DOI:
10.1039/C9AN01629E
(Paper)
Analyst, 2020,
145, 213-222
Two isomeric and distinguishable H2S fluorescence probes for monitoring spoilage of eggs and visualizing exogenous and endogenous H2S in living cells†
Received
23rd August 2019
, Accepted 30th October 2019
First published on 5th November 2019
Abstract
Accurate fabrication of fluorescence probes to efficiently monitor and detect H2S levels in the fields of foodstuffs and physiology is crucial. Herein, we report two isomeric phenanthro[9,10-d]imidazole benzene sulfonamide-derived fluorescence probes (PI-2-SA and PI-4-SA), both of which display remarkable responses toward H2S over other analytes. The spectral characteristics of the two probes were investigated and are discussed in detail. By comparison, PI-2-SA was specific, sensitive (the limit of detection was ca. 12.3 nM), rapid (within ≤3 min) and dynamic (the rate constant was 0.02 s−1). Significantly, PI-2-SA was proved to be effective in monitoring the shelf-time progress of egg samples in real time as well as in the imaging of exogenous and endogenous H2S in HeLa cells.
Introduction
Recently, hydrogen sulfide (H2S) has emerged as an endogenous gaseous transmitter of signaling molecules because it can be automatically generated in cells from cysteine (Cys) and cysteine derivatives with enzymatic assistance. At a normal level, H2S has positive functions in physiological and pathological processes, such as transmitting intracellular signals,1 improving the utilization rate of oxygen by the body,2 regulating cardiovascular and immune systems,3 and relieving the burden of vascular smooth muscle.4 On the other hand, H2S is an odorous and toxic compound. Aberrant production of H2S in the human body has been proved to be connected with many diseases, such as ischemic disease,5 atherosclerosis,6 diabetes,7 Down syndrome,8 and Alzheimer's disease.9 Furthermore, H2S has displayed many advantages in the field of foodstuffs.10,11 Organosulfur-rich foods, including fumigated bananas, vinegar, grapes, and lychee, can endogenously release certain amounts of H2S; this can not only contribute to human health but can also prolong the storage time of postharvest foods. However, during sulfur-rich food spoilage, which is a naturally occurring process leading to undesirable modifications in sensory characteristics, excessive H2S is released as a prominent volatile due to yeast fermentation caused by the accumulation of various sulfides.12–14 In this case, H2S has deleterious effects and causes sensory changes. For example, excessive H2S may be detrimental to the flavors of vinegar and wine. Hence, the amount of released H2S may be a vital indicator when evaluating the fresh-like quality of foods. Consequently, in view of the bio-function of H2S in cells and its key effects in food safety and food quality, it is necessary to accurately monitor H2S levels in living organisms and food samples.
At present, new strategies are rapidly emerging for H2S detection, including sulfide-selective electrodes,15,16 chromatography,17,18 capillary electrophoresis,19 colorimetry20 and fluorescence probes.21 Among these methods, fluorescence probes have received considerable attention due to their high sensitivity and selectivity, facile operation, convenient observation and real-time detection.22–24 Especially in the aspect of fluorescent biological imaging, noninvasive fluorescence probes have become an attractive approach to achieve real-time monitoring of the levels of exogenic or endogenic H2S with high spatiotemporal resolution for sensing and imaging in biological samples. Accordingly, many fluorescence probes have been designed and obtained based on various strategies.25–30 As a general rule, these probes mainly rely on nucleophilic substitution or addition reactions of H2S to promote changes in fluorescence response. Although the reactivity and response characteristics of various fluorescence probes to H2S have been discussed individually, detailed research about the similarities and differences of the responses of two isomeric fluorescence probes to H2S based on the same strategy is limited. Moreover, another critical bottleneck encountered with the development of H2S fluorescence probes is their limited application in real-time detection.
Hence, to address the problems enumerated above, we developed two isomeric fluorescence probes based on a phenanthro[9,10-d]imidazole platform for exhibition of excellent response toward H2S via the substitution reaction of benzene sulfonamide that was interposed into the phenanthro[9,10-d]imidazole platform as a leaving group in different positions. The similarities and differences of the responses of the two probes were discussed in detail by means of absorption and emission spectra. In contrast, PI-2-SA, which possesses closer steric distance between the illuminophore and the leaving group, exhibited a better response rate to H2S than PI-4-SA, in which the leaving group is far from the illuminophore. More importantly, fluorescence probe PI-2-SA as an indicator can be used successfully to monitor the spoilage process of egg samples in real time. Significantly, PI-2-SA can detect exogenously and endogenously produced H2S by enhancement of its fluorescence signal in living cells.
Experimental
Apparatus and reagents
Melting points were estimated on an X4 melting apparatus without correction. IR spectra were recorded as KBr disks with a PerkinElmer Spectrum One FT–IR spectrometer. 1H and 13C NMR spectra were determined using Bruker 600 and 150 MHz spectrometers, respectively, using DMSO as the solvent and TMS as an internal standard. Electrospray ionization mass spectra (ESI-MS) were collected on a Xevo G2-XS QTof instrument. All pH measurements were performed with a Sartorius PB-10 digital pH-meter (Shanghai Precision Scientific Instrument Co., Ltd, Shanghai, China). Absorption spectra were recorded on a TU-1901 UV–vis spectrophotometer. Fluorescence measurements were recorded on a PerkinElmer LS55 luminescence spectrometer, and fluorescence imaging was recorded under an Olympus CKX41-A32RC confocal laser scanning microscope.
Phenanthrene-9,1-dione, propargylglycine (PAG), cysteine (Cys), and 4-nitrobenzaldehyde were purchased from Innochem Co., Ltd (Beijing, China). Other agents, metal salts, and solvents were obtained from local agent shops. The solvents used for the reactions were analytical grade, and the solvents used for the spectral analysis were spectral grade. No agents or solvents were further treated before use. Water used in the experiments was distilled twice.
