Photochemistry of iron in aquatic environments
Received
11th September 2019
, Accepted 20th December 2019
First published on 20th December 2019
Abstract
Light energy is a driver for many biogeochemical element cycles in aquatic systems. The sunlight-induced photochemical reduction of ferric iron (Fe(III) photoreduction) to ferrous iron (Fe(II)) by either direct ligand-to-metal charge transfer or by photochemically produced radicals can be an important source of dissolved Feaq2+ in aqueous and sedimentary environments. Reactive oxygen species (ROS) are formed by a variety of light-dependent reactions. Those ROS can oxidize Fe(II) or reduce Fe(III), and due to their high reactivity they are key oxidants in aquatic systems where they influence many other biogeochemical cycles. In oxic waters with circumneutral pH, the produced Fe(II) reaches nanomolar concentrations and serves as a nutrient, whereas in acidic waters, freshwater and marine sediments, which are rich in Fe(II), the photochemically formed Fe(II) can reach concentrations of up to 100 micromolar and be used as additional electron donor for acidophilic aerobic, microaerophilic, phototrophic and, if nitrate is present, for nitrate-reducing Fe(II)-oxidizing bacteria. Therefore, Fe(III) photoreduction may not only control the primary productivity in the oceans but has a tremendous impact on Fe cycling in the littoral zone of freshwater and marine environments. In this review, we summarize photochemical reactions involving Fe, discuss the role of ROS in Fe cycling, and highlight the importance of photoreductive processes in the environment.
Environmental significance
Light drives a series of physico-chemical reactions in natural environments and it has a huge impact on the development of microbial life. Iron is required as trace element for almost any kind of living organisms and its provision in aquatic environments is controlled by iron(III) photoreduction. Here we summarize the current knowledge of the reaction network that connects light and aquatic environments via the redox cycling of iron and the consecutive formation of reactive oxygen species.
|
Light availability on Earth
The sun provides our planet with light and thereby with enormous amounts of energy.1,2 Light is electromagnetic radiation, propagating as wave through space.3 It consists of a wide range of wavelengths (electromagnetic spectrum) ranging from the very short and energy-rich gamma rays (<0.01 nm) to the very long and energy-poor radio waves (>10 cm). The visible light (VIS) of the electromagnetic spectrum covers only a narrow band of wavelengths ranging from 400 nm (violet) to 700 nm (red).3 Large parts of the electromagnetic spectrum coming from the sun are reflected or absorbed in the atmosphere, e.g. by ozone, aerosols or water vapor, before reaching the Earth's surface.4,5 Especially shorter wavelength radiation (<400 nm), which has the potential to photochemically damage cells,6,7 is absorbed in the atmosphere to a great extent (Fig. 1).4 On a cloudless day, typically 3–5% of the total surface irradiance is ultraviolet A (UVA; 320–400 nm) and ultraviolet B (UVB; 280–320 nm) light,8–10 but the percentage depends on latitude, season, time of the day and absorbing substances in the atmosphere such as ozone.9 Light can also be seen as particles of energy (photons)11 and light intensity can be denoted as photon flux in μmol photons m−2 s−1 (microeinstein) or as spectral irradiance in W m−2 nm−1 giving the power of a particular wavelength of light.1 The photon flux reaching the Earth's surface during the day is on average a few hundred1 to 2000 μmol photons m−2 s−1 under full sunlight.12,13 In lakes or in the ocean, the light intensity decreases exponentially with water depth by absorption and scattering4 and the light penetration depth into the water depends on concentration and composition of attenuating substances such as phytoplankton, particles or dissolved organic molecules in the water column as well as on wavelength (Fig. 1).7 In the clearest natural waters, visible light reaches a depth of 170 m with 1% of the surface irradiance remaining, whereas in highly turbid waters, this threshold is already reached in a few meters depth.7,14 The infrared wavelength region (IR, 700 nm to 100 μm) of the electromagnetic spectrum is absorbed most strongly in the water column, followed by ultraviolet (UV) light (1 nm to 400 nm) if the concentration of attenuating substances is low.15,16 The greatest transparency of clear ocean water lies with 480 nm in the blue region of the VIS range15 and light attenuation in water generally increases with longer wavelengths.14 Reaching the sediment surface, e.g. in littoral waters, light is penetrating into the sediment, depending on particle size and composition.17 While UV light usually only penetrates some hundreds of micrometers into the sediment,18,19 light in the VIS range can penetrate several millimeters whereby light penetration depth decreases with decreasing grain size.17 Directly on the sediment surface, the scalar irradiance (i.e. the light coming from all directions at one point)17 can be up to 280% of the incident irradiance due to scattering effects of the sediment particles.17 In contrast to the water column, light in the range of 450 to 500 nm (blue) is attenuated most strongly in sediments and attenuation decreases towards longer wavelengths, lowest in the IR region (Fig. 1).17
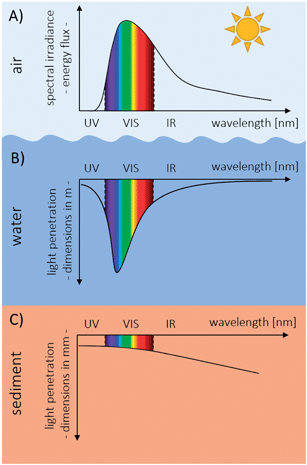 |
| Fig. 1 (A) Simplified daylight spectrum as energy flux reaching the Earth's surface; (B) and (C) Qualitative light penetration into water or sediment (here: pure sand) showing the relative depth, where 1% remains of the spectral energy flux at the water or sediment surface, respectively. | |
Impact of light in aquatic environments
The most important biochemical process in aquatic environments that is relying on light is oxygenic photosynthesis. During this process, plants or cyanobacteria absorb solar energy of specific wavelength regions in light-harvesting pigments by structures called chromophores,20,21 convert light energy into chemical energy (ATP) and further by CO2 fixation into biomass and release oxygen (O2) as a by-product.13 Additionally, anoxygenic phototrophic microorganisms such as green sulphur, purple sulphur or purple non-sulphur bacteria, use light as energy source for their metabolisms. Instead of water, they use reduced inorganic or organic compounds as electron donor, e.g. sulfide, hydrogen or ferrous iron (Fe(II)) (anoxygenic photosynthesis).22–25 However, light is not only the energy source of such microbially catalyzed reactions but also provides energy for purely photochemical processes in the environment. Light can photochemically degrade larger molecules of dissolved organic matter (DOM), especially in the UV region (280–400 nm),16,26–28 resulting in the production of a variety of organic molecules with reduced molecular weight27,29,30 or in the production of dissolved inorganic carbon (photochemical mineralization of DOM).27,31,32 The photochemically formed products can have different chemical properties with increased or decreased bioavailability.26,27,29,33–36 Light-induced dissolution of particulate organic matter from resuspended sediments releases significant amounts of dissolved organic carbon (DOC) into the water column, especially in coastal or estuarine regions that receive large sediment plumes.28,37–39 Reactive oxygen species (ROS) such as singlet oxygen (1O2), superoxide (O2−˙), hydroxyl radical (OH˙) and hydrogen peroxide (H2O2) can form during photolysis of DOM,20,40–45 which have tremendous implications on different biogeochemical processes due to their high reactivity.26,43,46–48 Photochemically produced superoxide or H2O2 are able to reduce for instance manganese oxides49 and the photoreduction of manganese oxides by dissolved organic substances induced by sunlight is an important reaction for maintaining dissolved Mn(II) concentrations in seawater for supply of manganese to phytoplankton.50,51
Besides the importance of light in the cycling of carbon or manganese, light is also a driving force in the Fe redox cycle of aquatic environments and sediments as it is able to photochemically induce the reduction of Fe(III) to Fe(II) (Fe(III) photoreduction). Light induced reactions are shown in Fig. 2. In this review, we summarize photochemical processes of Fe in aquatic ecosystems including the mechanisms responsible for light-induced reduction of Fe(III) and discuss the consequences of these photochemical reactions for biogeochemical Fe cycling.
