DOI:
10.1039/C9BM01377F
(Paper)
Biomater. Sci., 2020,
8, 342-352
Targeted multifunctional nanomaterials with MRI, chemotherapy and photothermal therapy for the diagnosis and treatment of bladder cancer†
Received
29th August 2019
, Accepted 22nd October 2019
First published on 25th October 2019
Abstract
Bladder cancer is a common urinary tract tumor in clinic, and its morbidity and mortality are always high. Surgical treatment is operator dependent, residual tumor cells often lead to tumor recurrence, and chemotherapy after surgery causes high side effects. So, it is urgent to develop new methods for the theranostics of bladder cancer. Among them, functional nanomaterials have shown good application in tumor theranostics, but they are rarely used in bladder cancer. In our work, we demonstrate the fabrication of folate-modified vincristine-loaded polydopamine-coated Fe3O4 superparticles (Fe3O4@PDA-VCR-FA SPs), and applied them in the theranostics of bladder cancer. The PDA shell not only improves the colloidal stability and biocompatibility, but also enhances the photothermal effect and prolongs the blood circulation half-life. The half-life of Fe3O4@PDA-VCR-FA SPs in blood is calculated as 2.83 h, and the tumor retention rate is 5.96 %ID g−1, these data are significantly higher than those before folic acid modification. The superparamagnetism of Fe3O4 and loading of vincristine endow Fe3O4@PDA-VCR-FA SPs with magnetic resonance imaging and chemotherapy capabilities. Further by employing NIR laser-triggered photothermal therapy, bladder tumors were ablated completely, and no recurrence was observed. Blood and histological tests of the major organs confirm that Fe3O4@PDA-VCR-FA SPs show good biosafety.
1. Introduction
Bladder cancer is the 10th most common type of cancer worldwide, with 549
000 new cases (3% of new cancer cases) and 200
000 deaths (2.1% of cancer deaths) estimated in 2018.1 Bladder cancer is the most common malignant cancer of urinary system, and it seriously endangers human health.2,3 In clinical treatment, transurethral resection of the bladder tumors (TURBT) serves as a definitive treatment for most bladder cancer, but TURBT is operator dependent, and recurrence may be caused by residual tumor over time.4,5 To prevent the development of bladder cancer and reduce the recurrence rate, chemotherapy is often used.6–8 However, severe side effects brought by chemotherapeutic agents on vital organs and tissues are far from the expected satisfaction, which urges the development of new methods for bladder cancer treatment.9,10
In recent years, nanoscience and nanotechnology have been developing rapidly, and tumor diagnosis and treatment methods based on nanomaterials have also been widely developed.11–17 Many functional nanomaterials, such as rare-earth compounds,18,19 bismuth compounds,20,21 and Fe3O4,22 have been developed for use in cell imaging and tumor diagnosis. These nanomaterials with good biocompatibility and low toxicity have also shown great potential in tumor therapy.23,24 Photothermal therapy,25–27 photodynamic therapy,28,29 immunotherapy,30,31 and other emerging tumor therapy methods have also been widely used. However, the application of nanomaterials in the diagnosis and treatment of bladder cancer is rarely reported. Therefore, it is of great significance to design a reasonable diagnosis and treatment system and apply nanomaterials to the diagnosis and treatment of bladder cancer.
In a variety of nanomaterials, inorganic nanomaterials and polymer nanomaterials are widely recognized by researchers due to their outstanding clinical properties.32–36 Inorganic nanomaterials generally have high extinction coefficient and photothermal conversion efficiency, which can convert the absorbed near-infrared (NIR) light into heat, so as to achieve the goal of photothermal elimination of cancer cells.37 Some inorganic nanomaterials can also be used as imaging reagents due to some of their own characteristics.38 Among them, Fe3O4 nanoparticles are a good choice because of their magnetic properties and because they can be used as T2-weighted magnetic resonance imaging (MRI) contrast agents.39,40 At the same time, Fe3O4 nanoparticles (NPs) are also good photothermal reagents, and studies have proved that the photothermal properties of Fe3O4 can be further improved by assembling small Fe3O4 nanoparticles into superparticles (SPs).41 However, the potential toxicity of inorganic nanomaterials such as Fe3O4 nanoparticles in vivo limits their biological applications.42,43 To solve this problem, researchers have made many attempts, among which polymer coating has shown the most significant effect.44 When inorganic nanoparticles are coated with polymer shells, the toxicity of nanomaterials is greatly reduced.45 At the same time, the stability of nanomaterials is improved, and the abundant groups on the polymer shells also provide the possibility for further surface grafting or drug loading.46 In addition, if the polymer shells themselves can be used as photothermal reagents, the photothermal properties of nanomaterials can be further improved to achieve better therapeutic effects.47,48 Polypyrrole,49–51 polyaniline,52,53 and polydopamine (PDA)54,55 are the representative polymers. Among them, dopamine is a substance existing in the human body.56 The polydopamine generated by its polymerization has low toxicity, good stability and high photothermal conversion efficiency, making it an ideal polymer shell nanomaterial.57
In our work, Fe3O4 SPs were coated with a PDA shell. After loading chemotherapeutic VCR into the PDA shell, the surface of the nanomaterial is further modified with folic acid, which produces Fe3O4@PDA-VCR-FA SPs, and these are used for the diagnosis and treatment of bladder cancer. In comparison with Fe3O4 SPs, Fe3O4@PDA-VCR-FA SPs show better theranostic performance. The PDA shell improves the extinction ability of nanomaterials at 808 nm, and further improves their photothermal conversion ability. It also effectively reduces the toxicity and enhances the biocompatibility of nanomaterials. The chemotherapeutic drugs loaded into the PDA shell can be released under the stimulation of an acidic tumor microenvironment and a NIR laser to accomplish chemotherapy. Meanwhile, the PDA shell is negatively charged, which is not easy to be cleared in blood circulation. Combined with the targeting effect of folic acid, the biological utilization rate of nanomaterials is greatly improved. The half-life of Fe3O4@PDA-VCR-FA SPs in blood is calculated as 2.83 h, and the tumor retention rate is 5.96 %ID g−1. The superparamagnetism of Fe3O4 endows Fe3O4@PDA-VCR-FA SPs with MRI function. Further combining an 808 nm laser, under the synergistic effect of MRI, chemotherapy and photothermal therapy, the multi-functional diagnosis and treatment of bladder cancer has been successfully implemented. Bladder tumors are ablated completely, and no recurrence was observed. Blood tests and histological slices of the major organs confirm that Fe3O4@PDA-VCR-FA SPs show good biosafety.
2. Experimental
2.1. Materials
Iron(III) acetylacetonate (Fe(acac)3, 98%), 1,2-hexadecanediol (90%), 1-octadecene (ODE, 90%), oleylamine (OLA, 70%), oleic acid (OA, 90%), dopamine hydrochloride (DA), tris-(hydroxymethyl)aminomethane (Tris, 99%), folic acid (FA, ≥97%), and paraformaldehyde (95%) were purchased from Sigma-Aldrich. The vincristine sulfate salt (VCR, 98%) was purchased from Aladdin. Dodecyl sodium sulfate (SDS) was purchased from Sinopharm Chemical Reagent Co., Ltd. HaCat and T24 cell lines were bought from Beyotime Biotechnology. DMEM and fetal bovine serum (FBS) were bought from Solarbio Life Science Company. Cell counting kit-8 (CCK-8) was purchased from Beyotime Biotechnology. Annexin-binding buffer, annexin-V-FITC and propidium iodide (PI) were purchased from Nanjing Keygen Biotechnology Company. Balb/c nude mice were obtained from Beijing Vital River Laboratory Animal Technology Company. Methylbenzene, absolute ethanol, and normal hexane were of analytical grade. Phosphate buffered solution (PBS), saline, and deionized water were used as received.