Synthesis and characterization of PI-2-SA and PI-4-SA
Compounds PI-2-A and PI-4-A were prepared according to a procedure reported in the literature.31 A solution of 2,4-dinitrobenzenesulfonyl chloride (797 mg, 3.0 mmol) in dichloromethane (DCM, 10 mL) was slowly added dropwise to a solution of compound PI-2-A or PI-4-A (385 mg, 1.0 mmol) and triethyl amine (0.5 mL) in DCM (10 mL). The above mixture was stirred at 0 °C for 30 min and then at room temperature overnight. After the completion of the reaction (monitored by TLC), the mixture was slowly poured into ice water (50 mL), followed by the addition of saturated NaCl solution. Then, the DCM phase was collected, dried over anhydrous Na2SO4, and then concentrated. The residue was further purified by flash column chromatography to afford pure PI-2-SA and PI-4-SA as orange solids.
PI-2-SA, yield 50%, Mp > 300 °C. 1H NMR (600 MHz DMSO) δ (ppm): 8.94 (d, J = 6.0 Hz, 1H), 8.89 (d, J = 6.0 Hz, 1H), 8.63 (s, 1H), 8.52 (d, J = 8.4 Hz, 1H), 8.36 (s, 2H), 7.78 (t, J = 7.2 Hz, 1H), 7.72 (t, J = 7.2 Hz, 1H), 7.57–7.66 (m, 6H), 7.47 (d, J = 8.4 Hz, 1H), 7.33–7.39(m, 2H), 7.18 (d, J = 7.2 Hz, 1H), 7.02–7.07 (m, 2H). 13C NMR (150 MHz, DMSO) δ (ppm) 150.58, 150.08, 148.27, 138.31, 136.85, 136.64, 136.55, 132.12, 131.92, 130.81, 130.71, 129.81, 129.46, 129.06, 128.40, 128.19, 128.00, 126.69, 127.15, 127.09, 126.33, 126.13, 125.83, 125.00, 124.19, 122.89, 122.40, 121.92, 120.94, 120.69. HRMS (ESI): calcd for [M − H]− 614.1133, found 614.1135.
PI-4-SA, yield 45%, Mp > 300 °C. 1H NMR (600 MHz DMSO) δ (ppm) 11.29 (s, 1H), 8.91 (s, 1H), 8.91–8.92 (m, 2H), 8.87 (d, J = 8.4 Hz, 1H), 8.62 (d, J = 7.8 Hz, 1H), 8.60 (d, J = 7.8 Hz, 1H), 8.24 (d, J = 9.0 Hz, 1H), 7.77 (t, J = 7.8 Hz, 1H), 7.65–7.78 (m, 6H), 7.55 (t, J = 7.2 Hz, 1H), 7.50 (d, J = 9.0 Hz, 2H), 7.33 (t, J = 7.8 Hz, 1H), 7.10 (d, J = 9.0 Hz, 1H), 7.05 (d, J = 8.4 Hz, 1H). 13C NMR (150 MHz, DMSO) δ (ppm) 149.95, 147.81, 137.97, 136.21, 131.96, 131.60, 130.86, 130.62, 129.32, 129.05, 128.14, 128.00, 127.79, 127.68, 127.24, 126.66, 126.48, 126.05, 125.06, 124.22, 123.42, 122.66, 122.38, 120.81, 120.69. HRMS (ESI): calcd for [M + H]+ 616.1291, found 616.1278.
Detection of H2S in egg samples
Chicken, duck and quail eggs with similar sizes and colors were purchased from a local supermarket, respectively. All eggs were stored in an incubator (STIK (Shanghai) CO., China) at 25 °C and 50% relative humidity. Every 2 days, three groups of eggs were removed from the incubator for experiments up to 12 days. Prior to the experiment, the egg was firstly peeled, quickly poured into a 100 mL glass breaker and then sealed with plastic wrap. 20 μL of egg white sample was extracted with a syringe and injected into 5 mL DMF solution of the sensor (100 μM). Then, the mixed solution was diluted to 10 mL with 10 mM Hepes buffer (pH 7.4). Finally, the fluorescence intensity of the DMF/H2O solution containing egg white and the sensor was recorded at 424 nm for each sample as a response feature of the sensor to the H2S released from the egg. Each test was repeated three times.
Cell culture and cytotoxicity assay
The cell culture and cytotoxicity assay were performed according to our previous report.32 HeLa cells were cultured and seeded in Dulbecco's modified Eagle's medium (DMEM) in an atmosphere of 5% CO2 and 95% air at 37 °C for 24 h, followed by the addition of 0 to 50 μM PI-2-SA. The HeLa cells were seeded in a 12-well plate for 24 h. Finally, after the addition of 20 mL 3-(4,5-dimethylthiazol-2-yl)-2,5-diphenyltetrazolium bromide (MTT), the cells were cultured for another 4 h. Cells were seeded on a culture dish for fluorescence microscopic imaging by an inversion fluorescence microscope.
Imaging of exogenous and endogenous H2S in HeLa cells
Firstly, HeLa cells were seeded in a laser confocal dish, and the cells were cultured for 24 h for the fluorescence imaging experiments. After incubation for 24 h, the sensor (10 μM) was added to the original cell culture dish for 1 h, and 50 μM NaHS was then added to the original cell culture dish for another 1 h. To monitor endogenous H2S, the other group of HeLa cells were processed with the same procedure but with addition of only Cys (1 mM), Cys (1 mM) and PAG (1 mM), respectively. Additionally, there was no need for addition of NaHS in the monitoring of endogenous H2S. Fluorescence images of exogenous and endogenous H2S were photographed under an Olympus CKX41-A32RC confocal laser scanning microscope.