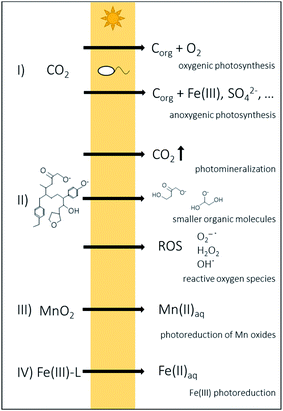 |
| Fig. 2 Illustration of light induced reactions in aquatic environments with reactants on the left and products on the right of the yellow bar indicating a photochemical reaction: (I) oxygenic and anoxygenic photosynthesis (microbially catalyzed), (II) degradation of DOC by light forming CO2, smaller organic molecules and/or reactive oxygen species (ROS), (III) photoreduction of manganese oxides (MnO2), (IV) photoreduction of organically complexed Fe(III) (Fe(III)–L). | |
General mechanisms of Fe photochemistry
In aquatic systems and sediments with circumneutral pH, dissolved Fe(III) (Feaq3+) occurs only complexed with organic molecules due to the poor solubility of Fe(III) and its tendency to precipitate as poorly soluble Fe(III) (oxyhydr)oxides.52–55 Depending on the kind of organic ligand, organically complexed Fe(III) can undergo photochemical reactions (i) by direct photon absorption and ligand-to-metal charge transfer (LMCT), at which an electron is transferred from the ligand to the Fe(III),44,52,56 or (ii) by indirect, secondary reactions with photochemically produced radicals such as superoxide.44,57–59
The type of organic ligand and its specific functional groups control the overall photochemical reactivity towards LMCT reactions of the dissolved complexes.44,60 For instance, the carboxylate group, which is a common functional group of dissolved organic compounds such as citrate or oxalate, is able to complex Fe(III) and to undergo photochemical reactions by photo-induced charge transfer.52,61–63 Organic ligands can also interact with Fe(III) (oxyhydr)oxide mineral or colloidal surfaces, where a photochemically mediated charge transfer from the ligand to the surface Fe(III) leads first to a reduction of Fe(III) to Fe(II), followed by either a re-oxidation of Fe(II) or by a dissociation of the formed Fe(II) from the surface leading to mineral dissolution.44,64–68 Photoreductive dissolution rates of Fe(III) (oxyhydr)oxides and colloidal Fe particles generally decrease with increasing pH.64 For photoreductive mineral dissolution, organic complexation of Fe(III) is not a necessary prerequisite. However, the photochemically induced reduction of inorganic Fe(III)–hydroxo complexes on mineral surfaces by a charge transfer from the surface hydroxide ion to the Fe(III) proceeds less efficient and only at acidic pH.64,65,67–71 Therefore, photoreduction of inorganic Fe(III)–hydroxo complexes is not a significant source of Fe(II) in waters above pH 6.5.72
The rate of photochemical LMCT reactions depends on intrinsic properties of the Fe(III) complex, temperature, pH and ionic strength of the surrounding environment, as well as on the intensity and wavelength of the absorbed light.52,56,66,71,73–77 Higher light intensities and lower wavelengths lead to faster Fe(III) photoreduction rates.66,69,71,74,78 Thus, light below certain wavelengths with sufficient energy is necessary to induce the photochemical LMCT reaction from a ligand to Fe(III), depending on the molecular structure of the complex. UV and lower wavelengths of the VIS region (<520 nm) of the solar spectrum are able to photochemically reduce Fe(III) complexes.69,79
Reduction of Fe(III) in natural waters at circumneutral pH by photochemically produced radicals is most likely mediated by superoxide by either the reduction of a Fe(III)–ligand complex (Fe(III)–L) to a Fe(II)–ligand complex (Fe(II)–L) (eqn (1)) or by the reduction of Fe(III) which dissociated from a ligand prior to reduction by superoxide (eqn (2)) (Fig. 3).57–59,80 Superoxide forms in oxic waters by photochemical reaction of DOM with O2,43,81 it can be produced by marine phytoplankton80,82,83 or during reaction of Fe(II) with O2 (eqn (3)) (Fig. 3).84,85
| Fe(III)–L + O2−˙ → Fe(II)–L + O2 | (1) |
| Fe(III) + O2−˙ → Fe(II) + O2 | (2) |
| Fe(II) + O2 → Fe(III) + O2−˙ | (3) |
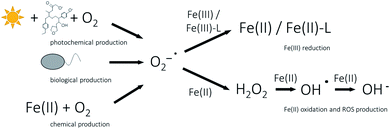 |
| Fig. 3 Superoxide (O2−˙) production processes via photochemical reaction of DOM with O2, biological production by marine phytoplankton or during the oxidation of Fe(II) by O2 as well as superoxide consumption processes via Fe(III) reduction or Fe(II) oxidation. | |
Superoxide can not only reduce Fe(III), but can also serve as oxidant of Fe(II) forming hydrogen peroxide (eqn (4)), which in turn forms hydroxyl radicals after reaction with Fe(II) (Fenton reaction, eqn (5)). Hydroxyl radicals can also oxidize Fe(II) forming hydroxide ions (eqn (6)) (Fig. 3).84,86
| Fe(II) + O2−˙ + 2H+ → Fe(III) + H2O2 | (4) |
| Fe(II) + H2O2 → Fe(III) + OH˙ + OH− | (5) |
| Fe(II) + OH˙ → Fe(III) + OH− | (6) |
As superoxide has the ability to act as both, oxidant or reductant, it plays an important role in the cycling of Fe.86 Voelker et al.58 pointed out that superoxide mediated reduction of dissolved Fe(III) is slower than oxidation of Fe(II) at seawater pH. While on the one hand at low concentrations of dissolved Fe(III), superoxide might accelerate Fe(II) oxidation, it can on the other hand retard Fe(II) oxidation at higher concentrations of dissolved Fe(III).86 The kind of organic complexation presumably controls the reactivity of Fe(III) towards superoxide.87,88 As superoxide is highly reactive towards other redox-active components, dissolved species such as copper or organic material can have a significant influence on the superoxide mediated Fe redox reaction kinetics.86
Light energy is able to photo-oxidize dissolved Fe(II) to dissolved Fe(III) forming dihydrogen gas (eqn (7)).
| 2Feaq2+ + 2H+ + hν → 2Feaq3+ + H2 | (7) |
While at acidic pH the photo-oxidation of Feaq2+ requires UV light with wavelengths in the range of 200–300 nm,89–91 the reaction at circumneutral pH is induced also by UV light with higher wavelengths due to the formation of the complex Fe(OH)+, which is sensitive to wavelengths >300 nm.90,92–94 However, it was shown that this reaction does not produce significant amounts of Fe(III) at seawater conditions.91 Due to absorption in the atmosphere, e.g. by ozone,5 only a low flux of UV is reaching the surface of the Earth and the oceans and due to only small concentrations of Feaq2+ in oxic waters, the impact of photooxidation of Fe(II) in the water column and in sediments is presumably negligible.
Fe(III) photoreduction in natural waters
Concentrations of dissolved Fe in oceans are extremely low (pico- to nanomolar range)95–98 and Fe is a limiting nutrient for primary production in many regions of the open ocean.44,95,99,100 The thermodynamically stable form of Fe in oxic seawater, Fe(III), precipitates quickly as Fe(III) (oxyhydr)oxides at circumneutral pH and is therefore removed from the water column.44,52 In regions without continental or atmospheric input, Fe concentrations were shown to be only about 100 pM.44 More than 99.9% of the dissolved Fe (<0.4 μm) in seawater is present in form of colloids (0.02–0.4 μm) or associated with biologically derived organic ligands of largely unknown identity,44,53,60,96,101–103 which keep Fe in solution and control the photochemical reactivity of Fe.44,53,104–106 Due to their small size, colloids remain suspended, even though they are distinct from water via a surface boundary.107 Most of Fe colloids in the ocean are also complexed by organic ligands108,109 and can contribute up to 90% of the total dissolved Fe pool.107,110–112 Due to their particle structure, Fe colloids may have different chemical composition and behavior such as often lower bioavailability than truly soluble Fe (<0.02 μm).107,113 Xing et al.114 only recently showed that superoxide is able to reduce colloidal Fe(III). The organic compounds in seawater binding to iron are a mixture of different molecules including polysaccharides and humic substances.115,116 A part of these Fe-binding ligands presumably are siderophores,78,96,101,104 that were produced and excreted by microorganisms for Fe acquisition under Fe-limiting conditions.117,118 Siderophores strongly bind to Fe(III) and solubilize it from Fe minerals or colloids.119,120 Depending on the functional groups binding to Fe(III), Fe(III)–siderophore complexes containing α-hydroxy carboxylate groups can undergo direct LMCT reactions leading to a reduction of Fe(III) to Fe(II) and an oxidation of the siderophore.104 Extensive studies on siderophores and their photochemical reactivity based on functional groups can be found in the literature.44,104,115,121–124 The photoproduced Fe(II) can subsequently be complexed with an Fe(II) ligand (Fe(II)–L) present in seawater. Organic ligands do not only influence the photochemical but also the redox behavior of Fe(II). Depending on the characteristics, molecular composition and origin of the organic ligands, oxidation rates of Fe(II) by O2 or H2O2 can be accelerated or slowed down.125–128 For instance, complexation of Fe(II) by more aromatic humic substances has been shown to slow down Fe(II) oxidation.125 Therefore, organic complexation of Fe(II) can lead to a stabilization of Fe(II) in water with the organic ligands serving as redox buffer and complexant and retain elevated Fe(II)–L concentrations in surface waters.125,129,130
Fe(II)–L or Feaq2+ can be taken up by microorganisms.78 The chemical and physical speciation of Fe determines its bioavailability.54,60 Photochemically induced reduction of Fe(III) forms Feaq2+, Fe(II)–L and more reactive Fe species, which are generally more bioavailable and are therefore an important nutrient source for phytoplankton in the oceans.54,131–133 Instead of being taken up by microorganisms, Fe(II) can also rapidly be re-oxidized, e.g. by O2 or H2O2,57,72,78,84,134,135 which are the main oxidants of Fe(II) in seawater.84,136 This re-oxidation of Fe(II) yields Fe(III) and the formation of ROS, which can further oxidize Fe(II) or reduce Fe(III) (eqn (1)–(6)), leading to rapid, light induced cycling of Fe in the oceans.54,55,57,78
Besides direct LMCT reactions, Fe(III)–organic complexes can also undergo indirect photochemical reactions involving photochemically produced radical species such as superoxide, which is the most likely reductant of Fe(III) at seawater pH.44,57–59,137 As superoxide is formed by photochemical reactions of DOM with O2,43,81 the contribution of the indirect photochemical reduction of Fe(III) via superoxide to the overall Fe(II) concentrations of the ocean is determined by the chemical composition of DOM present in seawater.44,54,74 Xing et al.114 only recently found that superoxide mediated Fe(III) reduction mainly is important in natural waters with limited concentrations of Fe binding ligands. In general, the interplay of both Fe(III) reduction pathways, direct LMCT reactions and indirect Fe(III) reduction involving superoxide, maintain the Fe(II) pool of the oceans.58,104 The actual Fe(III) photoreduction rates in the ocean depend on the photosusceptibility of the different organic complexes as well as light intensity and wavelength of the incoming sunlight.61,71 Although UVB light is most efficient for Fe(III) photoreduction,138,139 the UVA and VIS regions are quantitatively more important for photochemical Fe(II) production in the ocean due to their lower atmospheric attenuation and their deeper penetration into the water column of the ocean compared to UVB light.139