2.2. Preparation of Fe3O4@PDA SPs
Fe3O4@PDA SPs were prepared according to our previous work.37 Firstly, Fe3O4 NPs were synthesized by a thermal decomposition method. 2 mmol Fe(acac)3, 5 mmol 1,2-hexadecanediol, 6 mmol OLA, 30 mmol ODE and 6 mmol OA were added in a 50 mL three-necked bottle. Under the protection of a nitrogen atmosphere, the solution was stirred for 15 min. Then the solution was heated to 200 °C at the rate of 15 °C min−1. After 30 minutes, the solution was heated to 265 °C at the rate of 3 °C min−1. After 30 min of heating under reflux, the solution was cooled down to room temperature. After cooling, Fe3O4 NPs were extracted from the reaction solution and washed with absolute ethanol and normal hexane three times and dissolved in methylbenzene. Secondly, Fe3O4 SPs were prepared by an emulsification method. 4 mL of 7 mg ml−1 methylbenzene solution of Fe3O4 NPs was mixed with 12.5 mL of 4 mg mL−1 SDS solution under stirring at 400 rpm. After ultrasonication at 20 °C for 10 min, the emulsification temperature was then increased to 56 °C to evaporate methylbenzene for 3 h and then Fe3O4 SPs were obtained. Finally, 5 mL of 3 mg mL−1 Fe3O4 SP aqueous solution, 1 mL of 5.6 mg mL−1 DA aqueous solution and 20 ml Tris (10 mm, pH 8.5) were mixed at room temperature for 5 h, Fe3O4@PDA SPs were obtained by centrifugation at 8000 rpm for 15 min and then washed with deionized water three times.
2.3. Preparation of Fe3O4@PDA-VCR-FA SPs
Firstly, 5 mL of 2 mg mL−1 Fe3O4@PDA SPs were mixed with 5 ml of 2 mg ml−1 VCR solution. Under magnetic stirring in the dark for 24 h, the obtained suspension was purified by centrifugation at 7000 rpm for 10 min to obtain Fe3O4@PDA-VCR SPs. Secondly, 5 mL of 2 mg mL−1 Fe3O4@PDA-VCR SPs were mixed with FA solution of the same volume and concentration. After being stirred in the dark at room temperature for 12 h, the suspension was centrifuged at 6600 rpm for 10 min and washed with deionized water three times. The prepared Fe3O4@PDA-VCR-FA SPs were kept at 4 °C for further characterization.
2.4. Photothermal, MRI and drug release tests of Fe3O4@PDA-VCR-FA SPs in vitro
In photothermal performance tests in vitro, we first fixed the solution concentration as 100, 200 and 300 μg mL−1, adjusted the laser power density as 1, 2 and 3 W cm−2, respectively, and tested the temperature rise curve of the solution over time. After that, the laser power density was fixed as 1, 2 and 3 W cm−2, respectively, and the solution concentration was adjusted as 100, 200 and 300 μg mL−1 respectively to test the temperature rise curve of the solution with time. The temperature is recorded at an interval of 15 s by using an electric thermometer above the light path. Then the photothermal conversion efficiency was tested at 2 W cm−2. 200 μg mL−1 Fe3O4@PDA-VCR-FA SP aqueous solution was added into a tinfoil-capped quartz pool to prevent the vaporization of water. And the solution was irradiated for 960 s until the temperature gets stable. Then the solution was cooled to room temperature in an ambient environment. Finally, the solution was subjected to five heating and cooling cycles with a concentration of 300 μg mL−1 and a laser power density of 3 W cm−2 to observe the photothermal stability of Fe3O4@PDA-VCR-FA SPs. In MRI tests in vitro, we first prepared a concentration gradient Fe3O4@PDA-VCR-FA SP solution and tested the transverse relaxation time (T2). The transverse relaxation rate (r2) of Fe3O4@PDA-VCR-FA SPs was calculated. Then we used medical magnetic resonance imaging to compare the magnetic resonance imaging effects of Fe3O4@PDA-VCR-FA SPs. In drug release tests in vitro, Fe3O4@PDA-VCR-FA SPs were dissolved in PBS with a pH of 7.4 and 5.5, respectively. The content of VCR released in solution at 0, 0.5, 1, 2, 4, 6, 8, 10, 12, 16, 20, 24, 36 and 48 h was tested, respectively, and the release rate of chemotherapy drugs under different pH conditions was compared. Based on the above experiments, we used a 1 W cm−2 near-infrared laser to illuminate the solution for 5 min at the beginning of the two groups of experiments to compare the effect of laser on the release rate of chemotherapy drugs.
2.5. Cytotoxicity assay and the photothermal effect in vitro
In cytotoxicity studies, HaCat cells were cultured with Fe3O4 SPs, Fe3O4@PDA SPs, Fe3O4@PDA-VCR SPs, and Fe3O4@PDA-VCR-FA SPs at different concentrations of 0, 25, 50, 100, 150 and 200 μg mL−1. The relative cell viability was determined by the CCK-8 assay after 24 h, which was revealed by the optical density at 490 nm. In the photothermal treatment experiment in vitro, T24 cells were mixed with Fe3O4@PDA-VCR-FA SPs at a concentration of 50 μg mL−1 for 1 h in a 96-well plate, and then irradiated with an 808 nm laser for 10 min with different laser power densities of 0, 0.33, 0.5, 1, 2 and 3 W cm−2. The cells in the control group were not only treated with Fe3O4@PDA-VCR-FA SPs, but were also exposed to the same laser for the same time. Flow cytometry is also used to study the photothermal properties of nanomaterials in vitro. T24 cells were cultured with Fe3O4@PDA-VCR-FA SPs at a concentration of 50 μg mL−1 for 1 h in a 6-well plate, and then irradiated with the 808 nm laser with a laser power density of 0.33 W cm−2 for different times of 0, 5, 10, 15 and 20 min. The cells in the control group were not only treated with Fe3O4@PDA-VCR-FA SPs, but were also exposed to the same laser for the same time. All these experiments were repeated three times.
2.6. Safety study in vivo
50 μL of 0.12 mg mL−1 VCR, 50 μL of 2 mg mL−1 Fe3O4@PDA SPs, Fe3O4@PDA-VCR SPs and Fe3O4@PDA-VCR-FA SPs were i.v. injected into mice, respectively. 1 day after injection, the serum of mice was taken for blood glucose, blood lipids, and liver and kidney function tests. All these experiments were repeated five times, and five healthy mice of the same age were used as the control.
2.7. Pharmacokinetics tests in vivo
Pharmacokinetic studies of Fe3O4@PDA SPs, Fe3O4@PDA-VCR SPs and Fe3O4@PDA-VCR-FA SPs were performed by measuring the amount of Fe(III) in the blood. 50 μL of 2 mg mL−1 Fe3O4@PDA SPs, Fe3O4@PDA-VCR SPs and Fe3O4@PDA-VCR-FA SPs were i.v. injected into mice, respectively. Then, 10 μL of blood were taken from the tail vein at different time points in 0, 2, 4, 6, 8, 10, 12, 14 and 16 h. All of the blood samples were dissolved in aqua regia to measure the content of Fe(III) by inductively coupled plasma atomic emission spectroscopy (ICP-AES). By calculating the ratio between the blood Fe(III) and the total amount of Fe(III) in the injected SPs, the blood circulation half-life was calculated. 12 h after the last blood collection, heart, liver, spleen, lungs, kidneys, and tumors of the mice were dissected to determine the Fe(III) contents for biodistribution study. The primary organs and tumors of the mice were also collected 3 and 7 days after the injection of Fe3O4@PDA-VCR-FA SPs. Fe(III) contents were also determined by ICP-AES measurements after all these organs and tumors dissolved totally in aqua regia.