Results and discussion
Design and synthesis of two isomeric sensors for H2S
In this work, we aimed to develop two isomeric sensors which could respond to H2S with distinguishable fluorescence efficiencies. Therefore, two 1-phenyl-1H-phenanthro[9,10-d]imidazole derivatives with 2,4-dinitrobenzene sulfonamide groups were constructed, in which a 2,4-dinitrobenzene sulfonyl group as the leaving group was introduced into 1-phenyl-1H-phenanthro[9,10-d]imidazol-2-yl benzenamine at two different leaving sites; that is, only the position of the leaving group differed. Benzene sulfonamide has been known to efficiently react with H2S by thiolysis to afford amino derivatives and phenthiol along with release of SO2. Simultaneously, a dinitro group was designed in the phenyl ring subunit to enhance the leaving reactivity of benzene sulfonamide with H2S. Furthermore, when the illuminophore 1-phenyl-1H-phenanthro[9,10-d]imidazole and the leaving group 2,4-dinitrobenzene sulfonamide were forced close to each other, the 2,4-dinitrobenzene sulfonamide moiety could more easily react with H2S due to considerable steric action. Combined with the above reasons, we designed and synthesized two isomeric fluorescence sensors that could detect H2S at different fluorescence efficiencies. The synthetic method of the fluorescence sensors PI-2-SA and PI-4-SA is shown in Scheme 1. The structures of PI-2-SA and PI-4-SA were fully characterized and determined by 1H NMR, 13C NMR, and ESI-MS spectroscopy (Fig. S1–S6†).
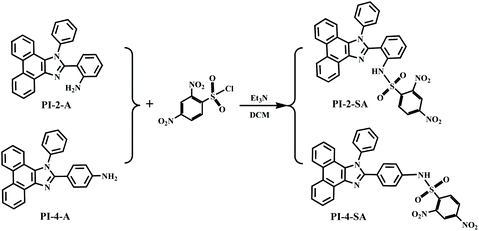 |
| Scheme 1 Synthetic method of PI-2-SA and PI-4-SA. | |
Spectral response of the two sensors to H2S
Initially, the absorption and emission spectra of free PI-2-SA and PI-4-SA (10 μM) were examined in DMF/H2O solution (v/v = 1/1, Hepes 10 μM, pH 7.4). As shown in Fig. 1a and b, two significant absorption peaks at around 340 and 355 nm for free PI-2-SA were observed, and only a slight bulge was observed at 360 nm for PI-4-SA. They showed almost no obvious absorption above 400 nm. However, outstanding absorption peaks occurred at around 440 nm for PI-2-SA and 450 nm for PI-4-SA (Fig. 1a and b), respectively, with the addition of NaHS (50 μM) (a commonly used H2S source) to the solutions of PI-2-SA and PI-4-S; this was responsible for the distinct color change from colorless to yellow (Fig. 1a inset and Fig. 1b inset). The above varying of the absorption spectra and the naked-eye-visible changes may be caused by the transformation of the sulfonamide to an amino group. This transformation of the functional group also led to distinct changes in the fluorescence spectra of PI-2-SA and PI-4-SA after treatment with H2S. In DMF/H2O (v/v = 1/1, Hepes 10 μM, pH 7.4) solution, when excited at 310 and 329 nm, both PI-2-SA and PI-4-SA displayed negligible fluorescence intensity, with fluorescence quantum yields of 0.026 (ΦPI-2-SA) and 0.007 (ΦPI-4-SA), respectively; this may be due to the photoinduced electron transfer (PET) effect from the full charge of the phenanthro[9,10-d]imidazole moiety to the electron-withdrawing sulfonamide group. Meanwhile, the addition of H2S (50 μM) elicited a dramatic increase in the fluorescence emissions of PI-2-SA and PI-4-SA at 425 and 450 nm (Fig. 1c and d) with 19-fold (ΦPI-2-SA = 0.283) and 36-fold (ΦPI-4-SA = 0.332) enhancements, respectively. The fluorescence enhancing phenomenon indicated that the original PET process in free PI-2-SA and PI-4-SA did not occur, which indicates the disappearance of the electron-withdrawing sulfonamide as the leaving group because of the response of the sensors to H2S (Fig. 1 inset).
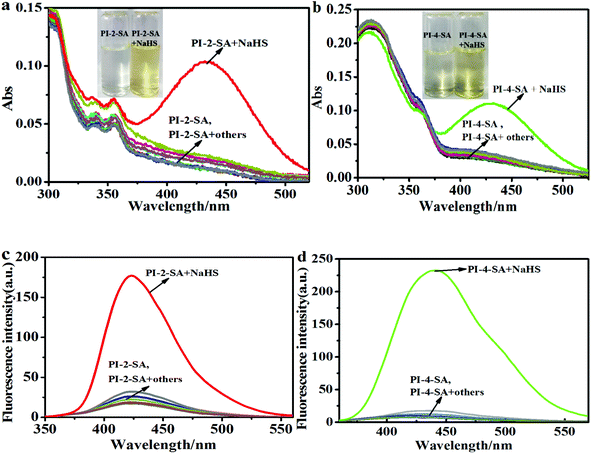 |
| Fig. 1 Absorption (a) (b) and fluorescence (c) (d) spectra of PI-2-SA (10 μM, λex = 310 nm) and PI-4-SA (10 μM, λex = 329 nm) upon the addition of various species in DMF/H2O solution (v/v = 1/1, Hepes 10 μM, pH 7.4). | |
To further understand the sensing mechanism, a solution of PI-2-SA treated with NaHS was subjected to thin layer chromatography (TLC) and mass spectrum analysis. TLC analysis clearly showed an additional fluorescence spot on the plate (Fig. S7a†), which was attributed to PI-2-A and showed a comparable Rf value to the PI-2-A sample. Therefore, we inferred that the thiolysis reaction occurred, which led to the formation of the corresponding amino product. Accordingly, the ESI-MS analysis for the reaction mixture provided clear evidence for the above inference. One peak at m/z 386.1643 for PI-2-A (M + H)+ and another at m/z 286.0004 for 2,4-dinitro phenthiol (M + Na+ + NO3− + H+)+ were observed (Fig. S8†). The same operations were carried out for the reactive products of PI-4-SA with NaHS, and similar results were also noted in the TLC analysis (Fig. S7b†) as well as the ESI-MS analysis (m/z 386.1643 for PI-2-A (M + H)+; m/z 223.0451 for 2,4-dinitro phenthiol (M + Na)+) (Fig. S9†). The above results show that PI-2-SA and PI-4-SA were partially transformed into the corresponding amino products PI-2-A and PI-4-A as well as 2,4-dinitro phenthiol. According to the above analysis, a possible sensing mechanism was proposed, as depicted in Scheme 2. In this case, we continued to evaluate the differences in the sensibility and efficiency of the two sensors to detect H2S. To compare the sensibility, titration experiments of H2S for the two sensors were firstly performed under the same experimental conditions (DMF/H2O solution, v/v = 1/1, Hepes 10 μM, pH 7.4) and monitored by absorption and emission spectra, respectively. As shown in Fig. S10a,† the absorbance intensity of PI-2-SA enhanced gradually with increasing H2S concentration and reached a stable plateau at 50 μM NaHS; this reflected a reactive equilibrium of PI-2-SA and its etherification products in the presence of an appropriate amount of H2S. A similar plateau for the absorption intensity of PI-4-SA was also formed at an NaHS concentration of 0.25 mM (Fig. S10b†). It is obvious that the equilibrium concentration of PI-2-SA with H2S was much lower than that of PI-4-SA with H2S; the lower the concentration, the more readily the reaction occurs. Meanwhile, the results from the absorption spectra primarily confirmed that the response of PI-2-SA to H2S was more sensitive than that of PI-4-SA.
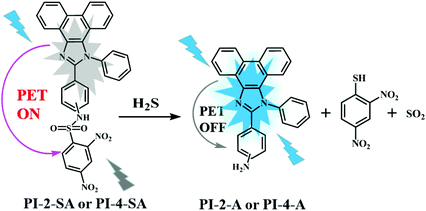 |
| Scheme 2 Proposed mechanism for PI-2-SA with H2S. | |
To further distinguish one sensor from the other, the fluorescence spectra of PI-2-SA and PI-4-SA mixed with different concentrations of H2S were also individually assessed in DMF/H2O solution (v/v = 1/1, Hepes 10 μM, pH 7.4). Gradual enhancements of the fluorescence intensities of the two sensors were realized by incubation with different concentrations of H2S (Fig. 2a and c). Similar to the absorption spectra, the NaHS concentrations at which the fluorescence plateau was reached for PI-2-SA (50 μM) and PI-4-SA (0.25 mM) were evidently different from each other. The results of the titration experiments indicated that PI-2-SA reacts much more readily with H2S. A good linear calibration graph of PI-2-SA (R2 = 0.9956) with the concentration of NaHS in the range of 0 to 0.9 μM was measured (Fig. 2b), and the limit of detection (LOD) was calculated to be as low as 12.3 nM based on LOD = 3SD/B, as defined by IUPAC.33 For PI-4-SA, a good linear relationship (R2 = 0.9972) was observed (Fig. 2d), with a still-nanomolar LOD of 14.72 nM. The LOD values for PI-2-SA and PI-4-SA were compatible with physiological H2S levels, and PI-2-SA appeared to be more sensitive for mapping of H2S compared with PI-4-SA (Fig. 2 inset).34–36
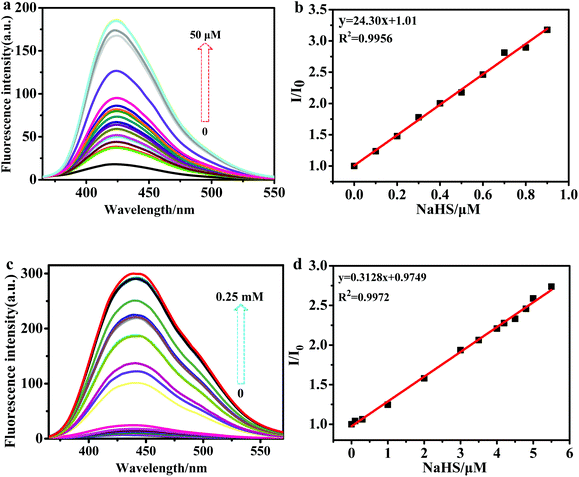 |
| Fig. 2 (a) Fluorescence spectra of PI-2-SA (10 μM) with various amounts of H2S (0 to 50 μM); (b) plot of the changes in the fluorescence intensity of PI-2-SA with H2S ranging from 0 to 0.9 μM; (c) fluorescence spectra of PI-4-SA (10 μM) with various amounts of H2S (0 to 0.25 mM); (d) plot of the changes in the fluorescence intensity of PI-2-SA with H2S ranging from 0 to 5.5 μM. | |
Subsequently, the time-response studies of the two sensors to H2S were carried out by monitoring the fluorescence emission ratio. It was found that the fluorescence emission ratio (I − Imax/Imax) at 420 nm of PI-2-SA to H2S quickly increased during the initial reaction and reached equilibrium at 200 s (Fig. 3a); that is to say, the response of PI-2-SA to H2S occurred within ≤3 min. This fast response is considered beneficial for the application of a probe to test H2S concentrations in real time. Meanwhile, the fluorescence emission ratio (I − Imax/Imax) at 450 nm of PI-4-SA to H2S slowly and continuously increased, and the detection process continued until about 80 min (Fig. 3b). Finally, 60 min was the time found to achieve reaction equilibrium for PI-4-SA toward H2S. It is obvious that the reaction time for PI-2-SA toward H2S was much shorter than that of PI-4-SA; this is mainly due to the steric hindrance created between the sulfonamide group and adjacent phenanthro[9,10-d]imidazole, due to which the sulfonamide group is a much better leaving group (Fig. 3 inset). Furthermore, the fluorescence intensity changes of PI-2-SA and PI-4-SA before and after the addition of NaHS were considered. As shown in Fig. 4 within 240 min, the individual fluorescence intensities remained fundamentally stable for both free PI-2/4-SA and PI-2/4-SA + NaHS; this illustrates that the two probes could sense NaHS with chemical and photic stability in the tested time.