Despite fast oxidation of Fe(II) by O2, H2O2 or other oxidants in the oceans, a steady state concentration of Fe(II) can build up if rates of light-induced Fe(III) reduction are high enough.52,55,104,140 While Fe(III) photoreduction leads to significant Fe(II) concentrations in seawater following diurnal cycles131,138,141 and elevated Fe(II) concentrations near the water surface,55,74,96 the combination of both, Fe(III) photoreduction and Fe(II) oxidation by O2 and ROS, largely control the Fe(II) concentrations in irradiated seawater.57
The photochemically induced reduction of Fe(III) does not only drive Fe cycling in the oceans, but also plays an important role for Fe(II) availability in freshwater systems.74,85,142 Usually, Fe concentrations and DOM in coastal waters but also in freshwater lakes are higher than in the open ocean due to larger particulate inputs,74,82,143 with dissolved Fe almost entirely being present in organic complexes.54,85,142 In circumneutral lakes, Fe(III) photoreduction leads to diel cycling of Fe(II) with maximum concentrations close to the water surface similar as in the ocean.74,142 Both, light-induced LMCT reactions and superoxide mediated reduction of Fe(III) are responsible for the photoproduced Fe(II) concentrations in the investigated circumneutral freshwater lakes.74,85 The molecular weight of DOM present influences the extent of Fe(III) photoreduction.54,85
Fe(III) photoreduction in anthropogenically altered waters
Anthropogenic influences such as acid main drainage can lower the pH of surface waters leading typically to pH values between 2 and 4.76 As the rate of Fe(III) photoreduction generally increases with decreasing pH,71,74 Fe(III) photoreduction is an important Fe(II) source in acidic rivers and lakes. Due to slower abiotic Fe(II) oxidation rates by O2 at low pH,144 higher dissolved Fe(II) concentrations can build up in these waters compared to waters with circumneutral pH, where abiotic Fe(II) oxidation to Fe(III) by O2 and quick precipitation as Fe(III) (oxyhydr)oxides lead to a removal of Fe from the water column.52,76,145 Waters influenced by acid mine drainage often have low concentrations of DOM,146,147 which lead to low photochemical production of superoxide and H2O2.146,148 This would imply that direct LCMT reactions are the more important photochemical Fe(III) reduction pathway in acidic waters. Due to low pH and low DOM concentrations, photoreduction of dissolved inorganic Fe(III) species and photoreductive dissolution of particulate Fe(III) species or Fe(III) (oxyhydr)oxides play a relatively larger role in these environments73,76,147 than in circumneutral waters. At low pH, the dominant dissolved inorganic Fe(III) species is Fe(III)OH2+
75,148 and a light-induced LMCT induced by UV-light generates Fe(II) and hydroxyl radicals.62,147 During photoreductive mineral dissolution, formerly adsorbed phosphate or trace metals such as Cu, Zn, As or Pb145,146,149,150 are released into the water and can impact the activity of aquatic biota76 on a relatively short time scale. With increasing pH, e.g. in rivers with a downstream pH gradient, dissolved Feaq2+ is oxidized to Fe(III) and usually precipitates as Fe(III) (oxyhydr)oxides, resulting in the scavenging of trace metals due to sorption processes.145,150 Fe(II) concentrations in acidic rivers and lakes also follow diel cycles75,151–153 correlated with light intensity146,152,154 with maximum concentrations at midday and minimum concentrations at night. The amounts of Fe(II) photochemically produced in acidic waters can be sufficient to serve as substrate for populations of Fe(II)-oxidizing bacteria154 as slow abiotic Fe(II) oxidation kinetics leads to longer residence times of Fe(II) in the water. If no other external sources of Fe(II) exist, e.g. supplied by inflow of anoxic groundwater or oxidation of pyrite,154,155 Fe(III) photoreduction represents an important and also renewable Fe(II) source in acidic surface waters.154 Dominant photochemical processes involving Fe(III) and the fate of produced Feaq2+ in a river with a downstream pH gradient are illustrated in Fig. 4.
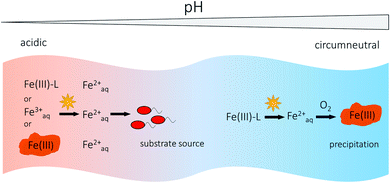 |
| Fig. 4 River with downstream pH gradient showing dominant Fe processes in waters with acidic or circumneutral pH. At acidic pH, dissolved organically complexed (Fe(III)–L), dissolved inorganic Fe(III) species (Feaq3+) or Fe(III) (oxyhydr)oxides can photochemically be reduced to Feaq2+, which can, due to high concentrations, be used as substrate for Fe(II)-oxidizing bacteria. At circumneutral pH, dissolved Fe(III) occurs organically complexed and photoproduced Feaq2+ is mostly re-oxidized, e.g. by O2, and predominantly precipitates as Fe(III) (oxyhydr)oxides. | |
Sedimentary Fe(III) photoreduction
Only recently, Feaq2+ formed by Fe(III) photoreduction in the porewater of freshwater and marine sediments was quantified.156,157 In sediments, Fe concentrations usually are in the range of micromolar concentrations158–160 and therefore Fe is generally not considered to be a limiting nutrient. However, gradients of Fe(II) establish at a millimeter to centimeter scale with increasing concentrations downwards due to chemical redox processes and microbially catalyzed reactions, including Fe(III) reduction in deeper, anoxic as well as abiotic Fe(II) oxidation by O2 in shallow, oxic sediment layers.156,157,160 Fe-metabolizing bacteria that use Fe for gaining electrons and energy, need Fe concentrations above the trace element level for growth. In the upper millimeters of light-influenced, oxic sediment layers, Fe(III) photoreduction produces substantial amounts (micromolar range) of Feaq2+, where it is usually limited as substrate for growth.156 The produced Feaq2+ persists even in the presence of O2, probably due to stabilization by organic matter, which can slow down Fe(II) oxidation rates.85,147,161 By this, Fe(III) photoreduction has a strong impact on Fe(II) gradients in sediments and presumably has consequences for the inhabiting microbial community of Fe-metabolizing bacteria, which cannot only oxidize free, dissolved Feaq2+ but also Fe(II)–organic complexes.162–164 Also in sediments, the extent of Fe(III) photoreduction is determined by the DOM concentration of the sediment porewater.156 So far, the relative contribution of direct LMCT and reduction of Fe(III) via photochemically produced superoxide to the overall photochemical Fe(II) production in sediments was not determined yet. However, photochemical production of superoxide and ROS in sediments can be expected by photolysis of dissolved DOM of the porewater, especially in organic rich sediments. While in water columns, photochemical reduction of Fe(III) mainly controls primary production by delivering bioavailable Feaq2+ as a nutrient, it substantially produces Feaq2+ as additional Feaq2+ source serving as substrate for growth for Fe(II)-oxidizing bacteria besides Feaq2+ originating from the Fe(III) reduction zone. Thereby, Fe(III) photoreduction changes Feaq2+ gradients and fluxes in sediments.156Fig. 5 summarizes sources and fate of Fe(II) in ocean and sediments.
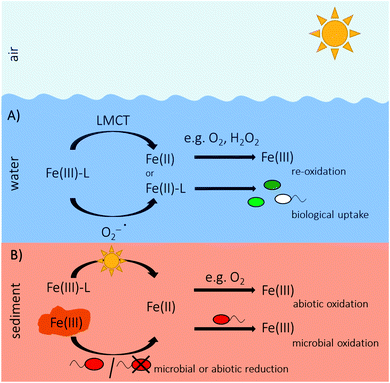 |
| Fig. 5 (A) Illustration of photoreduction in the water column of organically complexed Fe(III) (Fe(III)–L) via ligand-to-metal-charge transfer (LMCT) reaction or via superoxide(O2−˙) forming Fe(II), which either gets re-oxidized by e.g. O2 or H2O2 to Fe(III) or is taken up by phytoplankton. (B) Sketch of Fe(II) production in sediments by either Fe(III) photoreduction or by biotic or abiotic Fe(III) reduction. Fe(II) either gets abiotically re-oxidized, e.g. by O2 or serves as substrate for microaerophilic, phototrophic or nitrate-reducing Fe(II)-oxidizing bacteria. | |
Light as driver of microbial Fe cycling
Besides the purely chemical process of Fe(III) photoreduction, light can also serve as energy source for the metabolism of anoxygenic phototrophic Fe(II)-oxidizing bacteria present in freshwater or marine sediments159,165 or in water columns166,167 which thereby couple the oxidation of Fe(II) to CO2 fixation.22 Anoxygenic photosynthesis was suggested to be an important metabolism in ancient environments of the Archean Ocean where O2 was absent and reduced species such as Fe(II) dominated in the water column.168,169 It probably accounted for most of the primary production in sunlit waters.170 With the evolution of oxygenic photosynthesis, today's mostly oxic aquatic environments formed and anoxygenic photoferrotrophs were displaced to anoxic, sunlit niches. However, they are still present as primary producers in many environments today and are part of the biogeochemical cycling of Fe.25 The production of O2 by oxygenic photosynthesis also has consequences for other Fe-metabolizing bacteria that either have anaerobic metabolisms or need to compete with the fast abiotic Fe(II) oxidation kinetics with O2 at circumneutral pH. In contrast, the oxidation kinetics of Fe(II) by O2 in acidic conditions is much slower144 and in acidic waters, Fe(III) photoreduction produces significant amounts of Fe(II) and can thus control the presence and distribution of Fe(II)-oxidizing acidophilic bacteria146 due to longer half-life times of Fe(II) at acidic pH.144 The discovery of Fe(III) photoreduction in freshwater and marine sediments adds another Fe(II) source, besides biotic and abiotic Fe(III) reduction, to the sedimentary biogeochemical Fe cycle.156 By supplying light-influenced sediments with Fe(II) concentrations in the micromolar range, microaerophilic Fe(II)-oxidizing bacteria can grow in oxic sediment layers, in which rapid abiotic Fe(II) oxidation by O2 would otherwise dominate due to the rapid oxidation kinetics at circumneutral pH.171 Light is therefore driving the biogeochemical cycling of Fe in modern aquatic environments and sediments by not only serving as energy source for phototrophic Fe(II)-oxidizers but also providing substantial amounts of Fe(II) for other Fe(II)-oxidizing bacteria.