2.8. Animal experiments
40 of 4-week-old balb/c nude mice were purchased from Beijing Vital River Laboratory Animal Technology Company. All animal procedures were performed in accordance with the Guidelines for Care and Use of Laboratory Animals of Jilin University and approved by the Animal Ethics Committee of Jilin University. After one week's feed, 150 μL cell suspension containing 2.0 × 106 of T24 cells were subcutaneously injected into the right back leg of mice. Then, after the tumor volume reached around 400 mm3, the T24 tumor-bearing mice were randomly assigned to 8 groups: (1) control group; (2) laser control group; (3) Fe3O4@PDA SP group; (4) VCR group; (5) Fe3O4@PDA-VCR SP group; (6) Fe3O4@PDA-VCR-FA SP group; (7) Fe3O4@PDA-VCR SP + laser group; and (8) Fe3O4@PDA-VCR-FA SP + laser group. In groups 1 and 2, mice were i.v. injected with 50 μL saline and mice in group 2 were further irradiated with a 0.33 W cm−2 laser for 12 min. In group 3, mice were i.v. injected with 50 μL saline containing 100 μg Fe3O4@PDA SPs. In group 4, mice were i.v. injected with 50 μL saline containing 6 μg VCR. For groups 5 and 7, the mice were i.v. injected with 50 μL aqueous solution containing 100 μg Fe3O4@PDA-VCR SPs and mice in group 7 were further irradiated with a 0.33 W cm−2 laser for 12 min. As for groups 6 and 8, the mice were i.v. injected with 50 μL aqueous solution containing 100 μg Fe3O4@PDA-VCR SPs and mice in group 8 were further irradiated with a 0.33 W cm−2 laser for 12 min. A thermal camera was used to record the increases of temperature at the tumor sites of groups 2, 6 and 8. Every other day, the tumor size was monitored using a digital caliper and the body weights were recorded using an electronic balance in the following 14 days. The tumor volume (V mm3) is calculated as D2L/2 (D/L represents the short/long axis length of tumors, respectively. mm).
2.9. Characterization
TEM images were obtained by using a JEOL JEM-2100F electron microscope (acceleration voltage: 200 kV). UV-vis absorption spectra were performed by Shimadzu 2600 UV-vis spectrophotometer. FTIR spectra were recorded using a Bruker VERTEX 80V. The zeta potential and hydrate particle size were measured using a Zetasizer NanoZS (Malvern). The concentrations of Fe(III) were recorded by ICP-AES (Agilent 725). Flow cytometry analysis was performed by using a FACSCalibur (BD). T2-Weighted MRI images were obtained using a GE Signa unit (1.5 T, General Electric Company). Infrared thermal images were obtained using a FLUKE infrared thermal camera.
3. Results and discussion
3.1. Preparation and characterization of Fe3O4@PDA-VCR-FA SPs
In this work, oleylamine (OA)-stabilized Fe3O4 NPs are firstly synthesized by a classical thermal decomposition method, and the diameter of Fe3O4 NPs under a transmission electron microscope (TEM) was found to be 5 nm (Fig. 1a). Subsequently, in the presence of sodium dodecyl sulfate (SDS), through the method of evaporating toluene in the oil-in-water microemulsion, Fe3O4 SPs with a diameter of 60 nm are obtained (Fig. 1b). Then, utilizing the properties that a dopamine monomer can adsorb on the surface of negatively charged Fe3O4 SPs and be oxidized and polymerized under alkaline conditions, the surface of Fe3O4 SPs is successfully coated with a PDA shell with a thickness of 20 nm (Fig. 1c). Then, VCR is added into the Fe3O4@PDA SP aqueous solution to obtain Fe3O4@PDA-VCR SPs, in which VCR is loaded on the basis of π–π stacking with PDA. Most of the VCR absorbed on the surface of the PDA shell and just a little was embedded inside. The cross-linked PDA shell was considered to be loose so that some of the VCR could pass through the shell surface and enter into the shell. After 24 h, the FA is added into the reaction mixtures for another 24 h (Scheme 1), Fe3O4@PDA-VCR-FA SPs are collected and the diameter of Fe3O4@PDA-VCR-FA SPs observed from TEM is 100 nm (Fig. 1d). The addition of VCR and FA can scarcely affect the morphology of Fe3O4@PDA SPs (Fig. 1c and d); however, it will affect the hydrate particle size of nanomaterials. As shown in Fig. 1e, the average hydration particle diameter of Fe3O4 SPs is 70.15 ± 0.71 nm, which is measured by dynamic light scattering (DLS). After PDA coating, the hydration particle diameter increases to 125.83 ± 1.96 nm. After the binding of VCR and FA, the hydration particle diameter increases to 128.57 ± 1.29 nm and 133.87 ± 1.04 nm, respectively. The loading ratio of Fe3O4@PDA-VCR is calculated to be 19.3% (Fig. S1†). The zeta potential of Fe3O4@PDA-VCR-FA SPs is −35.2 ± 2.8 mV. The negative charge on the surface of SPs can not only make it steadily dispersible in aqueous solution but can also effectively avoid the scavenging effect of phagocytes and extend blood circulation in vivo, so as to efficiently accumulate into tumor tissues, which has potential application value. The component of Fe3O4@PDA-VCR-FA SPs is confirmed by Fourier-transform infrared (FTIR) spectra. The characteristic peaks of Fe3O4@PDA SPs, VCR and FA all appear in the characteristic peaks of Fe3O4@PDA-VCR-FA SPs (Fig. 1f), which suggests that these three components do participate in the composition of Fe3O4@PDA-VCR-FA SPs.
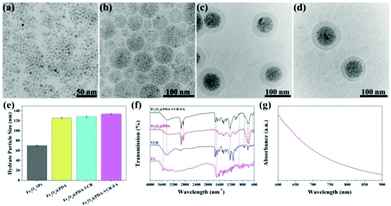 |
| Fig. 1 TEM images of Fe3O4 NPs (a), Fe3O4 SPs (b), Fe3O4@PDA SPs (c) and Fe3O4@PDA-VCR-FA SPs (d). DLS size (e) of Fe3O4 SPs, Fe3O4@PDA SPs, Fe3O4@PDA-VCR SPs and Fe3O4@PDA-VCR-FA SPs, FTIR spectra (f) of Fe3O4@PDA SPs, Fe3O4@PDA-VCR-FA SPs, VCR and FA and UV-vis-NIR absorption spectra (g) of Fe3O4@PDA-VCR-FA SPs. | |
 |
| Scheme 1 Schematic Illustration of the preparation process and function mechanism of Fe3O4@PDA-VCR-FA SPs. | |
As shown in Fig. S2,† PDA coating can enhance optical extinction at 808 nm. Besides, Fe3O4@PDA-VCR-FA SPs also show high extinction at 808 nm attributed to the coating of PDA, which can be used for photothermal therapy (Fig. 1g). To evaluate the photothermal properties of Fe3O4@PDA-VCR-FA SPs, the aqueous solution of Fe3O4@PDA-VCR-FA SPs is irradiated with an 808 nm laser. As shown in Fig. 2a–f, temperature increments increase with the increase of laser power density at fixed concentrations of 100, 200 and 300 μg mL−1 on account of more dose of irradiation applied. Obviously, when the laser power density is fixed at 1, 2 and 3 W cm−2, temperature increments increase with the increase of solution concentration as the collective heating effect results in a higher temperature increment. The photothermal conversion efficiency of Fe3O4@PDA-VCR-FA SPs is calculated as 55.5% (Fig. 2g and h). As shown in Fig. S3,† the photothermal conversion efficiency of Fe3O4 SPs is calculated as 38.4% (Fig. S2a and b†), and the photothermal conversion efficiency of Fe3O4@PDA SPs is measured as 52.5% (Fig. S2c and d†) because the PDA shell can protect the Fe3O4 SP core from more scattering and further improve the photothermal performance. In addition, a thermal imaging camera is further used to monitor the heating process (Fig. S4†). The laser stability of Fe3O4@PDA-VCR-FA SPs is assessed by NIR laser irradiation at the laser power density of 3 W cm−2; the photothermal properties did not decay even after 5 cycles of heating up and cooling down, and a slight increase is observed in the absorption spectra due to a small amount of evaporation of the solvent (Fig. 2i). The colloidal stability of the Fe3O4@PDA-VCR-FA SPs is further investigated and it was found that the SPs are well dispersible in physiological saline solution, PBS, and cell culture medium containing 10% FBS even after 2 weeks of incubation (Fig. S5a and b†). No obvious changes are observed in the absorption spectra even after 5 cycles of heating up and cooling down (Fig. S5c†). The excellent photothermal conversion efficiency, colloidal stability and photothermal stability mean that Fe3O4@PDA-VCR-FA SPs can be effectively applied in further application of photothermal therapy.