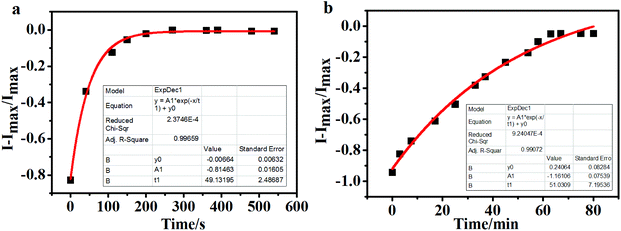 |
| Fig. 3 The kinetic study of the responses of PI-2-SA (10 μM, λem = 420 nm) (a) and PI-4-SA (10 μM, λem = 450 nm) (b) to H2S under pseudo-first-order conditions in DMF/H2O solution (v/v = 1/1, Hepes 10 μM, pH 7.4). | |
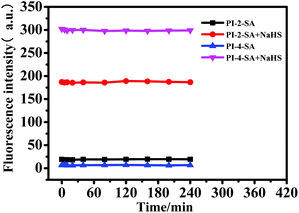 |
| Fig. 4 Fluorescence intensities of PI-2-SA and PI-4-SA before and after the addition of NaHS in DMF/H2O solution (v/v = 1/1, Hepes 10 μM, pH 7.4). | |
In addition, the rate constant k is a fundamental characteristic of a reaction. If a reaction is fast, it has a large rate constant, and vice versa. Therefore, according to the quasi-first-order dynamic equation It = Imax + A
exp(kt),37,38 the reactive rate constants kPI-2-SA and kPI-4-SA were estimated to be 0.02 s−1 and 0.02 min−1, respectively, which are comparable with those of reported H2S sensors.39 More importantly, the obtained kPI-2-SA value was much larger than kPI-4-SA, representing the higher sensitivity of PI-2-SA toward H2S; this proves that PI-2-SA is the more susceptible tool for H2S detection presented in this paper.
Sensing selectivity and effects of pH
Of course, the effects of other analytes on the responses of the two sensors to H2S were also considered. The fluorescence spectra of PI-2-SA and PI-4-SA in the coexistence of H2S with other analytes, including GSH, L-Cys, Hcy, and some anions, are recorded in Fig. S11.† The phenomena of fluorescence enhancement for PI-2-SA (10 μM) and PI-4-SA (10 μM) were retained in the presence of excess analyte with a mole ratio of 1
:
2 between NaHS and the analyte. As observed above, both PI-2-SA and PI-4-SA can selectively respond to H2S with significant fluorescence enhancements without disturbances caused by various analytes, including excess biothiols.
Moreover, the effects of pH on H2S detection were investigated for PI-2-SA and PI-4-SA in the absence and presence of NaHS (Fig. 5). Without NaHS, both PI-2-SA and PI-4-SA were quite stable over a wide pH range of 2 to 11. However, in the presence of NaHS, PI-2-SA and PI-4-SA showed significant fluorescence turn-on at 420 and 450 nm in the range of pH 5 to 9, respectively, within which the majority of biological samples (5.25 to 8.93) can be detected. These results imply that PI-2-SA and PI-4-SA can provide increasing fluorescence signals, allowing H2S detection in the main physiological pH range; this is highly favorable for their biological applications (Fig. 5 inset).
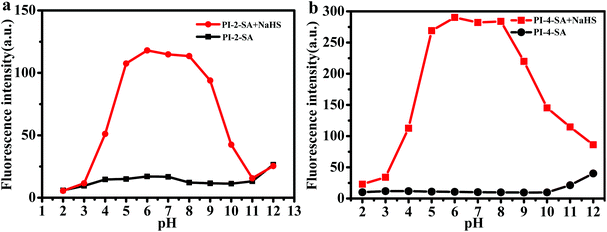 |
| Fig. 5 Fluorescence intensity changes of PI-2-SA (10 μM, λem = 420 nm) (a) and PI-4-SA (10 μM, λem = 450 nm) (b) in the absence and presence of H2S at different pH values in DMF/H2O solution (v/v = 1/1, Hepes 10 μM). | |
Surveying the fresh-like quality of eggs
As is well known, spoiling eggs can produce H2S due to the decomposition of nutrients such as proteins, lipids, and carbohydrates.40,41 Therefore, it is highly meaningful to determine the freshness and spoiling process of eggs in an efficient and nondestructive way. In this paper, PI-2-SA, as an example, was used to further explore its application properties. The releasing process of H2S from three different kinds egg samples (chicken eggs, duck eggs, and quail eggs) was investigated to survey the shelf-life extension of eggs. Three groups of eggs stored for 0 day, 2 days, 4 days, 6 days, 8 days, and 12 days, respectively, were tested. The fluorescence intensities of PI-2-SA were selected as an indicator to characterize the whole spoiling processes of the different eggs. Three groups of fluorescence intensity data for free PI-2-SA, eggs, and PI-2-SA injected with eggs stored for different storage times were recorded by histograms, respectively, as described in Fig. 6a–c. The fluorescence emissions for the three types of eggs were all negligible for the fresh as well as the spoiled samples. However, after the three types of eggs were stored for 4 days, distinct differences in the emission intensities between PI-2-SA and PI-2-SA-eggs began to be noted, which signifies that the eggs were slightly spoiled and released some amount of H2S. After that, with increasing storage time, the fluorescence intensities of PI-2-SA-eggs were significantly enhanced, indicating that the degree of metamorphism of the eggs deepened and the released quantity of H2S increased. By comparing the fluorescence intensities of PI-2-SA-eggs with those of PI-2-SA titrated by NaHS concentration (Fig. 2a), the response of PI-2-SA to spoiling chicken eggs stored for 12 days was approximately equivalent to the response to 0.5 μM NaHS solution. Additionally, with increased storage time, the fluorescence color changes of the PI-2-SA-eggs solutions were easily observed from cloudy and colorless to bright blue under a 365 nm UV lamp (Fig. 6d). The above evidence suggests that PI-2-SA can be conveniently employed as a visual indicator for inspecting the fresh-like quality and monitoring the spoiling process of eggs without any laboratory instrumentation (Fig. 6 inset).