ROS related processes in the dark
Sunlight is directly or indirectly driving many chemical and biological reactions in natural waters and sediments. However, most of our ecosystems on Earth are never reached by light and only exist in darkness – the deep biosphere.172 However, even in the dark, there are parallel processes compared to photochemical mechanisms that involve, among others, the formation and consumption of ROS species.
Due to their high temperatures, hydrothermal vents emit light into the dark of the ocean, mainly as thermal radiation in the IR wavelength region (>700 nm), but temporally also in small intensity in the VIS wavelength region.173,174 The energy of this radiation can potentially be used by photosynthetic bacteria such as green sulphur bacteria, which are potentially able to oxidize Fe(II), for the fixation of CO2 to organic carbon (Fig. 6).175 Apart from being used for photosynthesis, IR presumably does not deliver sufficient energy for the formation of ROS in the environment as photolysis of DOM and concomitant ROS production usually occurs by absorption of UV and VIS light.16,26,176
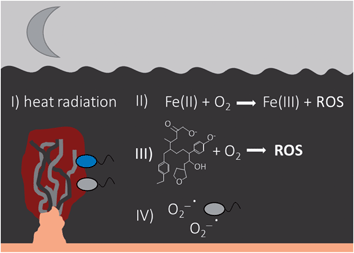 |
| Fig. 6 Illustration of reactions proceeding in darkness (I) IR light emission at hydrothermal vents, which may be used for anoxygenic photosynthesis, (II) Fenton reaction forming ROS, (III) ROS formation by oxidation of reduced organic molecules, (IV) extracellularly production of superoxide by bacteria or phytoplankton. | |
The aeration of anoxic water containing Fe(II) leads to the formation of hydroxyl radicals by Fenton reactions (eqn (5)),177,178 as the oxidation of Fe(II) by O2 also proceeds in darkness producing H2O2 and further hydroxyl radicals (eqn (4) and (5)). In general, biotic reductive processes (e.g. Fe(III)-reducing bacteria such as Shewanella oneidensis) or abiotic reductants (e.g. sulphide) that are able to reduce Fe(III) to Fe(II), enhance the formation of ROS, as was observed in natural waters or marsh sediments.179–182 The formed Fe(II) further reacts as described in eqn (3)–(6) with O2 forming ROS (Fig. 6). These reactions involving O2 are important e.g. at oxic–anoxic interfaces or anoxic soils or sediments that are flushed with oxygenated water.183 During abiotic or biotic Fe(III) reduction, the reducing equivalents are not photons, but they are produced in chemical and biological reactions, which have a similar impact on ROS production as photochemical sources.179 Besides the Fenton-like reactions yielding ROS, the abiotic oxidation of reduced DOC or humic acids by O2 forms ROS as well (Fig. 6).177,183 Superoxide is also extracellularly produced by several microorganisms such as different heterotrophic bacteria or phytoplankton (Fig. 6).80,82,83,184,185 This light-independent biological production of superoxide in marine and freshwater systems is a significant source of ROS concentrations as measured in sunlit waters.186 Those reactions also proceeding in darkness are similar important drivers of biogeochemical cycles as photochemically-induced processes.
Conclusions
Photochemical reduction of Fe(III) by either direct LMCT reactions or by photochemically produced superoxide plays a major role in the Fe cycle of aquatic environments. It is the main source for the production of Feaq2+ in water bodies such as the ocean and thus strongly influences the primary productivity of marine systems by supplying Feaq2+. Besides biological uptake, rapid chemical oxidation of photochemically formed Fe(II), mainly by O2 or H2O2,84,136 closes this photochemically driven diel Fe cycle in the oceans.55,131,187 Fe(III) photoreduction also is a major source of ROS (eqn (1)–(6)), which are key oxidants in natural waters71,188 playing an important role in the biogeochemical cycling of trace metals and carbon.189,190 In acidic waters or sediments, Fe(III) photoreduction can even provide micromolar concentrations of Feaq2+ and influence the presence and abundance of different Fe(II)-oxidizing bacteria. Photochemical effects generally decrease with water or sediment depth as light is attenuated depending on the wavelength.56 Especially light in the UV region, which is not only very efficient for Fe(III) photoreduction but also for the photochemical production of radicals,43,44 is strongly attenuated in both, water and sediments. Photochemical processes thus strongly impact and control the biological life and biotic processes in natural waters of different pH and in sediments, mainly by supplying bioavailable Fe(II) as nutrient or substrate for growth to the inhabiting organisms. Without those light-induced chemical processes, other element cycles such as the carbon cycle would lack an important driver.
Conflicts of interest
There are no conflicts to declare.
Acknowledgements
C. S. received funding from a Margarete von Wrangell fellowship (Ministry of Baden-Württemberg, Germany) and the DFG grant (SCHM2808/4-1).
References
- G. D. Scholes, G. R. Fleming, A. Olaya-Castro and R. van Grondelle, Lessons from nature about solar light harvesting, Nat. Chem., 2011, 3, 763 CrossRef CAS PubMed.
- M. S. Dresselhaus and I. L. Thomas, Alternative energy technologies, Nature, 2001, 414, 332–337 CrossRef CAS PubMed.
- D. W. Ball, The electromagnetic spectrum: a history, Spectroscopy, 2007, 22, 14–17 Search PubMed.
- R. G. Zepp and D. M. Cline, Rates of direct photolysis in aquatic environment, Environ. Sci. Technol., 1977, 11, 359–366 CrossRef CAS.
- H. Horvath, Atmospheric light absorption—A review, Atmos. Environ., Part A, 1993, 27, 293–317 CrossRef.
- D. Karentz, Impact of UV-B radiation on pelagic freshwater ecosystems: Report of working group on bacteria and phytoplankton, Adv. Limnol., 1994, 43, 31–69 Search PubMed.
- H. Piazena, E. Perez-Rodrigues, D. P. Häder and F. Lopez-Figueroa, Penetration of solar radiation into the water column of the central subtropical Atlantic Ocean—optical properties and possible biological consequences, Deep Sea Res., Part II, 2002, 49, 3513–3528 CrossRef CAS.
- R. H. Grant and G. M. Heisler, Estimation of ultraviolet-B irradiance under variable cloud conditions, J. Appl. Meteorol., 2000, 39, 904–916 CrossRef.
- R. A. Larson and M. R. Berenbaum, Environmental phototoxicity, Environ. Sci. Technol., 1988, 22, 354–360 CrossRef CAS.
- M. Nunez, B. Forgan and C. Roy, Estimating ultraviolet radiation at the earth's surface, Int. J. Biometeorol., 1994, 38, 5–17 CrossRef.
- D. W. Ball, Light: particle or wave?, Spectroscopy, 2006, 21, 30–33 Search PubMed.
-
J. Masojídek and G. Torzillo, Mass cultivation of freshwater microalgae, in Encyclopedia of Ecology, ed. S. E. Jørgensen and B. D. Fath, Academic Press, Oxford, 2008, pp. 2226–2235 Search PubMed.
- N. Y. Kiang, J. Siefert, Govindjee and R. E. Blankenship, Spectral signatures of photosynthesis I: Review of earth organisms, Astrobiology, 2007, 7, 222–251 CrossRef CAS PubMed.
- R. C. Smith and K. S. Baker, Optical properties of the clearest natural waters (200–800 nm), Appl. Opt., 1981, 20, 177–184 CrossRef CAS PubMed.
- S. Q. Duntley, Light in the sea, J. Opt. Soc. Am., 1963, 53, 214–233 CrossRef.
- S. C. Johannessen, W. L. Miller and J. J. Cullen, Calculation of UV attenuation and colored dissolved organic matter absorption spectra from measurements of ocean color, J. Geophys. Res.: Oceans, 2003, 108(C9), 3301 CrossRef.
- M. Kühl, C. Lassen and B. B. Jørgensen, Light penetration and light intensity in sandy marine sediments measured with irradiance and scalar irradiance fiber-optic microprobes, Mar. Ecol.: Prog. Ser., 1994, 105, 139–148 CrossRef.
- F. Garcia-Pichel, A scalar irradiance fiber-optic microprobe for the measurement of ultraviolet radiation at high spatial resolution, Photochem. Photobiol., 1995, 61, 248–254 CrossRef.
- F. Garcia-Pichel and B. M. Bebout, Penetration of ultraviolet radiation into shallow water sediments: high exposure for photosynthetic communities, Mar. Ecol.: Prog. Ser., 1996, 131, 257–262 CrossRef.
- V. F. Farjalla, A. M. Amado, A. L. Suhett and F. Meirelles-Pereira, DOC removal paradigms in highly humic aquatic ecosystems, Environ. Sci. Pollut. Res., 2009, 16, 531–538 CrossRef CAS PubMed.
- R. van Grondelle, J. P. Dekker, T. Gillbro and V. Sundstrom, Energy transfer and trapping in photosynthesis, Biochim. Biophys. Acta, 1994, 1187, 1–65 CrossRef.
- F. Widdel, S. Schnell, S. Heising, A. Ehrenreich, B. Assmus and B. Schink, Ferrous iron oxidation by anoxygenic phototrophic bacteria, Nature, 1993, 362, 834–836 CrossRef CAS.
- V. V. Yurkov and J. T. Beatty, Aerobic anoxygenic phototrophic bacteria, Microbiol. Mol. Biol. Rev., 1998, 62, 695–724 CrossRef CAS PubMed.