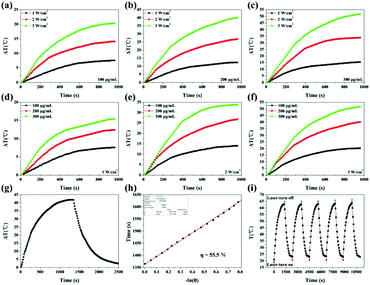 |
| Fig. 2 Temperature increment of Fe3O4@PDA-VCR-FA SP solution by altering the laser power density at a fixed concentration of 100 (a), 200 (b) and 300 μg mL−1 (c). Temperature increment of Fe3O4@PDA-VCR-FA SP solution by altering the concentration at the fixed power density of 1 (d), 2 (e) and 3 W cm−2 (f). The photothermal conversion efficiency is measured at the laser power density of 3 W cm−2 and the concentration of 200 μg mL−1. The temperature is recorded at the interval of 15 s by an electric thermometer. (g) The real-time temperature curve. (h) Time constants for heat transfer of Fe3O4@PDA-VCR-FA SPs are determined to be τ's = 325.4 s by applying the linear time data from the cooling period (after 1335 s) versus negative natural logarithm of driving force temperature. (i) The real-time temperature records of Fe3O4@PDA-VCR-FA SP aqueous solution as heating up and cooling down for 5 cycles at the time interval of 15 s. Red arrows under the curve and blue arrows on the curve represent laser-turn on and laser-turn off, respectively. | |
Besides, Fe3O4@PDA-VCR-FA SPs can serve as an excellent contrast agent in T2-weighted MRI due to the superparamagnetism of the Fe3O4 NP core. As illustrated in Fig. S6a,† the transverse relaxation rate (r2) is calculated using a nuclear magnetic resonance (NMR) spectrometer. Reasonably, 1/T2 of the Fe3O4@PDA-VCR-FA SPs increases with the concentration and the r2 of Fe3O4@PDA-VCR-FA SPs is measured as 210.91 mM−1 s−1. In addition, an increasing darkening effect is detected with the increasing concentration as revealed by using a 1.5 T MR clinical scanner (Fig. S6b†). Fe3O4@PDA-VCR-FA SPs can be potentially useful for tumor diagnosis as a T2-weighted MRI contrast agent.
To investigate the release behavior of VCR from Fe3O4@PDA-VCR-FA SPs under different conditions, Fe3O4@PDA-VCR-FA SPs are dispersed in PBS in different pH environments. As illustrated in Fig. S7,† only 10% of VCR is released in the physiological environment (pH 7.4), however, 30% of VCR is released from the Fe3O4@PDA-VCR-FA SPs at pH 5.5 within 48 h. The slightly acidic environment causes partial depolymerization of the PDA shell, which facilitates drug release (Fig. S8†). Then, NIR-triggered drug release was further studied. Fe3O4@PDA-VCR-FA SPs dissolved in PBS with a pH of 5.5 and 7.4 are irradiated by a NIR laser for 5 min with a laser power density of 1 W cm−2. Notably, an intense release of VCR from Fe3O4@PDA-VCR-FA SPs is observed because the PDA shell expands with heat, accelerating drug release. These results suggest that both the acidic environment and the NIR laser contribute to drug release. When the Fe3O4@PDA-VCR-FA SPs are stuck at the tumor site, the acidic environment of the tumor and the NIR laser given externally can actually stimulate the release of VCR, as a result, an excellent chemotherapy effect can be achieved while the adverse side-effects of VCR can be effectively minimized.
3.2. Cytotoxicity assay and photothermal therapy in vitro
HaCat cells are selected as a normal cell model to evaluate the cytotoxicity of Fe3O4 SPs, Fe3O4@PDA SPs, Fe3O4@PDA-VCR SPs, and Fe3O4@PDA-VCR-FA SPs by the CCK-8 assay. As shown in Fig. 3a, Fe3O4 SPs show high cytotoxicity on account of the toxic effect of SDS on the surface. At the concentration of 200 μg mL−1, the cell survival rate was only 60%. Comparatively, Fe3O4@PDA SPs show low cytotoxicity to HaCat cells mainly due to the prominent biocompatibility of the PDA shell. Even at the concentration of 200 μg mL−1, the cell survival rate was still higher than 86.2%. With the increasing concentration, slight increases in the cytotoxicity of both Fe3O4@PDA-VCR SPs and Fe3O4@PDA-VCR-FA SPs are observed. This is because as the concentration increases, the amount of VCR also increases, leading to increased cytotoxicity. However, it should be noted that the amount of VCR that can be released into the cell culture medium is very small, so the effect on cytotoxicity is not significant. 83.0% of HaCat cells still remain viable after incubation with 200 μg mL−1 Fe3O4@PDA-VCR-FA SPs for 24 h. For photothermal therapy in vitro, T24 cells were cultured with 50 μg mL−1 Fe3O4@PDA-VCR-FA SPs for 1 h and irradiated with an 808 nm laser for 10 min at different laser power densities of 0.33, 0.5, 1, 2 and 3 W cm−2. With the increase of laser power density, the cell survival rate decreases gradually. When the laser power density was 3 W cm−2, the relative cell survival rate was only 23.8%. However, cells untreated with Fe3O4@PDA-VCR-FA SPs showed little effect on the survival rate (Fig. 3b). Then, T24 cells were cultured with 50 μg mL−1 Fe3O4@PDA-VCR-FA SPs for 1 h and irradiated with the 808 nm NIR laser at a fixed laser power density of 0.33 W cm−2 for 0, 5, 10, 15 and 20 min, respectively. Then these cells are stained with Annexin V-FITC and propidium iodide (PI) to investigate the influence of thermo-chemotherapy by flow cytometry analysis. As shown in Fig. 3h–l, with increased laser irradiation time, the percentages of viable cells decrease radically as a result of the rise of temperature and the increased release of chemotherapy drugs. The differences of cell viability are statistically significant, which are 97.96 ± 0.32%, 92.35 ± 0.36%, 85.46 ± 0.26%, 62.75 ± 0.24% and 26.60 ± 0.42%, respectively. Conversely, T24 cells without Fe3O4@PDA-VCR-FA SP treatment exhibit little effect on cell viability. Even after 20 min irradiation, the cell survival rate is 95.59 ± 0.35%, which suggests that the NIR laser alone is unlikely to kill T24 bladder cancer cells efficiently (Fig. 3c–g).