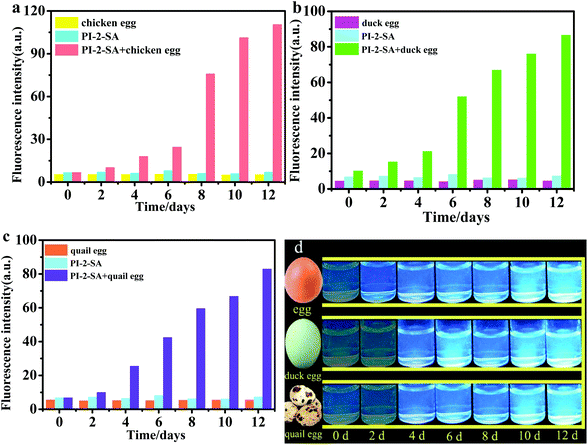 |
| Fig. 6 Histograms of the independent fluorescence intensities (λem = 420 nm) of eggs, free PI-2-SA (10 μM), and PI-2-SA-eggs for different storage times in DMF/H2O solution (v/v = 1/1, Hepes 10 μM, pH 7.4) (a: chicken egg; b: duck egg; c: quail egg). (d) Photograph of PI-2-SA solution (10 μM) with the addition of three types of eggs stored for different times (0 days, 2 days, 4 days, 6 days, 8 days, 10 days and 12 days) under 365 nm UV light. | |
Fluorescence imaging of exogenic and endogenic H2S in living cells
The results of the spectral response of PI-2-SA and PI-4-SA toward H2S showed that these two sensors could detect H2S with good selectivity and sensitivity. Moreover, PI-2-SA could be used as an indicator to inspect the fresh-like quality and monitor the spoiling process of eggs. Considering the bio-application prospects of these two sensors, we continued to evaluate the capacities of PI-2-SA to image exogenous and endogenous H2S in living systems. Prior to cell imaging, the cytotoxicity and compatibility of PI-2-SA were tested by the standard MTT assay using HeLa cells. PI-2-SA with various concentrations from 0 to 50 μM displayed low cytotoxicity and excellent biocompatibility for cultured cell lines under the experimental conditions for 6 h (Fig. S12†), which indicates that PI-2-SA is suitable for imaging H2S in living cells. First, the fluorescence imaging response of PI-2-SA to exogenous H2S in HeLa cells was considered by the incubation of PI-2-SA-stained HeLa cells with 50 μM NaHS. In Fig. 7b, in HeLa cells only treated with PI-2-SA, an extremely faint fluorescence signal was detected. However, relatively strong blue fluorescence appeared when PI-2-SA-loaded HeLa cells were further handled with NaHS (Fig. 7e). The enhancement of the blue signal implies that PI-2-SA can be employed to image intracellular H2S in living cells. Subsequently, we further checked the identification ability of PI-2-SA for endogenic H2S in living cells. According to previous literature reports,42–44 cysteine as a precursor can produce more endogenous biosynthesis of H2S inside the cells. When HeLa cells were pretreated with cysteine (1 mM), incubated for 1 h, and then treated with PI-2-SA (10 μM), a distinct blue fluorescence signal was collected (Fig. 7h), which was clearly discriminative of HeLa cells without cysteine stimulation (Fig. 7b). The enhancement phenomenon of the fluorescence signal may be caused by the response of PI-2-SA to endogenous H2S generated in HeLa cells. In order to confirm this, HeLa cells stained with PI-2-SA were simultaneously treated with H2S precursor (cysteine, 1 mM) and H2S inhibitor (propargylglycine PAG, 1 mM);45–49 the corresponding fluorescence images are provided in Fig. 7k. In this case, the blue fluorescence signal was almost suppressed, which verifies that the addition of cysteine was no longer valid and that the production of endogenous H2S was inhibited in presence of the PAG. All the data suggest that PI-2-SA is capable of fluorescence imaging of both exogenic and endogenic H2S in living cells (Fig. 7 inset).
