonaqueous Sol-Gel Chemistry, Metal Oxide Nanoparticles in Organic Solvents, Springer, London, 2009, pp. 7–19 Search PubMed
.
- A. E. Danks, S. R. Hall and Z. Schnepp, The Evolution of ‘Sol–gel’ Chemistry as a Technique for Materials Synthesis, Mater. Horiz., 2016, 3, 91–112 RSC
.
-
A. R. West, Solid State Chemistry and Its Applications, Wiley, 2nd edn, 2014 Search PubMed
.
- L. Kong, I. Karatchevtseva, D. J. Gregg, M. G. Blackford, R. Holmes and G. Triani, Gd2Zr2O7 and Nd2Zr2O7 Pyrochlore Prepared by Aqueous Chemical Synthesis, J. Eur. Ceram. Soc., 2013, 33, 3273–3285 CrossRef CAS
.
- L. Kong, I. Karatchevtseva, M. G. Blackford, N. Scales and G. Triani, Aqueous Chemical Synthesis of Ln2Sn2O7 Pyrochlore-Structured Ceramics, J. Am. Ceram. Soc., 2013, 96, 2994–3000 CrossRef CAS
.
- J. Nair, P. Nair, E. B. M. Doesburg, J. G. Van Ommen, J. R. H. Ross, A. J. Burggraaf and F. Mizukami, Preparation and Characterization of Lanthanum Zirconate, J. Mater. Sci., 1998, 33, 4517–4523 CrossRef CAS
.
- M. B. Moghadam, S. M. Zebarjad, J. S. Emampour and A. Youssefi, A Study on the Role of Ethylene Glycol/Alcohol Ratio on Synthesis of Nano-Size SnO2, Part. Sci. Technol., 2013, 31, 66–70 CrossRef CAS
.
- K. V. G. Kutty, R. Asuvathraman, R. R. Madhavan and H. Jena, Actinide Immobilization in Crystalline Matrix: A Study of Uranium Incorporation in Gadolinium Zirconate, J. Phys. Chem. Solids, 2005, 66, 596–601 CrossRef CAS
.
- K. Li, H. Wang and H. Yan, Hydrothermal Preparation and Photocatalytic Properties of Y2Sn2O7 nanocrystals, J. Mol. Catal. A: Chem., 2006, 249, 65–70 CrossRef CAS
.
- D. Chen and R. Xu, Hydrothermal Synthesis and Characterization of La2M2O7 (M = Ti, Zr) Powders, Mater. Res. Bull., 1998, 33, 409–417 CrossRef CAS
.
- R. C. Ewing, W. J. Weber and J. Lian, Nuclear Waste Disposal-Pyrochlore (A2B2O7): Nuclear Waste Form for the Immobilization of Plutonium and “Minor” Actinides, J. Appl. Phys., 2004, 95, 5949–5971 CrossRef CAS
.
- K. Koteswara Rao, T. Banu, M. Vithal, G. Y. S. K. Swamy and K. Ravi Kumar, Preparation and Characterization of Bulk and Nano Particles of La2Zr2O7 and Nd2Zr2O7 by Sol-Gel Method, Mater. Lett., 2002, 54, 205–210 CrossRef CAS
.
- A. Alemi and R. E. Kalan, Preparation and Characterization of Neodymium Tin Oxide Pyrochlore Nanocrystals by the Hydrothermal Method, Radiat. Eff. Defects Solids, 2008, 163, 229–236 CrossRef CAS
.
- W. J. Weber, Radiation and Thermal Ageing of Nuclear Waste Glass, Procedia Mater. Sci., 2014, 7, 237–246 CrossRef CAS
.
- R. C. Ewing, A. Meldrum, L. M. Wang and S. Wang, Radiation-Induced Amorphization, Rev. Mineral. Geochem., 2000, 39, 319–361 CrossRef CAS
.
- G. R. Lumpkin, K. L. Smith and M. G. Blackford, Heavy Ion Irradiation Studies of Columbite, Brannerite, and Pyrochlore Structure Types, J. Nucl. Mater., 2001, 289, 177–187 CrossRef CAS
.