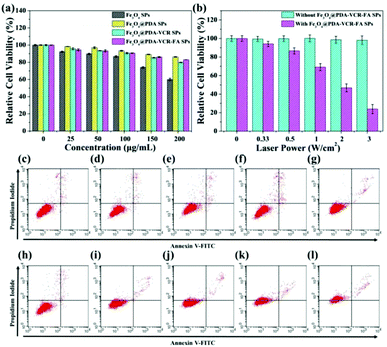 |
| Fig. 3 (a) T24 cells are incubated with Fe3O4 SPs, Fe3O4@PDA SPs, Fe3O4@PDA-VCR SPs, and Fe3O4@PDA-VCR-FA SPs at different concentrations of 0, 25, 50, 100, 150 and 200 μg mL−1 for 24 h, and the relative cell viabilities are estimated through the CCK-8 assay method. (b) T24 cells are incubated with 50 μg mL−1 Fe3O4@PDA-VCR-FA SPs for 1 h and then irradiated by an 808 nm laser at different power densities of 0, 0.33, 0.5, 1, 2 and 3 W cm−2 for 5 min, and the relative cell viabilities are estimated through the CCK-8 assay method. (h–l) The figures of the corresponding flow cytometry analysis. The images of the control group are (c–g). | |
3.3. Safety study in vivo
Assays for serum glucose, serum lipid and hepatorenal functions are used to evaluate the short-term toxicity of VCR, Fe3O4@PDA SPs, Fe3O4@PDA-VCR SPs, and Fe3O4@PDA-VCR-FA SPs (Fig. 4). The injection volume of VCR with a concentration of 0.12 mg mL−1 was 50 μL (the usual clinical dosage of VCR for intravenous injection is 0.02 mg kg−1). And the injection volume of Fe3O4@PDA SPs, Fe3O4@PDA-VCR SPs and Fe3O4@PDA-VCR-FA SPs with a concentration of 2 mg mL−1 was also 50 μL. 24 h after injection, serum is separated at different time points for the tests. Compared with the control group, indicators are largely unaffected in the injected groups. And there is barely noticeable difference between the groups in blood glucose (GLU), total cholesterol (TC), triglyceride (TG), high-density lipoprotein cholesterol (HDL-C), low-density lipoprotein cholesterol (LDL-C), total protein (TP), albumin (ALB), globulin (GLO), alanine aminotransferase (ALT), aspartate transaminase (AST), alkaline phosphatase (ALP), total bile acid (TBA), prealbumin (PA), blood urea nitrogen (BUN), uric acid (UA), creatinine (CREA), bicarbonate (HCO3), serum phosphorus (P), serum magnesium (Mg), and total calcium (tCa). These observations suggest that these materials make no difference to metabolism and homeostasis in vivo due to little short-term toxicity.
 |
| Fig. 4 (a–t) Indexes of blood glucose (GLU), total cholesterol (TC), triglyceride (TG), high-density lipoprotein cholesterol (HDL-C), low-density lipoprotein cholesterol (LDL-C), total protein (TP), albumin (ALB), globulin (GLO), alanine aminotransferase (ALT), aspartate transaminase (AST), alkaline phosphatase (ALP), total bile acid (TBA), prealbumin (PA), blood urea nitrogen (BUN), uric acid (UA), creatinine (CREA), bicarbonate (HCO3), serum phosphorus (P), serum magnesium (Mg), and total calcium (tCa). No obvious difference is found between healthy mice and mice treated with VCR, Fe3O4@PDA, Fe3O4@PDA-VCR SPs, and Fe3O4@PDA-VCR-FA SPs. The control group is age-matched healthy mice. | |
3.4. Pharmacokinetics tests and bio-distribution in vivo
Inductively coupled plasma atomic emission spectroscopy (ICP-AES) is used to evaluate the pharmacokinetics and biodistribution. As shown in Fig. 5a, the half-life time for Fe3O4@PDA SPS, Fe3O4@PDA-VCR SPs, and Fe3O4@PDA-VCR-FA SPs in blood is calculated as 1.12 ± 0.05, 2.23 ± 0.03, and 2.83 ± 0.02 h, respectively. The prolonged half-life of blood circulation is mainly the result of two factors. On the one hand, the negative charge of the PDA shell enhances the stealth effect of nanomaterials, which can effectively avoid being labeled and cleared by the immune system. On the other hand, the active targeting effect of FA is beneficial for improving the utilization rate of nanomaterials, which not only extends the half-life, but also greatly improves the retention rate of nanomaterials in tumors. 24 h after injection, it is found that Fe3O4@PDA-VCR-FA SPs show a higher accumulation rate of 5.96 ± 0.01 %ID g−1 at the tumor site while Fe3O4@PDA-VCR SPs exhibit a lower rate of 4.81 ± 0.01 %ID g−1 (Fig. 5b). The targeting ability of FA-modified Fe3O4@PDA-VCR-FA SPs was enhanced compared with unmodified Fe3O4@PDA-VCR SPs. T2-Weighted MRI is further used to investigate the targeting diagnostic capability of Fe3O4@PDA-VCR-FA SPs. As shown in Fig. 5c–e and h–j, due to the targeting effect of FA, nude mice injected with Fe3O4@PDA-VCR-FA SPs have a higher accumulation rate, the tumor site appears dark black (high MRI signal) and the boundary between tumor and surrounding normal tissues appears clear, indicating that Fe3O4@PDA-VCR-FA SPs have extraordinary ability of tumor diagnosis and tumor localization, while Fe3O4@PDA-VCR SPs have a slightly lower effect. In the control group, the tumor site appears bright yellow, indicating a very low MRI signal. The heart, liver, spleen, lungs and kidneys in these two groups are also dissected and dissolved with aqua regia respectively to evaluate the biodistribution of nanomaterials in the major organs. The retention rates of Fe3O4@PDA-VCR SPs are calculated to be 11.39 ± 0.02, 25.30 ± 0.10, 18.84 ± 0.12, 8.44 ± 0.01 and 7.36 ± 0.05 %ID g−1 in the heart, liver, spleen, lungs and kidneys, respectively, while the retention rates of Fe3O4@PDA-VCR-FA SPs show slightly decreased values, which is 10.50 ± 0.03, 24.50 ± 0.13, 16.36 ± 0.12, 7.99 ± 0.05 and 6.83 ± 0.01 %ID g−1, respectively, in these organs (Fig. 5b). The retention in the major organs was minimized by folic acid modification on the surface. The nanomaterials are highly enriched in the liver due to the phagocytosis of the reticuloendothelial system, but folic acid modification greatly reduces the amount of enriched nanomaterials, which leads to low side effects in vivo. Even nanomaterials that remain in organs can be gradually metabolized out of the body. We compared the residues of nanomaterials in organs 1 day, 3 days and 7 days after the injection of Fe3O4@PDA-VCR-FA SPs, and found that with the extension of time, the residues of nanomaterials gradually decreased, and could be reduced to a very low level by the 7th day. The accumulation rates of Fe3O4@PDA-VCR-FA SPs in the heart, liver, spleen, lungs and kidneys are only 3.38 ± 0.02, 10.20 ± 0.10, 6.14 ± 0.12, 4.06 ± 0.01 and 2.28 ± 0.05 %ID g−1, respectively, on the 7th day after injection, indicating that Fe3O4@PDA-VCR-FA SPs can be metabolized out of the body slowly (Fig. 5b). In addition, this study is also evaluated by MRI. The color of the major organs gradually becomes lighter on day 1, day 5 and day 10, and the signal intensity of the tumor becomes similar to that of the control group by the 10th day as revealed by coronal and axial MRI images. This change is consistent with the data of ICP-AES and can also prove the safety of Fe3O4@PDA-VCR-FA SPs in vivo (Fig. 5e–g and j–l).