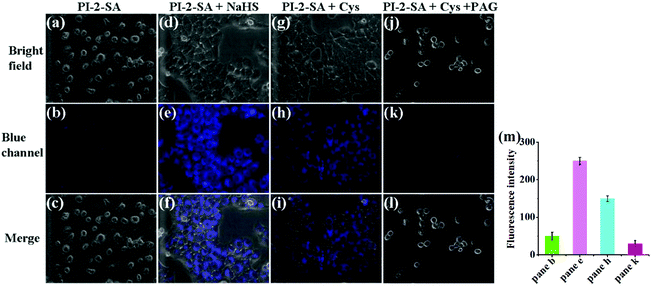 |
| Fig. 7 Fluorescence images of H2S in HeLa cells using PI-2-SA. (a–c) Bright field (a), blue channel (b) and merged image (c) of HeLa cells incubated with PI-2-SA only (10 μM) for 30 min. (d–f) Bright field (d), blue channel (e) and merged image (f) of HeLa cells incubated with PI-2-SA (10 μM) for 30 min and then with added NaHS (50 μM) for another 30 min. (g–i) Bright field (g), blue channel (h) and merged image (i) of HeLa cells stimulated with cysteine (100 μM) and then incubated with PI-2-SA (10 μM) for another 1 h. (j–h) Bright field (j), blue channel (k) and merged image (l) of HeLa cells stimulated with cysteine (1 mM) and PAG (1 mM) and then incubated with PI-2-SA (10 μM) for another 1 h. (m) Average intensities from images (b), (e), (h), and (k). | |
Conclusions
In this work, we successfully designed and synthesized two isomeric and distinguishable fluorescence probes based on phenanthro[9,10-d]imidazole derivatives modified by benzene sulfonamide groups in two different positions. The fluorescence intensities of the two probes were significantly trigged by the nucleophilic substitution reaction of the benzene sulfonamide group driven by H2S, which proved that two isomeric fluorescence probes could selectively detect H2S. Notably, PI-2-SA, which retained closer steric distance between the phenanthro[9,10-d]imidazole moiety and the benzene sulfonamide group, displayed excellent H2S detection efficiency with high sensitivity (LOD of 12.3 nM), rapid response (less than 3 min), and good dynamic driving (rate constant of 0.02 s−1). PI-2-SA was successfully used as a fluorescence indicator for H2S in the supervision of the spoilage process of egg samples in real time. Furthermore, it can be used to visualize exogenous and endogenous H2S in HeLa cells by fluorescence turn-on signals. Thus, we believe that probes PI-2-SA and PI-4-SA are distinguishable during the detection of H2S, and PI-2-SA can be applied as a more effective tool in foodstuffs and philological fields.
Conflicts of interest
All authors have declared that no competing interest exists.
Acknowledgements
The work was supported by the National Natural Science Foundation of China (21506106), the Natural Science Foundation of Heilongjiang Province (LC2017004), the Heilongjiang Province Leading Talent Echelon Reserve Leader Fund, and the Qiqihar University Graduate Innovation Fund Grants (YJSCX2018-ZD18).
Notes and references
- V. S. Lin and C. J. Chang, Curr. Opin. Chem. Biol., 2012, 16, 5–6 Search PubMed.
- J. W. Calvert, S. Jha, S. Gundewar, J. W. Elrod, A. Ramachandran, C. B. Pattillo, C. G. Kevil and D. J. Lefer, Circ. Res., 2009, 105, 365–374 CrossRef CAS.
- W. M. Xuan, C. Q. Sheng, Y. T. Cao, W. H. He and W. Wang, Angew. Chem., Int. Ed., 2012, 51, 2282–2284 CrossRef CAS.
- T. Cao, Z. D. Teng, D. Y. Gong, J. Qian, W. Liu, K. Iqbal, W. W. Qin and H. C. Guo, Talanta, 2019, 198, 185–192 CrossRef CAS.
- S. Fiorucci, E. Antonelli, A. Mencarelli, S. Orlandi, B. Renga, G. Rizzo, E. Distrutti, V. Shah and A. Morelli, Hepatology, 2005, 42, 539–548 CrossRef CAS PubMed.
- S. Y. Liu, X. C. Duan, S. Jin, X. Teng, L. Xiao, H. M. Xue and Y. M. Wu, Am. J. Hypertens., 2017, 30, 67–74 CrossRef CAS.
- C. Szabo, Nat. Rev. Drug Discovery, 2007, 6, 917–935 CrossRef CAS.
- P. Kamoun, M. C. Belardinelli, A. Chabli, K. Lallouchi and C. V. Bernadette, Am. J. Med. Genet., Part A, 2003, 116, 310–311 CrossRef.
- Y. Zhang, Z. H. Tang, Z. Ren, S. L. Qu, M. H. Liu, L. S. Liu and Z. S. Jiang, Mol. Cell Biol., 2013, 33, 1104–1113 CrossRef CAS PubMed.
- D. Li, L. Li, Z. Ge, J. Limwachiranon, Z. Ban, D. Yang and Z. Luo, Postharvest Biol. Technol., 2017, 129, 136–142 CrossRef CAS.
- S. P. Li, K. D. Hu, L. Y. Li, A. M. Jiang, F. Xiao, Y. Han, Y. S. Liu and H. Zhang, J. Agric. Food Chem., 2014, 62, 1119–1129 CrossRef CAS.
- H. Francis, K. Lotta, M. Meriem, C. Arnaud, B. Cédric, V. Mathias, D. Frank, M. Gaël and B. Robin, Analyst, 2018, 143, 5536–5554 RSC.
- M. F. Iulietto, P. Sechi, E. Borgogni and B. T. Cenci-Goga, Ital. J. Anim. Sci., 2015, 14, 316–326 Search PubMed.
- R. Tocmo, Y. Wu, D. Liang, V. Fogliano and D. Huang, Food Chem., 2017, 221, 1867–1873 CrossRef CAS.
- A. V. Kroll, V. Smorchkov and A. Y. Nazarenko, Sens. Actuators, B, 1994, 21, 97–100 CrossRef CAS.
- M. García-Calzada, G. Marbán and A. B. Fuertes, Anal. Chim. Acta, 1999, 380, 39–45 CrossRef.
- J. Radford-Knoery and G. A. Cutter, Anal. Chem., 1993, 65, 76–982 CrossRef.
- P. R. Brub, P. D. Parkinson and E. R. Hall, J. Chromatogr. A, 1999, 830, 485–489 CrossRef.
- N. S. Lawrence, R. P. Deo and J. Wang, Anal. Chim. Acta, 2004, 517, 131–137 CrossRef CAS.
- W. J. Zhang, F. J. Huo and C. X. Yin, Org. Lett., 2019, 21, 5277–5280 CrossRef CAS PubMed.