- H. Larsen, On the culture and general physiology of the green sulfur bacteria, J. Bacteriol., 1952, 64, 187–196 CrossRef CAS PubMed.
- C. Bryce, N. Blackwell, C. Schmidt, J. Otte, Y.-M. Huang, S. Kleindienst, E. Tomaszewski, M. Schad, V. Warter, C. Peng, J. M. Byrne and A. Kappler, Microbial anaerobic Fe(II) oxidation – Ecology, mechanisms and environmental implications, Environ. Microbiol., 2018, 20, 3462–3483 CrossRef CAS PubMed.
- N. M. Scully, W. J. Cooper and L. J. Tranvik, Photochemical effects on microbial activity in natural waters: the interaction of reactive oxygen species and dissolved organic matter, FEMS Microbiol. Ecol., 2003, 46, 353–357 CrossRef CAS PubMed.
- M. A. Moran and R. G. Zepp, Role of photoreactions in the formation of biologically labile compounds from dissolved organic matter, Limnol. Oceanogr., 1997, 42, 1307–1316 CrossRef CAS.
- L. M. Mayer, L. L. Schick, K. Skorko and E. Boss, Photodissolution of particulate organic matter from sediments, Limnol. Oceanogr., 2006, 51, 1064–1071 CrossRef CAS.
- W. L. Miller and M. A. Moran, Interaction of photochemical and microbial processes in the degradation of refractory dissolved organic matter from a coastal marine environment, Limnol. Oceanogr., 1997, 42, 1317–1324 CrossRef CAS.
- A. Geller, Light-induced conversion of refractory, high molecular weight lake water constituents, Swiss J. Hydrol., 1985, 47, 21–26 CrossRef CAS.
- W. L. Miller and R. G. Zepp, Photochemical production of dissolved inorganic carbon from terrestrial organic matter: Significance to the oceanic organic carbon cycle, Geophys. Res. Lett., 1995, 22, 417–420 CrossRef CAS.
- C. J. Miles and P. L. Brezonik, Oxygen consumption in humic-colored waters by a photochemical ferrous-ferric catalytic cycle, Environ. Sci. Technol., 1981, 15, 1089–1095 CrossRef CAS PubMed.
- D. J. Kieber, J. McDaniel and K. Mopper, Photochemical source of biological substrates in sea water: implications for carbon cycling, Nature, 1989, 341, 637–639 CrossRef CAS.
- S. Bertilsson and L. J. Stefan, Photochemically produced carboxylic acids as substrates for freshwater bacterioplankton, Limnol. Oceanogr., 1998, 43, 885–895 CrossRef CAS.
- R. Benner and B. Biddanda, Photochemical transformations of surface and deep marine dissolved organic matter: Effects on bacterial growth, Limnol. Oceanogr., 1998, 43, 1373–1378 CrossRef CAS.
- L. J. Tranvik and S. Bertilsson, Contrasting effects of solar UV radiation on dissolved organic sources for bacterial growth, Ecol. Lett., 2001, 4, 458–463 CrossRef.
- J. A. Riggsbee, C. H. Orr, D. M. Leech, M. W. Doyle and R. G. Wetzel, Suspended sediments in river ecosystems: Photochemical sources of dissolved organic carbon, dissolved organic nitrogen, and adsorptive removal of dissolved iron, J. Geophys. Res.: Biogeosci., 2008, 113, G03019 Search PubMed.
- M. W. Southwell, R. N. Mead, C. M. Luquire, A. Barbera, G. B. Avery, R. J. Kieber and S. A. Skrabal, Influence of organic matter source and diagenetic state on photochemical release of dissolved organic matter and nutrients from resuspendable estuarine sediments, Mar. Chem., 2011, 126, 114–119 CrossRef CAS.
- R. J. Kieber, R. F. Whitehead and S. A. Skrabal, Photochemical production of dissolved organic carbon from resuspended sediments, Limnol. Oceanogr., 2006, 51, 2187–2195 CrossRef CAS.
- R. G. Zepp, B. C. Faust and J. Hoigne, Hydroxyl radical formation in aqueous reactions (pH 3-8) of iron(II) with hydrogen peroxide: the photo-Fenton reaction, Environ. Sci. Technol., 1992, 26, 313–319 CrossRef CAS.
- S. L. H. Sandvik, P. Bilski, J. D. Pakulski, C. F. Chignell and R. B. Coffin, Photogeneration of singlet oxygen and free radicals in dissolved organic matter isolated from the Mississippi and Atchafalaya River plumes, Mar. Chem., 2000, 69, 139–152 CrossRef CAS.
- N. M. Scully, D. J. McQueen and D. R. S. Lean, Hydrogen peroxide formation: The interaction of ultraviolet radiation and dissolved organic carbon in lake waters along a 43–75°N gradient, Limnol. Oceanogr., 1996, 41, 540–548 CrossRef CAS.
- S. Garg, A. L. Rose and T. D. Waite, Photochemical production of superoxide and hydrogen peroxide from natural organic matter, Geochim. Cosmochim. Acta, 2011, 75, 4310–4320 CrossRef CAS.
- K. Barbeau, Photochemistry of organic iron(III) complexing ligands in oceanic systems, Photochem. Photobiol., 2006, 82, 1505–1516 CrossRef CAS PubMed.
- S. E. Page, J. R. Logan, R. M. Cory and K. McNeill, Evidence for dissolved organic matter as the primary source and sink of photochemically produced hydroxyl radical in arctic surface waters, Environ. Sci.: Processes Impacts, 2014, 16, 807–822 RSC.
- B. M. Voelker, D. L. Sedlak and O. C. Zafiriou, Chemistry of superoxide radical in seawater: reactions with organic Cu complexes, Environ. Sci. Technol., 2000, 34, 1036–1042 CrossRef CAS.
- J.-A. Marshall, T. Ross, S. Pyecroft and G. Hallegraeff, Superoxide production by marine microalgae, Mar. Biol., 2005, 147, 541–549 CrossRef CAS.
- J. V. Goldstone, M. J. Pullin, S. Bertilsson and B. M. Voelker, Reactions of hydroxyl radical with humic substances: bleaching, mineralization, and production of bioavailable carbon substrates, Environ. Sci. Technol., 2002, 36, 364–372 CrossRef CAS PubMed.
- S. Baral, C. Lume-Pereira, E. Janata and A. Henglein, Chemistry of colloidal manganese dioxide. 2. Reaction with superoxide anion (O2−) and hydrogen peroxide (pulse radiolysis and stop flow studies), J. Phys. Chem., 1985, 89, 5779–5783 CrossRef CAS.
- W. G. Sunda, S. A. Huntsman and G. R. Harvey, Photoreduction of manganese oxides in seawater and its geochemical and biological implications, Nature, 1983, 301, 234–236 CrossRef CAS.
- W. G. Sunda and S. A. Huntsman, Photoreduction of manganese oxides in seawater, Mar. Chem., 1994, 46, 133–152 CrossRef CAS.
- K. Kuma, S. Nakabayashi and K. Matsunaga, Photoreduction of Fe(III) by hydroxycarboxylic acids in seawater, Water Res., 1995, 29, 1559–1569 CrossRef CAS.
- K. Kuma, J. Nishioka and K. Matsunaga, Controls on iron(III) hydroxide solubility in seawater: The influence of pH and natural organic chelators, Limnol. Oceanogr., 1996, 41, 396–407 CrossRef CAS.
- B. Sulzberger, Light-induced redox cycling of iron: Roles for CO2 uptake and release by aquatic ecosystems, Aquat. Geochem., 2015, 21, 65–80 CrossRef CAS.
- D. W. O'Sullivan, A. K. Hanson, W. L. Miller and D. R. Kester, Measurement of Fe(II) in surface water of the equatorial Pacific, Limnol. Oceanogr., 1991, 36, 1727–1741 CrossRef.
- B. M. Voelker, F. M. M. Morel and B. Sulzberger, Iron redox cycling in surface waters: effects of humic substances and light, Environ. Sci. Technol., 1997, 31, 1004–1011 CrossRef CAS.
- W. L. Miller, D. W. King, J. Lin and D. R. Kester, Photochemical redox cycling of iron in coastal seawater, Mar. Chem., 1995, 50, 63–77 CrossRef CAS.
- B. M. Voelker and D. L. Sedlak, Iron reduction by photoproduced superoxide in seawater, Mar. Chem., 1995, 50, 93–102 CrossRef CAS.
- A. L. Rose and T. D. Waite, Reduction of organically complexed ferric iron by superoxide in a simulated natural water, Environ. Sci. Technol., 2005, 39, 2645–2650 CrossRef CAS PubMed.
- M. J. A. Rijkenberg, L. J. A. Gerringa, V. E. Carolus, I. Velzeboer and H. J. W. de Baar, Enhancement and inhibition of iron photoreduction by individual ligands in open ocean seawater, Geochim. Cosmochim. Acta, 2006, 70, 2790–2805 CrossRef CAS.
- W. Feng and D. Nansheng, Photochemistry of hydrolytic iron (III) species and photoinduced degradation of organic compounds. A minireview, Chemosphere, 2000, 41, 1137–1147 CrossRef CAS.
- B. C. Faust and J. Hoigné, Photolysis of Fe (III)-hydroxy complexes as sources of OH radicals in clouds, fog and rain, Atmos. Environ., Part A, 1990, 24, 79–89 CrossRef.
- Y. Zuo and J. Hoigne, Formation of hydrogen peroxide and depletion of oxalic acid in atmospheric water by photolysis of iron(III)-oxalato complexes, Environ. Sci. Technol., 1992, 26, 1014–1022 CrossRef CAS.
- T. D. Waite and F. M. M. Morel, Photoreductive dissolution of colloidal iron oxides in natural waters, Environ. Sci. Technol., 1984, 18, 860–868 CrossRef CAS PubMed.