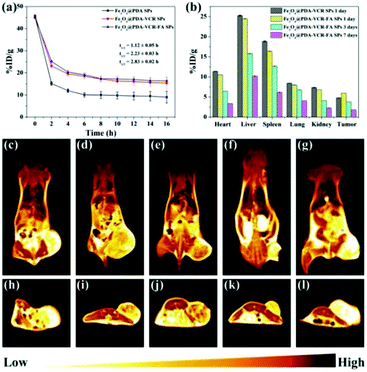 |
| Fig. 5 (a) Blood circulation of Fe3O4@PDA-VCR SPs and Fe3O4@PDA-VCR-FA SPs in vivo. (b) Biodistribution of Fe3O4@PDA-VCR-FA SPs in primary organs and tumor 1, 3 and 7 days after injection, and biodistribution of Fe3O4@PDA-VCR SPs 1 day after injection. Coronal T2-weighted MRI image of nude mice bearing T24 cell tumor without treatment (c), 1 day (d) after the injection of Fe3O4@PDA-VCR SPs, 1 day (e), 5 days (f) and 10 days (g) after the injection of Fe3O4@PDA-VCR-FA SPs. Axial T2-weighted MRI image of nude mice bearing T24 cell tumor without treatment (h), 1 day (i) after the injection of Fe3O4@PDA-VCR SPs, 1 day (j), 5 days (k) and 10 days (l) after the injection of Fe3O4@PDA-VCR-FA SPs. The color bar from yellow to black represents the MRI signal from low to high. | |
3.5. Synergistic therapy in vivo
In addition to imaging diagnostics, Fe3O4@PDA-VCR-FA SPs also have photothermal therapy and chemotherapy functions. The in vivo therapeutic studies were tested with T24 tumor models. In the control group, mice are only injected with saline. The tumor growing trend is shown in Fig. 6a. In the control group, tumors grew rapidly and the average volume of tumors reached 3344 mm3 and weighed 3.92 g 14 days later (Fig. 6a and c, k). Similar results are also found in the laser control group and the average volume and weight reached 3035 mm3 and 3.66 g, respectively (Fig. 6a and d, i). This suggests that the laser alone does not inhibit tumor growth. As for the mice injected with Fe3O4@PDA SPs, tumor growth was barely affected and it grew rapidly. The average volume and weight reached 2924 mm3 and 3.48 g, respectively. No necrosis of tumor cells was observed in H&E stained tumor slices in these groups (Fig. 6q and r, s). In contrast, the mice injected with VCR showed significant inhibition of the tumor in the first 6 days but rapid recurrence later, with the average volume and weight reaching 1123 mm3 and 0.92 g, respectively (Fig. 6a and f, n). H&E stained tumor slices showed necrotic cells (Fig. 6t). In the group of mice injected with Fe3O4@PDA-VCR SPs, tumors grew slowly and the average volume and weight were 1347 mm3 and 1.09 g, respectively (Fig. 6a and j, o). The inhibition of tumor growth is mainly due to the release of VCR loaded in the nanomaterials stimulated by the tumor microenvironment. In contrast, tumors with Fe3O4@PDA-VCR-FA SP injection showed an effectively depressed growth, and the average volume and weight were 942 mm3 and 0.88 g, respectively (Fig. 6a and h, p). Because of the specific binding between folic acid and the folic acid receptor on tumor cells, Fe3O4@PDA-VCR-FA SPs were more concentrated at the tumor sites than the Fe3O4@PDA-VCR SPs. In these two groups, local necrosis of tumor cells could also be found in H&E analysis because of the chemotherapeutic behavior of released VCR (Fig. 6u and v). However, chemotherapy alone cannot completely suppress the tumor growth. The tumor growth inhibition rate is calculated as 71.8% on the 14th day after Fe3O4@PDA-VCR-FA SP injection. Hence, photothermal therapy is performed with an 808 nm laser to completely eliminate the tumors. As shown in Fig. 7, the mice are injected with saline, Fe3O4@PDA-VCR SPs and Fe3O4@PDA-VCR-FA SPs, respectively. At 0, 3, 6, 9 and 12 min of 0.33 W cm−2 laser irradiation, infrared pictures reflecting the increase of temperature at the tumor sites are taken. In the control group, the tumor site temperature grows slowly, only reaching 33.2 degrees Celsius after 12 min irradiation (Fig. 7a). Tumor heating is significant in the mice injected with Fe3O4@PDA-VCR SPs, and the tumor site temperature is 45.2 degrees Celsius after 12 min irradiation (Fig. 7b). In contrast, mice injected with Fe3O4@PDA-VCR-FA SPs show a faster temperature increase at the tumor site, reaching 54.4 degrees Celsius 12 min later (Fig. 7c). Hence, the photothermal therapy effect of Fe3O4@PDA-VCR-FA SPs is better than that of Fe3O4@PDA-VCR SPs because Fe3O4@PDA-VCR-FA SPs were more concentrated at the tumor sites. Both the groups showed extensive necrocytosis at the tumor sites (Fig. 6w and x) and complete tumor ablation after laser treatment (Fig. 6a and i, j). Therefore, Fe3O4@PDA-VCR-FA SPs can achieve a better therapeutic effect with less nanomaterials, and has a better application prospect in the future. However, in the initial stage of thermo-chemotherapy treatment, the heat generated by nanomaterials can cause a large number of tumor cells to die, so photothermal therapy plays a greater role in synergistic therapy at this time. But in the later stage of treatment after the removal of the NIR laser, the photothermal therapy loses its effect, and chemotherapy plays a therapeutic role by relying on the chemotherapeutics released by photothermal stimulation to prevent the tumor recurrence.
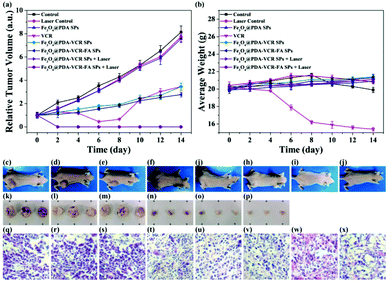 |
| Fig. 6
In vivo chemo-photothermal therapy. The mice are divided into 8 groups: (1) control group; (2) laser control group; (3) Fe3O4@PDA SP group; (4) VCR group; (5) Fe3O4@PDA-VCR SP group; (6) Fe3O4@PDA-VCR-FA SP group; (7) Fe3O4@PDA-VCR SP + laser group; (8) Fe3O4@PDA-VCR-FA SP + laser group. (a) Relative tumor volume growing trend curves for each group of T24 cell tumors. (b) Average body weight for each group. Photographs of the typical mouse bearing tumor model (c–j) and tumors (k–p) are taken from them in each group on the 14th day after injection. The average tumor weight is 3.92, 3.66, 3.48, 0.92, 1.09, and 0.88 g for each group, respectively. The distance between adjacent asterisks in (k–p) is 1 cm. In H&E stained tumor slices, tumors are taken from each group on the 14th day after the injection (q–v). H&E staining of tumor slices for groups 7 and 8 is performed immediately after continuous NIR laser irradiation (w, x). The dose of injected Fe3O4@PDA SPs, Fe3O4@PDA-VCR SPs and Fe3O4@PDA-VCR-FA SPs is 100 μg in 50 μL saline per mouse. The dose of injected VCR is 6 μg VCR dispersed in 50 μL saline per mouse. The laser power density is 0.33 W cm−2. | |
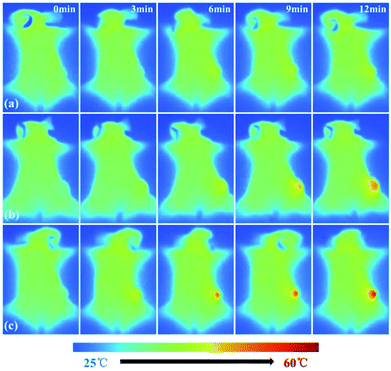 |
| Fig. 7 Infrared thermal images of the mice treated with saline (a), Fe3O4@PDA-VCR SPs (b), and Fe3O4@PDA-VCR-FA SPs (c) under the irradiation of an 808 nm laser for 0, 3, 6, 9, and 12 min at the power density of 0.33 W cm−2. | |
By recording the weight of mice, we found that in only two groups of thermo-chemotherapy, the mice gained weight steadily, which indicated that our treatment was safe and reliable without any effect on metabolism (Fig. 7b). As shown in Fig. 8, compared with the control group, there was no significant tissue hyperemia, cell edema, atrophy, necrosis, or infiltration of inflammatory cells in the H&E stained histopathological slices of vital organs observed in the experimental groups. This confirms that Fe3O4@PDA-VCR-FA SPs show good biosafety for the theranostics of bladder cancer.