- L. L. Long, M. Y. Huang, N. Wang, Y. J. Wu, K. Wang, A. Gong, Z. J. Zhang and J. L. Sessler, J. Am. Chem. Soc., 2018, 140, 1870–1875 CrossRef CAS PubMed.
- Y. N. Luo, C. Z. Zhu, D. Du and Y. H. Lin, Anal. Chim. Acta, 2019, 1061, 1–12 CrossRef CAS.
- M. G. Ren, Z. H. Li, B. B. Deng, L. Wang and W. Y. Lin, Anal. Chem., 2019, 91, 2932–2938 CrossRef CAS.
- J. P. Wang, Y. Wen, F. J. Huo and C. X. Yin, Sens. Actuators, B, 2019, 294, 141–147 CrossRef CAS.
- Z. S. Wu, D. W. Liang and X. J. Tang, Anal. Chem., 2016, 88, 9213–9218 CrossRef CAS.
- A. K. Steiger, S. Pardue, C. G. Kevil and M. D. Pluth, J. Am. Chem. Soc., 2016, 138, 7256–7259 CrossRef CAS.
- J. X. Hong, W. Y. Feng and G. Q. Feng, Sens. Actuators, B, 2018, 262, 837–844 CrossRef CAS.
- H. Wang, X. M. Wu, S. X. Yang, H. Y. Tian, Y. G. Liu and B. G. Sun, Dyes Pigm., 2018, 160, 757–764 CrossRef.
- H. D. Li, Q. C. Yao, J. L. Fan, N. Jiang, J. Y. Wang, J. Xia and X. J. Peng, Chem. Commun., 2015, 51, 16225–16228 RSC.
- L. L. Zhang, H. K. Zhu, M. M. Li and X. F. Gu, Chem. Commun., 2015, 51, 13135–13137 RSC.
- B. Zhao, T. Liu, Y. Fang, L. Y. Wang, B. Song and Q. G. Deng, Tetrahedron Lett., 2016, 57, 4417–4423 CrossRef CAS.
- B. Zhao, T. Liu, Y. Fang, L. Y. Wang, W. Kan, Q. G. Deng and B. Song, Sens. Actuators, B, 2017, 246, 370–379 CrossRef CAS.
- J. J. Zhang, J. X. Wang, J. T. Liu, L. L. Ning, X. Y. Zhu, B. F. Yu, X. Y. Liu, X. J. Yao and H. X. Zhang, Anal. Chem., 2015, 87, 4856–4863 CrossRef CAS.
- W. Y. Feng, M. X. Li, Y. Sun and G. Q. Feng, Anal. Chem., 2015, 89, 6106–6112 CrossRef.
- B. X. Zhang, C. P. Ge, J. Yao, Y. P. Liu, H. C. Xie and J. G. Fang, J. Am. Chem. Soc., 2015, 137, 757–769 CrossRef CAS.
- T. Fang, X. D. Jiang, C. L. Sun and Q. Li, Sens. Actuators, B, 2019, 290, 551–557 CrossRef CAS.
- X. W. Cao, W. Y. Lin, K. B. Zheng and L. W. He, Chem. Commun., 2012, 48, 10529–10531 RSC.
- A. Roy, D. Kand, T. Saha and P. Talukdar, Chem. Commun., 2014, 50, 5510–5513 RSC.
- P. He, L. J. Tang, K. L. Zhong, S. H. Hou and X. M. Yan, Chin. J. Org. Chem., 2017, 37, 423–428 CrossRef CAS.
- S. Landaud, S. Helinck and P. Bonnarme, Appl. Microbiol. Biotechnol., 2008, 77, 1191–1205 CrossRef CAS.
- H. Liu, Y. Saito, D. F. Ai Riza, N. Kondo, X. T. Yang and D. H. Han, Food Chem., 2019, 287, 369–374 CrossRef CAS.
- H. Kimura, Amino Acids, 2011, 41, 113–121 CrossRef CAS.
- N. Velusamy, A. Binoy, K. N. Bobba, D. Nedungadi, N. Mishra and S. Bhuniya, Chem. Commun., 2017, 53, 8802–8805 RSC.
- Y. Xu, H. P. Du, J. Li, R. Xu, Y. L. Wang, S. J. You, H. Liu, F. Wang, Y. J. Cao, C. F. Liu and L. F. Hu, Pharmacol. Res., 2014, 87, 18–25 CrossRef CAS.
- H. Zhang, X. Kong, Y. Tang and W. Lin, ACS Appl. Mater. Interfaces, 2016, 8, 16227–16239 CrossRef CAS.
- C. C. Zhao, X. L. Zhang, K. B. Li, S. J. Zhu, Z. Q. Guo, L. L. Zhang, F. Y. Wang, Q. Fei, S. H. Luo, P. Shi, H. Tian and W. H. Zhu, J. Am. Chem. Soc., 2015, 137, 8490–8498 CrossRef CAS.
- C. R. Powell, K. Kaur, K. M. Dillon, M. J. Zhou, M. Alaboalirat and J. B. Matson, ACS Chem. Biol., 2019, 146, 1129–1134 CrossRef.
- F. R. Wang, G. Xu, X. F. Gu, Z. G. Wang, Z. Q. Wang, B. Shi, C. F. Lu, X. Q. Gong and C. C. Zhao, Biomaterials, 2018, 159, 82–90 CrossRef CAS.
- G. Xu, Q. L. Yan, X. G. Lv, Y. Zhu, K. Xin, B. Shi, R. C. Wang, J. Chen, W. Gao, P. Shi, C. H. Fan, C. C. Zhao and H. Tian, Angew. Chem., Int. Ed., 2018, 57, 3626–3630 CrossRef CAS.
Footnote |
† Electronic supplementary information (ESI) available. See DOI: 10.1039/c9an01629e |
|
This journal is © The Royal Society of Chemistry 2020 |
Click here to see how this site uses Cookies. View our privacy policy here.