- B. Sulzberger, D. Suter, C. Siffert, S. Banwart and W. Stumm, Dissolution of Fe(III)(hydr)oxides in natural waters; laboratory assessment on the kinetics controlled by surface coordination, Mar. Chem., 1989, 28, 127–144 CrossRef CAS.
- B. Sulzberger and H. Laubscher, Reactivity of various types of iron(III) (hydr)oxides towards light-induced dissolution, Mar. Chem., 1995, 50, 103–115 CrossRef CAS.
- C. Siffert and B. Sulzberger, Light-induced dissolution of hematite in the presence of oxalate. A case study, Langmuir, 1991, 7, 1627–1634 CrossRef CAS.
- P. Borer, B. Sulzberger, S. J. Hug, S. M. Kraemer and R. Kretzschmar, Photoreductive dissolution of iron(III) (hydr)oxides in the absence and presence of organic ligands: experimental studies and kinetic modeling, Environ. Sci. Technol., 2009, 43, 1864–1870 CrossRef CAS PubMed.
- P. Borer, B. Sulzberger, S. J. Hug, S. M. Kraemer and R. Kretzschmar, Wavelength-dependence of photoreductive dissolution of lepidocrocite (γ-FeOOH) in the absence and presence of the siderophore DFOB, Environ. Sci. Technol., 2009, 43, 1871–1876 CrossRef CAS PubMed.
- D. M. Sherman, Electronic structures of iron(III) and manganese(IV) (hydr)oxide minerals: Thermodynamics of photochemical reductive dissolution in aquatic environments, Geochim. Cosmochim. Acta, 2005, 69, 3249–3255 CrossRef CAS.
- W. D. King, R. A. Aldrich and S. E. Charnecki, Photochemical redox cycling of iron in NaCl solutions, Mar. Chem., 1993, 44, 105–120 CrossRef.
- D. W. King, H. A. Lounsbury and F. J. Millero, Rates and mechanism of Fe(II) oxidation at nanomolar total iron concentrations, Environ. Sci. Technol., 1995, 29, 818–824 CrossRef CAS PubMed.
- T. D. Waite and F. M. M. Morel, Photoreductive dissolution of colloidal iron oxide: Effect of citrate, J. Colloid Interface Sci., 1984, 102, 121–137 CrossRef CAS.
- L. Emmenegger, R. Schönenberger, L. Sigg and B. Sulzberger, Light-induced redox cycling of iron in circumneutral lakes, Limnol. Oceanogr., 2001, 46, 49–61 CrossRef CAS.
- R. H. Collienne, Photoreduction of iron in the epilimnion of acidic lakes, Limnol. Oceanogr., 1983, 28, 83–100 CrossRef CAS.
- D. M. McKnight, B. A. Kimball and R. L. Runkel, pH dependence of iron photoreduction in a rocky mountain stream affected by acid mine drainage, Hydrol. Processes, 2001, 15, 1979–1992 CrossRef.
- F. David and P. G. David, Photoredox chemistry of iron(III) chloride and iron(III) perchlorate in aqueous media. A comparative study, J. Phys. Chem., 1976, 80, 579–583 CrossRef CAS.
- K. Barbeau, E. L. Rue, K. W. Bruland and A. Butler, Photochemical cycling of iron in the surface ocean mediated by microbial iron(III)-binding ligands, Nature, 2001, 413, 409–413 CrossRef CAS PubMed.
- H. W. Rich and F. M. M. Morel, Availability of well-defined iron colloids to the marine diatom Thalassiosira weissflogii, Limnol. Oceanogr., 1990, 35, 652–662 CrossRef CAS.
- A. B. Kustka, Y. Shaked, A. J. Milligan, D. W. King and F. M. M. Morel, Extracellular production of superoxide by marine diatoms: Contrasting effects on iron redox chemistry and bioavailability, Limnol. Oceanogr., 2005, 50, 1172–1180 CrossRef CAS.
-
W. J. Cooper, R. G. Zika, R. G. Petasne and A. M. Fischer, Sunlight-induced photochemistry of humic substances in natural waters: major reactive species, in Aquatic humic substances, American Chemical Society, 1988, vol. 219, ch. 22, pp. 333–362 Search PubMed.
- A. L. Rose, T. P. Salmon, T. Lukondeh, B. A. Neilan and T. D. Waite, Use of superoxide as an electron shuttle for iron acquisition by the marine cyanobacterium lyngbya majuscula, Environ. Sci. Technol., 2005, 39, 3708–3715 CrossRef CAS PubMed.
- D. Kim, A. Nakamura, T. Okamoto, N. Komatsu, T. Oda, T. Iida, A. Ishimatsu and T. Muramatsu, Mechanism of superoxide anion generation in the toxic red tide phytoplankton Chattonella marina: possible involvement of NAD(P)H oxidase, Biochim. Biophys. Acta, Gen. Subj., 2000, 1524, 220–227 CrossRef CAS.
- J. M. Santana-Casiano, M. González-Dávila and F. J. Millero, The role of Fe(II) species on the oxidation of Fe(II) in natural waters in the presence of O2 and H2O2, Mar. Chem., 2006, 99, 70–82 CrossRef CAS.
- L. Meunier, H. Laubscher, S. J. Hug and B. Sulzberger, Effects of size and origin of natural dissolved organic matter compounds on the redox cycling of iron in sunlit surface waters, Aquat. Sci., 2005, 67, 292–307 CrossRef CAS.
- A. L. Rose and T. D. Waite, Kinetic model for Fe(II) oxidation in seawater in the absence and presence of natural organic matter, Environ. Sci. Technol., 2002, 36, 433–444 CrossRef CAS PubMed.
- P. Croot and M. Heller, The importance of kinetics and redox in the biogeochemical cycling of iron in the surface ocean, Front. Microbiol., 2012, 3, 219 Search PubMed.
- M. I. Heller and P. L. Croot, Superoxide decay as a probe for speciation changes during dust dissolution in Tropical Atlantic surface waters near Cape Verde, Mar. Chem., 2011, 126, 37–55 CrossRef CAS.
- Z. K. Borowska and D. C. Mauzerall, Efficient near ultraviolet light induced formation of hydrogen by ferrous hydroxide, Orig. Life Evol. Biosph., 1987, 17, 251–259 CrossRef CAS PubMed.
- P. S. Braterman, A. G. Cairns-Smith, R. W. Sloper, T. G. Truscott and M. Craw, Photo-oxidation of iron(II) in water between pH 7.5 and 4.0, J. Chem. Soc., Dalton Trans., 1984, 1441–1445, 10.1039/DT9840001441.
- K. O. Konhauser, L. Amskold, S. V. Lalonde, N. R. Posth, A. Kappler and A. Anbar, Decoupling photochemical Fe(II) oxidation from shallow-water BIF deposition, Earth Planet. Sci. Lett., 2007, 258, 87–100 CrossRef CAS.
- P. S. Braterman, A. G. Cairns-Smith and R. W. Sloper, Photo-oxidation of hydrated Fe2+ - significance for banded iron formations, Nature, 1983, 303, 163–164 CrossRef CAS.
- A. D. Anbar and H. D. Holland, The photochemistry of manganese and the origin of banded iron formations, Geochim. Cosmochim. Acta, 1992, 56, 2595–2603 CrossRef CAS PubMed.
- L. M. François, Extensive deposition of banded iron formations was possible without photosynthesis, Nature, 1986, 320, 352–354 CrossRef.
- P. W. Boyd, A. J. Watson, C. S. Law, E. R. Abraham, T. Trull, R. Murdoch, D. C. E. Bakker, A. R. Bowie, K. O. Buesseler, H. Chang, M. Charette, P. Croot, K. Downing, R. Frew, M. Gall, M. Hadfield, J. Hall, M. Harvey, G. Jameson, J. LaRoche, M. Liddicoat, R. Ling, M. T. Maldonado, R. M. McKay, S. Nodder, S. Pickmere, R. Pridmore, S. Rintoul, K. Safi, P. Sutton, R. Strzepek, K. Tanneberger, S. Turner, A. Waite and J. Zeldis, A mesoscale phytoplankton bloom in the polar Southern Ocean stimulated by iron fertilization, Nature, 2000, 407, 695–702 CrossRef CAS PubMed.
- P. W. Boyd and M. J. Ellwood, The biogeochemical cycle of iron in the ocean, Nat. Geosci., 2010, 3, 675 CrossRef CAS.
- C. Schlosser, C. L. De La Rocha, P. Streu and P. L. Croot, Solubility of iron in the Southern Ocean, Limnol. Oceanogr., 2012, 57, 684–697 CrossRef CAS.
- A. L. King and K. A. Barbeau, Dissolved iron and macronutrient distributions in the southern California Current System, J. Geophys. Res.: Oceans, 2011, 116, C03018 Search PubMed.
- J. H. Martin and S. E. Fitzwater, Iron deficiency limits phytoplankton growth in the north-east Pacific subarctic, Nature, 1988, 331, 341–343 CrossRef CAS.
- J. H. Martin, K. H. Coale, K. S. Johnson, S. E. Fitzwater, R. M. Gordon, S. J. Tanner, C. N. Hunter, V. A. Elrod, J. L. Nowicki, T. L. Coley, R. T. Barber, S. Lindley, A. J. Watson, K. Van Scoy, C. S. Law, M. I. Liddicoat, R. Ling, T. Stanton, J. Stockel, C. Collins, A. Anderson, R. Bidigare, M. Ondrusek, M. Latasa, F. J. Millero, K. Lee, W. Yao, J. Z. Zhang, G. Friederich, C. Sakamoto, F. Chavez, K. Buck, Z. Kolber, R. Greene, P. Falkowski, S. W. Chisholm, F. Hoge, R. Swift, J. Yungel, S. Turner, P. Nightingale, A. Hatton, P. Liss and N. W. Tindale, Testing the iron hypothesis in ecosystems of the equatorial Pacific Ocean, Nature, 1994, 371, 123–129 CrossRef CAS.