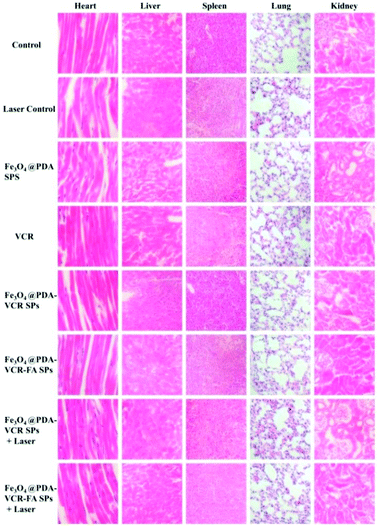 |
| Fig. 8 H&E stained splanchnic slices of each group after 14 days. | |
4. Conclusions
In conclusion, we show the fabrication of Fe3O4@PDA-VCR-FA SPs with MRI, chemotherapy and photothermal therapy. In comparison with Fe3O4 SPs, Fe3O4@PDA-VCR-FA SPs have better theranostic performance. The PDA shell not only improves the extinction ability of Fe3O4 SPs at 808 nm, but also improves the photothermal conversion efficiency. In addition, the PDA shell further effectively reduces the toxicity and enhances the biocompatibility of nanomaterials. The chemotherapeutic drugs loaded in the PDA shell can be released under the stimulation of an acidic tumor microenvironment and NIR laser to accomplish chemotherapy. Meanwhile, the negative charge of the PDA shell makes Fe3O4@PDA-VCR-FA SPs not easy to be cleared in blood circulation. Combined with the targeting effect of folic acid, the biological utilization rate of nanomaterials is greatly improved. The half-life of Fe3O4@PDA-VCR-FA SPs in blood is calculated as 2.83 h, and the tumor retention rate is 5.96 %ID g−1. The superparamagnetism of Fe3O4 makes Fe3O4@PDA-VCR-FA SPs be used in T2-weight MRI. Further combining an 808 nm NIR laser, under the synergistic effect of MRI, photothermal therapy and chemotherapy, the multi-functional diagnosis and treatment of bladder cancer has been successfully implemented. Bladder tumors are completely ablated, and no recurrence is observed. Blood tests and histological slices of the major organs confirm that Fe3O4@PDA-VCR-FA SPs show good biosafety.
Author contributions
Kepeng Tao and Shuwei Liu contributed equally to this paper. H. Z. and Y. C. H. proposed and supervised this research. H. Z., Y. C. H., K. P. T., S. W. L. and Y. L. co-designed and performed the tests and co-wrote the paper. L. W, H. P. Q., B. X. L., M. S. Z. M. Y. G., H. L., and X. Z. participated in most experiments. All authors have given approval to the final version of the manuscript.
Conflicts of interest
There are no conflicts to declare.
Acknowledgements
This work was supported by the National Natural Science Foundation of China (51425303, 51603084), the Science and Technology Development Program of Jilin Province (20180101167JC), the Jilin University Science and Technology Innovative Research Team 2017TD-06, and the Jilin University doctoral students interdisciplinary research funding project (10183201819).
References
- F. Bray, J. Ferlay, I. Soerjomataram, R. L. Siegel, L. A. Torre and A. Jemal, CA Cancer J. Clin., 2018, 68, 394–424 CrossRef.
- S. Antoni, J. Ferlay, I. Soerjomataram, A. Znaor, A. Jemal and F. Bray, Eur. Urol., 2017, 71, 96–108 CrossRef.
- L. C. Harshman, Cancer, 2016, 122, 1966–1970 CrossRef.
- M. Babjuk, A. Böhle, M. Burger, O. Capoun, D. Cohen, E. Compérat, V. Hernández, E. Kaasinen, J. Palou, M. Rouprêt, B. W. G. Van Rhijnm, S. F. Shariat, V. Soukup, R. J. Sylvester and R. Zigeuner, Eur. Urol., 2017, 71, 447–461 CrossRef.
- M. Rouprêt, M. Babjuk, E. Compérat, R. Zigeuner, R. J. Sylvester, M. Burger, N. C. Cowan and P. Contero, Eur. Urol., 2018, 73, 111–122 CrossRef.
- W. Chol, S. Porten, S. Kim, D. Willis, E. R. Plimack, J. Hoffman-Censits, B. Roth, T. W. Cheng, M. Tran, I. L. Lee, J. Melquist, J. Bondaruk, T. Majewski, S. Z. Zhang, S. Pretzsch, K. Baggerly, A. Siegker-Radtke, B. Czerniak, C. P. N. Dinney and D. J. McConkey, Cancer Cell, 2014, 25, 152–165 CrossRef.
- J. E. Rosenberg, J. Hoffman-Censits, T. Powles, M. S. van der Heijden, A. V. Balar, A. Necchi, N. Dawson, P. H. O'Donnell, A. Balmanoukian, Y. Loriot, S. Srinivas, M. M. Retz, P. Grivas, R. W. Joseph, M. D. Galsky, M. T. Fleming, D. P. Petrylak, J. L. Perez-Gracia, H. A. Burris, D. Castellano, C. Canil, J. Bellmunt, D. Bajorin, D. Nickles, R. Bourgon, G. M. Frampton, N. Cui, S. Mariathasan, O. Abidoye, G. D. Fine and R. Dreicer, Lancet, 2016, 387, 1909–1920 CrossRef CAS.
- R. J. Sylvester, W. Oosterlinck, S. Holmang, M. R. Sydes, A. Birtle, S. Gudjonsson, C. De Nunzio, K. Okamura, E. Kaasinen, E. Solsona, B. Ali-El-Dein, C. A. Tatar, B. A. Inman, J. N'Dow, J. R. Oddens and M. Babjuk, Eur. Urol., 2016, 69, 231–244 CrossRef.
- M. Vila-Caballer, G. Codolo, F. Munari, A. Malfanti, M. Fassan, M. Rugge, A. Balasso, M. de Bernard and S. Salmaso, J. Controlled Release, 2016, 238, 31–42 CrossRef CAS.
- H. Guo, F. P. Li, W. G. Xu, J. J. Chen, Y. C. Hou, C. W. Wang and J. X. Ding, Adv. Sci., 2018, 5, 1800004 CrossRef.
- W. P. Fan, B. Yung, P. Huang and X. Y. Chen, Chem. Rev., 2017, 117, 13566–13638 CrossRef CAS.
- L. Cheng, C. Wang, L. Z. Feng, K. Yang and Z. Liu, Chem. Rev., 2014, 114, 10869–10939 CrossRef CAS.
- X. Yang, M. X. Yang, B. Pang, M. Vara and Y. Xia, Chem. Rev., 2015, 115, 10410–10488 CrossRef CAS.
- M. P. Melancon, M. Zhou and C. Li, Acc. Chem. Res., 2011, 44, 947–956 CrossRef CAS.
- D. Kim, K. Shin, S. G. Kwon and T. Hyeon, Adv. Mater., 2018, 30, 1802309 CrossRef.
- Z. J. Gu, S. Zhu, L. Yan, F. Zhao and Y. L. Zhao, Adv. Mater., 2019, 31, 1800662 CrossRef.
- W. H. Chen, G. F. Luo and X. Z. Zhang, Adv. Mater., 2019, 31, 1802725 CrossRef.
- X. J. Xie, Z. J. Li, Y. W. Zhang and S. H. Guo, Small, 2017, 13, 1602843 CrossRef PubMed.
- L. N. Sun, R. Y. Wei, J. Feng and H. J. Zhang, Coord. Chem. Rev., 2018, 364, 10–32 CrossRef CAS.
- S. A. Zhu, L. J. Gong, J. N. Xie, Z. J. Gu and Y. L. Zhao, Small Methods, 2017, 1, 1700220 CrossRef.
- X. Li, J. Y. Shan, W. Z. Zhang, S. Su, L. H. Yuwen and L. H. Wang, Small, 2017, 13, 1602660 CrossRef.
- J. Estelrich and M. A. Busquets, Molecules, 2018, 23, 1567 CrossRef.
- F. Jabeen, M. Najam-ul-Haq, R. Javeed, C. W. Huck and G. K. Bonn, Molecules, 2014, 19, 20580–20593 CrossRef.
- R. Ge, M. Lin, X. Li, S. W. Liu, W. J. Wang, S. Y. Li, X. Zhang, Y. Liu, L. D. Liu, F. Shi, H. C. Sun, H. Zhang and B. Yang, ACS Appl. Mater. Interfaces, 2017, 9, 19706–19716 CrossRef CAS.