- S. V. Yudintsev, A. A. Lizin, T. S. Livshits, S. V. Stefanovsky, S. V. Tomilin and R. C. Ewing, Ion-Beam Irradiation and 244Cm-Doping Investigations of the Radiation Response of Actinide-Bearing Crystalline Waste Forms, J. Mater. Res., 2015, 30, 1516–1528 CrossRef CAS
.
-
M. Tribet, S. Rolland, S. Peuget, V. Broudic, M. Magnin, T. Wiss and C. Jégou, Irradiation Impact on the Leaching Behavior of HLW Glasses, 2nd Int. Summer Sch. Nucl. Glas. Wasteform Struct. Prop. Long-Term Behav. SumGLASS 2013, 2014, vol. 7, pp. 209–215 Search PubMed
.
- M. Lang, C. L. Tracy, R. I. Palomares, F. Zhang, D. Severin, M. Bender, C. Trautmann, C. Park, V. B. Prakapenka and V. A. Skuratov,
et al., Characterization of Ion-Induced Radiation Effects in Nuclear Materials Using Synchrotron X-Ray Techniques, J. Mater. Res., 2015, 30, 1366–1379 CrossRef CAS
.
- H. S. Potdar, S. Vijayanand, K. K. Mohaideen, K. R. Patil, P. A. Joy, R. R. Madhavan, K. V. G. Kutty, R. D. Ambashta and P. K. Wattal, A Simple Chemical Co-Precipitation/Calcination Route for the Synthesis of Simulated Synroc-B and Synroc-C Powders, Mater. Chem. Phys., 2010, 123, 695–699 CrossRef CAS
.
-
J. F. Ziegler, J. P. Biersack and M. D. Ziegler, SRIM (The Stopping and Rang of Ions in Solids), 2013 Search PubMed
.
- H. H. Anderson, The Depth Resolution of Sputter Profiling, Appl. Phys., 1979, 18, 131–140 Search PubMed
.
- X-ray Attenuation Length http://henke.lbl.gov/optical_constants/atten2.html, accessed Mar 26, 2018.
- S. M. Heald, D. L. Brewe, E. A. Stern, K. H. Kim, F. C. Brown, D. T. Jiang, E. D. Crozier and R. A. Gordon, XAFS and Micro-XAFS at the PNC-CAT Beamlines, J. Synchrotron Radiat., 1999, 6, 347–349 CrossRef CAS PubMed
.
- B. Ravel and M. Newville, Athena, Artemis, Hephaestus: Data Analysis for X-Ray Absorption Spectroscopy Using IFEFFIT, J. Synchrotron Radiat., 2005, 12, 537–541 CrossRef CAS PubMed
.
- T. Yamamoto, Assignment of Pre-Edge Peaks in K-Edge X-Ray Absorption Spectra of 3d Transition Metal Compounds: Electric Dipole or Quadrupole?, X-Ray Spectrom., 2008, 37, 572–584 CrossRef CAS
.
- G. Mountjoy, D. M. Pickup, R. Anderson, G. W. Wallidge, M. A. Holland, R. J. Newport and M. E. Smith, Phys. Chem. Chem. Phys., 2000, 2, 2455–2460 RSC
.
- M. W. Gaultois and A. P. Grosvenor, Phys. Chem. Chem. Phys., 2012, 14, 205–217 RSC
.
- R. B. Greegor, K. Y. Blohowiak, J. H. Osborne, K. A. Krienke, J. T. Cherian and F. W. Lytle, X-Ray Spectroscopic Investigation of the Zr-Site in Thin Film Sol-Gel Surface Preparations, J. Sol-Gel Sci. Technol., 2001, 20, 35–50 CrossRef CAS
.
- E. R. Aluri and A. P. Grosvenor, An Investigation of the Electronic Structure and Structural Stability of RE2Ti2O7 by Glancing Angle and Total Electron Yield XANES, J. Alloys Compd., 2014, 616, 516–526 CrossRef CAS
.
Footnote |
† Electronic supplementary information (ESI) available. See DOI: 10.1039/c8tc04560g |
|
This journal is © The Royal Society of Chemistry 2019 |
Click here to see how this site uses Cookies. View our privacy policy here.