- E. L. Rue and K. W. Bruland, Complexation of iron(III) by natural organic ligands in the Central North Pacific as determined by a new competitive ligand equilibration/adsorptive cathodic stripping voltammetric method, Mar. Chem., 1995, 50, 117–138 CrossRef CAS.
- M. Gledhill and C. M. G. van den Berg, Determination of complexation of iron(III) with natural organic complexing ligands in seawater using cathodic stripping voltammetry, Mar. Chem., 1994, 47, 41–54 CrossRef CAS.
- J. Wu and G. W. Luther, Complexation of Fe(III) by natural organic ligands in the Northwest Atlantic Ocean by a competitive ligand equilibration method and a kinetic approach, Mar. Chem., 1995, 50, 159–177 CrossRef CAS.
- K. Barbeau, E. L. Rue, C. G. Trick, K. W. Bruland and A. Butler, Photochemical reactivity of siderophores produced by marine heterotrophic bacteria and cyanobacteria based on characteristic Fe(III) binding groups, Limnol. Oceanogr., 2003, 48, 1069–1078 CrossRef CAS.
- F. L. L. Muller, Exploring the potential role of terrestrially derived humic substances in the marine biogeochemistry of iron, Front. Earth Sci. China, 2018, 6, 159 CrossRef.
- K. S. Johnson, R. M. Gordon and K. H. Coale, What controls dissolved iron concentrations in the world ocean?, Mar. Chem., 1997, 57, 137–161 CrossRef CAS.
- J. N. Fitzsimmons and E. A. Boyle, Both soluble and colloidal iron phases control dissolved iron variability in the tropical North Atlantic Ocean, Geochim. Cosmochim. Acta, 2014, 125, 539–550 CrossRef CAS.
- M. L. Wells and E. D. Goldberg, Marine submicron particles, Mar. Chem., 1992, 40, 5–18 CrossRef CAS.
- B. P. von der Heyden and A. N. Roychoudhury, A review of colloidal iron partitioning and distribution in the open ocean, Mar. Chem., 2015, 177, 9–19 CrossRef CAS.
- B. A. Bergquist, J. Wu and E. A. Boyle, Variability in oceanic dissolved iron is dominated by the colloidal fraction, Geochim. Cosmochim. Acta, 2007, 71, 2960–2974 CrossRef CAS.
- J. Wu, E. Boyle, W. Sunda and L.-S. Wen, Soluble and colloidal iron in the oligotrophic North Atlantic and North Pacific, Science, 2001, 293, 847–849 CrossRef CAS PubMed.
- J. N. Fitzsimmons, G. G. Carrasco, J. Wu, S. Roshan, M. Hatta, C. I. Measures, T. M. Conway, S. G. John and E. A. Boyle, Partitioning of dissolved iron and iron isotopes into soluble and colloidal phases along the GA03 GEOTRACES North Atlantic Transect, Deep Sea Res., Part II, 2015, 116, 130–151 CrossRef CAS.
- J. N. Fitzsimmons, R. M. Bundy, S. N. Al-Subiai, K. A. Barbeau and E. A. Boyle, The composition of dissolved iron in the dusty surface ocean: An exploration using size-fractionated iron-binding ligands, Mar. Chem., 2015, 173, 125–135 CrossRef CAS.
- G. Xing, S. Garg and T. D. Waite, Is superoxide-mediated Fe(III) reduction important in sunlit surface waters?, Environ. Sci. Technol., 2019, 53, 13179–13190 CrossRef CAS PubMed.
- M. Gledhill and K. Buck, The organic complexation of iron in the marine environment: A review, Front. Microbiol., 2012, 3, 69 Search PubMed.
- R. M. Bundy, H. A. N. Abdulla, P. G. Hatcher, D. V. Biller, K. N. Buck and K. A. Barbeau, Iron-binding ligands and humic substances in the San Francisco Bay estuary and estuarine-influenced shelf regions of coastal California, Mar. Chem., 2015, 173, 183–194 CrossRef CAS.
- C. G. Trick, Hydroxamate-siderophore production and utilization by marine eubacteria, Curr. Microbiol., 1989, 18, 375–378 CrossRef CAS.
- S. W. Wilhelm and C. G. Trick, Iron-limited growth of cyanobacteria: Multiple siderophore production is a common response, Limnol. Oceanogr., 1994, 39, 1979–1984 CrossRef CAS.
- S. A. Amin, D. H. Green, F. C. Küpper and C. J. Carrano, Vibrioferrin, an unusual marine siderophore: iron binding, photochemistry, and biological implications, Inorg. Chem., 2009, 48, 11451–11458 CrossRef CAS.
- J. Granger and N. M. Price, The importance of siderophores in iron nutrition of heterotrophic marine bacteria, Limnol. Oceanogr., 1999, 44, 541–555 CrossRef CAS.
- R. C. Hider and X. Kong, Chemistry and biology of siderophores, Nat. Prod. Rep., 2010, 27, 637–657 RSC.
- J. B. Neilands, Siderophores: structure and function of microbial iron transport compounds, J. Biol. Chem., 1995, 270, 26723–26726 CrossRef CAS PubMed.
- K. Barbeau, G. Zhang, D. H. Live and A. Butler, Petrobactin, a photoreactive siderophore produced by the oil-degrading marine bacterium Marinobacter hydrocarbonoclasticus, J. Am. Chem. Soc., 2002, 124, 378–379 CrossRef CAS PubMed.
- A. Butler and R. M. Theisen, Iron(III)–siderophore coordination chemistry: Reactivity of marine siderophores, Coord. Chem. Rev., 2010, 254, 288–296 CrossRef CAS PubMed.
- Y. P. Lee, M. Fujii, T. Kikuchi, K. Terao and C. Yoshimura, Variation of iron redox kinetics and its relation with molecular composition of standard humic substances at circumneutral pH, PLoS One, 2017, 12, e0176484 CrossRef PubMed.
- A. L. Rose and T. D. Waite, Effect of dissolved natural organic matter on the kinetics of ferrous iron oxygenation in seawater, Environ. Sci. Technol., 2003, 37, 4877–4886 CrossRef CAS PubMed.
- J. M. Santana-Casiano, M. González-Dávila, M. J. Rodríguez and F. J. Millero, The effect of organic compounds in the oxidation kinetics of Fe(II), Mar. Chem., 2000, 70, 211–222 CrossRef CAS.
- E. G. Roy and M. L. Wells, Evidence for regulation of Fe(II) oxidation by organic complexing ligands in the Eastern Subarctic Pacific, Mar. Chem., 2011, 127, 115–122 CrossRef CAS.
- M. J. Hopwood, P. J. Statham, S. A. Skrabal and J. D. Willey, Dissolved iron(II) ligands in river and estuarine water, Mar. Chem., 2015, 173, 173–182 CrossRef CAS.
- E. E. Daugherty, B. Gilbert, P. S. Nico and T. Borch, Complexation and redox buffering of iron(II) by dissolved organic matter, Environ. Sci. Technol., 2017, 51, 11096–11104 CrossRef CAS PubMed.
- K. S. Johnson, K. H. Coale, V. A. Elrod and N. W. Tindale, Iron photochemistry in seawater from the equatorial Pacific, Mar. Chem., 1994, 46, 319–334 CrossRef CAS.
- M. A. Anderson and F. M. M. Morel, The influence of aqueous iron chemistry on the uptake of iron by the coastal diatom Thalassiosira weissflogii, Limnol. Oceanogr., 1982, 27, 789–813 CrossRef CAS.
- M. L. Wells and L. M. Mayer, The photoconversion of colloidal iron oxyhydroxides in seawater, Deep-Sea Res., Part A, 1991, 38, 1379–1395 CrossRef CAS.
- A. L. Rose and T. D. Waite, Reduction of organically complexed ferrici ron by superoxide in a simulated natural water, Environ. Sci. Technol., 2005, 39, 2645–2650 CrossRef CAS PubMed.
- C. J. Miller, S. M. Vincent Lee, A. L. Rose and T. D. Waite, Impact of natural organic matter on H2O2-mediated oxidation of Fe(II) in coastal seawaters, Environ. Sci. Technol., 2012, 46, 11078–11085 CrossRef CAS PubMed.
- M. González-Davila, J.
M. Santana-Casiano and F. J. Millero, Oxidation of iron (II) nanomolar with H2O2 in seawater, Geochim. Cosmochim. Acta, 2005, 69, 83–93 CrossRef.
- J. L. Pierre, M. Fontecave and R. R. Crichton, Chemistry for an essential biological process: the reduction of ferric iron, BioMetals, 2002, 15, 341–346 CrossRef CAS PubMed.
- M. J. A. Rijkenberg, A. C. Fischer, J. J. Kroon, L. J. A. Gerringa, K. R. Timmermans, H. T. Wolterbeek and H. J. W. de Baar, The influence of UV irradiation on the photoreduction of iron in the Southern Ocean, Mar. Chem., 2005, 93, 119–129 CrossRef CAS.
- M. J. A. Rijkenberg, L. J. A. Gerringa, P. J. Neale, K. R. Timmermans, A. G. J. Buma and H. J. W. de Baar, UVA variability overrules UVB ozone depletion effects on the photoreduction of iron in the Southern Ocean, Geophys. Res. Lett., 2004, 31, L24310 CrossRef.
- A. L. Rose and T. D. Waite, Predicting iron speciation in coastal waters from the kinetics of sunlight-mediated iron redox cycling, Aquat. Sci., 2003, 65, 375–383 CrossRef CAS.