- M. Lin, D. D. Wang, S. W. Liu, T. T. Huang, B. Sun, Y. Cui, D. Q. Zhang, H. C. Sun, H. Zhang and H. Sun, ACS Appl. Mater. Interfaces, 2015, 7, 20801–20812 CrossRef CAS PubMed.
- Y. X. Chen, S. Y. Xiang, L. Wang, M. Y. Wang, C. C. Wang, S. W. Liu, K. Zhang and B. Yang, ChemPlusChem, 2018, 83, 1127–1134 CrossRef CAS.
- J.-G. Piao, L. M. Wang, F. Gao, Y.-Z. You, Y. J. Xiong and L. H. Yang, ACS Nano, 2014, 8, 10414–10425 CrossRef CAS.
- Y. D. Ruan, X. D. Jia, C. Wang, W. Y. Zhen and X. E. Jiang, ACS Biomater. Sci. Eng., 2019, 5, 1016–1022 CrossRef CAS.
- X. D. Sun, B. Zhuang, M. M. Zhang, H. L. Jiang and Y. G. Jin, ACS Biomater. Sci. Eng., 2019, 5, 724–739 CrossRef CAS.
- M. Luo, H. Wang, Z. H. Wang, H. C. Cai, Z. G. Lu, Y. Li, M. J. Du, G. Huang, C. S. Wang, X. Chen, M. R. Porembka, J. Lea, A. E. Frankel, Y. X. Fu, Z. J. Chen and J. M. Gao, Nat. Nanotechnol., 2017, 12, 648–654 CrossRef CAS.
- R. Kuai, L. J. Ochyl, K. S. Bahjat, A. Schwendeman and J. J. Moon, Nat. Mater., 2017, 16, 489–496 CrossRef CAS.
- W. H. Chen, J. H. Liu, Y. Wang, C. H. Jiang, B. Yu, Z. Sun and L. H. Lu, Angew. Chem., 2019, 58, 6290–6294 CrossRef CAS.
- Z. J. Zhang, J. Wang, X. Nie, T. Wen, Y. L. Ji, X. C. Wu, Y. L. Zhao and C. Y. Chen, J. Am. Chem. Soc., 2014, 136, 7317–7326 CrossRef CAS PubMed.
- M. Lin, S. W. Liu, D. D. Wang, S. Y. Li, X. Zhang, R. Ge, X. Li, Y. Liu, W. Z. Song and H. C. Sun, RSC Adv., 2017, 7, 56621–56628 RSC.
- Z. Zhang, T.-Y. Zeng, L. Xia, C.-Y. Hong, D.-C. Wu and Y.-Z. You, Nat. Commun., 2018, 9, 2577 CrossRef.
- L.-H. Wang, Z.-D. Zhang, C.-Y. Hong, X.-H. He, W. You and Y.-Z. You, Adv. Mater., 2015, 27, 3202–3207 CrossRef CAS.
- S. W. Liu, L. Wang, M. Lin, Y. Liu, L. N. Zhang and H. Zhang, Chin. J. Polym. Sci., 2019, 37, 115–128 CrossRef CAS.
- R. Ge, C. W. Liu, X. Zhang, W. J. Wang, B. X. Li, J. Liu, Y. Liu, H. C. Sun, D. Q. Zhang, Y. C. Hou, H. Zhang and B. Yang, ACS Appl. Mater. Interfaces, 2018, 10, 20342–20355 CrossRef CAS.
- L.-S. Lin, Z.-X. Cong, J.-B. Cao, K.-M. Ke, Q.-L. Peng and J. H. Gao, ACS Nano, 2014, 8, 3876–3883 CrossRef CAS.
- R. Ge, X. Li, M. Lin, D. D. Wang, S. Y. Li, S. W. Liu, Q. Tang, Y. Liu, J. L. Jiang, L. D. Liu, H. C. Sun, H. Zhang and B. Yang, ACS Appl. Mater. Interfaces, 2016, 8, 22942–22952 CrossRef CAS.
- X. Zhang, X. W. Xu, T. T. Li, M. Lin, X. Y. Lin, H. Zhang, H. C. Sun and B. Yang, ACS Appl. Mater. Interfaces, 2014, 6, 14552–14561 CrossRef CAS.
- A. Nel, T. Xia, L. Madler and N. Li, Science, 2006, 311, 622–627 CrossRef CAS PubMed.
- S. Sharifi, S. Behzadi, S. Laurent, M. L. Forrest, P. Stroeve and M. Mahmoudi, Chem. Soc. Rev., 2012, 41, 2323–2343 RSC.
- Z. L. Li, Y. Hu, K. A. Howard, T. T. Jiang, X. L. Fan, Z. H. Miao, Y. Sun, F. Besenbacher and M. Yu, ACS Nano, 2016, 10, 984–997 CrossRef CAS.
- Y. S. Jin, Y. Y. Li, X. B. Ma, Z. B. Zha, L. L. Shi, J. Tian and Z. F. Dai, Biomaterials, 2014, 35, 5795–5804 CrossRef CAS.
- S. W. Liu, L. Wan, M. Lin, D. D. Wang, Z. Q. Song, S. Y. Li, R. Ge, X. Zhang, Y. Liu, Z. M. Li, H. C. Sun, B. Yang and H. Zhang, ACS Appl. Mater. Interfaces, 2017, 9, 44293–44306 CrossRef CAS.
- Y. G. Shi, M. Y. Liu, F. J. Deng, G. J. Zeng, Q. Wan, X. Y. Zhang and Y. Wei, J. Mater. Chem. B, 2017, 5, 194–206 RSC.
- Y. S. Jin, X. Yang and J. Tian, Nanoscale, 2018, 10, 9594–9601 RSC.
- M. Z. Wang, Polymers, 2016, 8, 373 CrossRef.
- Z. B. Zha, X. L. Yue, Q. S. Ren and Z. F. Dai, Adv. Mater., 2013, 25, 777–782 CrossRef CAS PubMed.
- M. Chen, X. L. Fang, S. H. Tang and N. F. Zheng, Chem. Commun., 2012, 48, 8934–8936 RSC.
- J. Zhou, Z. G. Lu, X. J. Zhu, X. J. Wang, Y. Liao, Z. F. Ma and F. Y. Li, Biomaterials, 2013, 34, 9584–9592 CrossRef CAS.
- S. W. Liu, L. Wang, M. S. Zhang, K. P. Tao, B. Wang, M. Lin, X. Zhang, Y. Liu, Y. C. Hou, H. Zhang and B. Yang, ACS Appl. Mater. Interfaces, 2019, 11, 25730–25739 CrossRef CAS.
- R. Mrówczyński, ACS Appl. Mater. Interfaces, 2018, 10, 7541–7561 CrossRef.
- M. Y. Liu, G. J. Zeng, K. Wang, Q. Wan, L. Tao, X. Y. Zhang and Y. Wei, Nanoscale, 2016, 8, 16819–16840 RSC.
- J. J. Zhou, Q. R. Xiong, J. L. Ma, J. H. Ren, P. B. Messersmith, P. Chen and H. W. Duan, ACS Nano, 2016, 10, 11066–11075 CrossRef CAS.
- X. Y. Zhong, K. Yang, Z. L. Dong, X. Yi, Y. Wang, C. C. Ge, Y. L. Zhao and Z. Liu, ACS Appl. Mater. Interfaces, 2015, 25, 7327–7336 CAS.
Footnotes |
† Electronic supplementary information (ESI) available: Additional UV absorption spectra of VCR, IR thermal images, colloidal stability, MRI performance of Fe3O4@PDA-VCR-FA SPs and the content of VCR released from Fe3O4@PDA-VCR SPs. See DOI: 10.1039/c9bm01377f |
‡ These authors contributed equally. |
|
This journal is © The Royal Society of Chemistry 2020 |
Click here to see how this site uses Cookies. View our privacy policy here.