- K. Kuma, S. Nakabayashi, Y. Suzuki, I. Kudo and K. Matsunaga, Photo-reduction of Fe(III) by dissolved organic substances and existence of Fe(II) in seawater during spring blooms, Mar. Chem., 1992, 37, 15–27 CrossRef CAS.
- O. Sivan, Y. Erel, D. Mandler and A. Nishri, The dynamic redox chemistry of iron in the epilimnion of Lake Kinneret (Sea of Galilee), Geochim. Cosmochim. Acta, 1998, 62, 565–576 CrossRef CAS.
- L. Emmenegger, D. W. King, L. Sigg and B. Sulzberger, Oxidation kinetics of Fe(II) in a eutrophic swiss lake, Environ. Sci. Technol., 1998, 32, 2990–2996 CrossRef CAS.
- P. C. Singer and W. Stumm, Acidic mine drainage: the rate-determining step, Science, 1970, 167, 1121–1123 CrossRef CAS PubMed.
- C. H. Gammons, D. A. Nimick, S. R. Parker, T. E. Cleasby and R. B. McCleskey, Diel behavior of iron and other heavy metals in a mountain stream with acidic to neutral pH: Fisher Creek, Montana, USA, Geochim. Cosmochim. Acta, 2005, 69, 2505–2516 CrossRef CAS.
- M. Diez Ercilla, E. López Pamo and J. Sánchez España, Photoreduction of Fe(III) in the acidic mine pit lake of San Telmo (Iberian Pyrite Belt): field and experimental work, Aquat. Geochem., 2009, 15, 391–419 CrossRef CAS.
- B. Sulzberger, J. L. Schnoor, R. Giovanoli, J. G. Hering and J. Zobrist, Biogeochemistry of iron in an acidic lake, Aquat. Sci., 1990, 52, 56–74 CrossRef.
- D. M. McKnight and S. M. Duren, Biogeochemical processes controlling midday ferrous iron maxima in stream waters affected by acid rock drainage, Appl. Geochem., 2004, 19, 1075–1084 CrossRef CAS.
- C. M. Tate, R. E. Broshears and D. M. McKnight, Phosphate dynamics in an acidic mountain stream: Interactions involving algal uptake, sorption by iron oxide, and photoreduction, Limnol. Oceanogr., 1995, 40, 938–946 CrossRef CAS.
- C. H. Gammons, S. A. Wood and D. A. Nimick, Diel behavior of rare earth elements in a mountain stream with acidic to neutral pH, Geochim. Cosmochim. Acta, 2005, 69, 3747–3758 CrossRef CAS.
- D. M. McKnight, B. A. Kimball and K. E. Bencala, Iron photoreduction and oxidation in an acidic mountain stream, Science, 1988, 240, 637–640 CrossRef CAS PubMed.
- B. A. Kimball, D. M. McKnight, G. A. Wetherbee and R. A. Harnish, Mechanisms of iron photoreduction in a metal-rich, acidic stream (St. Kevin Gulch, Colorado, U.S.A.), Chem. Geol., 1992, 96, 227–239 CrossRef CAS.
- A. B. Sullivan, J. I. Drever and D. M. McKnight, Diel variation in element concentrations, Peru Creek, Summit County, Colorado, J. Geochem. Explor., 1998, 64, 141–145 CrossRef CAS.
- C. H. Gammons, D. A. Nimick, S. R. Parker, D. M. Snyder, R. B. McCleskey, R. Amils and S. R. Poulson, Photoreduction fuels biogeochemical cycling of iron in Spain's acid rivers, Chem. Geol., 2008, 252, 202–213 CrossRef CAS.
- D. C. Fernández-Remolar, R. V. Morris, J. E. Gruener, R. Amils and A. H. Knoll, The Río Tinto Basin, Spain: Mineralogy, sedimentary geobiology, and implications for interpretation of outcrop rocks at Meridiani Planum, Mars, Earth Planet. Sci. Lett., 2005, 240, 149–167 CrossRef.
-
U. Lueder, B. B. Jørgensen, A. Kappler and C. Schmidt, Fe(III) photoreduction producing Fe2+aq in oxic freshwater sediment, submitted, 2019.
-
U. Lueder, M. Maisch, K. Laufer, B. B. Jørgensen, A. Kappler and C. Schmidt, Influence of physical perturbation on Fe(II) supply in coastal marine sediments, submitted, 2019.
- E. D. Melton, P. Stief, S. Behrens, A. Kappler and C. Schmidt, High spatial resolution of distribution and interconnections between Fe- and N-redox processes in profundal lake sediments, Environ. Microbiol., 2014, 16, 3287–3303 CrossRef CAS PubMed.
- K. Laufer, M. Nordhoff, H. Røy, C. Schmidt, S. Behrens, B. B. Jørgensen and A. Kappler, Coexistence of microaerophilic, nitrate-reducing, and phototrophic Fe(II) oxidizers and Fe(III) reducers in coastal marine sediment, Appl. Environ. Microbiol., 2016, 82, 1433–1447 CrossRef PubMed.
- F. Schaedler, C. Lockwood, U. Lueder, C. Glombitza, A. Kappler and C. Schmidt, Microbially mediated coupling of Fe and N cycles by nitrate-reducing Fe(II)-oxidizing bacteria in littoral freshwater sediments, Appl. Environ. Microbiol., 2018, 84, e02013–02017 Search PubMed.
- P. L. Croot, A. R. Bowie, R. D. Frew, M. T. Maldonado, J. A. Hall, K. A. Safi, J. La Roche, P. W. Boyd and C. S. Law, Retention of dissolved iron and Fe(II) in an iron induced Southern Ocean phytoplankton bloom, Geophys. Res. Lett., 2001, 28, 3425–3428 CrossRef CAS.
- C. Peng, C. Bryce, A. Sundman, T. Borch and A. Kappler, Organic matter complexation promotes Fe(II) oxidation by the photoautotrophic Fe(II)-oxidizer Rhodopseudomonas palustris TIE-1, ACS Earth Space Chem., 2019, 3, 531–536 CrossRef CAS.
- C. Peng, C. Bryce, A. Sundman and A. Kappler, Cryptic cycling of complexes containing Fe(III) and organic matter by phototrophic Fe(II)-oxidizing bacteria, Appl. Environ. Microbiol., 2019, 85, e02826–02818 CAS.
- C. Peng, A. Sundman, C. Bryce, C. Catrouillet, T. Borch and A. Kappler, Oxidation of Fe(II)–organic matter complexes in the presence of the mixotrophic nitrate-reducing Fe(II)-oxidizing bacterium Acidovorax sp. BoFeN1, Environ. Sci. Technol., 2018, 52, 5753–5763 CrossRef CAS PubMed.
- J. M. Otte, J. Harter, K. Laufer, N. Blackwell, D. Straub, A. Kappler and S. Kleindienst, The distribution of active iron-cycling bacteria in marine and freshwater sediments is decoupled from geochemical gradients, Environ. Microbiol., 2018, 20, 2483–2499 CrossRef CAS PubMed.
- X. A. Walter, A. Picazo, M. R. Miracle, E. Vicente, A. Camacho, M. Aragno and J. Zopfi, Phototrophic Fe(II)-oxidation in the chemocline of a ferruginous meromictic lake, Front. Microbiol., 2014, 5, 713 Search PubMed.
- M. Llirós, T. García-Armisen, F. Darchambeau, C. Morana, X. Triadó-Margarit, Ö. Inceoğlu, C. M. Borrego, S. Bouillon, P. Servais, A. V. Borges, J. P. Descy, D. E. Canfield and S. A. Crowe, Pelagic photoferrotrophy and iron cycling in a modern ferruginous basin, Sci. Rep., 2015, 5, 13803 CrossRef PubMed.
- S. W. Poulton and D. E. Canfield, Ferruginous conditions: A dominant feature of the ocean through Earth's history, Elements, 2011, 7, 107–112 CrossRef CAS.
- D. E. Canfield, M. T. Rosing and C. Bjerrum, Early anaerobic metabolisms, Philos. Trans. R. Soc., B, 2006, 361, 1819–1836 CrossRef CAS PubMed.
- A. Camacho, X. A. Walter, A. Picazo and J. Zopfi, Photoferrotrophy: Remains of an ancient photosynthesis in modern environments, Front. Microbiol., 2017, 8, 323 Search PubMed.
- F. J. Millero, S. Sotolongo and M. Izaguirre, The oxidation kinetics of Fe(II) in seawater, Geochim. Cosmochim. Acta, 1987, 51, 793–801 CrossRef CAS.
- K. J. Edwards, K. Becker and F. Colwell, The deep, dark energy biosphere: Intraterrestrial life on earth, Annu. Rev. Earth Planet. Sci., 2012, 40, 551–568 CrossRef CAS.
- C. L. Van Dover, G. T. Reynolds, A. D. Chave and J. A. Tyson, Light at deep-sea hydrothermal vents, Geophys. Res. Lett., 1996, 23, 2049–2052 CrossRef.
- S. N. White, A. D. Chave and G. T. Reynolds, Investigations of ambient light emission at deep-sea hydrothermal vents, J. Geophys. Res.: Solid Earth, 2002, 107, EPM 1-1–EPM 1-13 CrossRef.
- J. T. Beatty, J. Overmann, M. T. Lince, A. K. Manske, A. S. Lang, R. E. Blankenship, C. L. Van Dover, T. A. Martinson and F. G. Plumley, An obligately photosynthetic bacterial anaerobe from a deep-sea hydrothermal vent, Proc. Natl. Acad. Sci. U. S. A., 2005, 102, 9306–9310 CrossRef CAS PubMed.
- H. Gao and R. G. Zepp, Factors influencing photoreactions of dissolved organic matter in a coastal river of the Southeastern United States, Environ. Sci. Technol., 1998, 32, 2940–2946 CrossRef CAS.
- S. E. Page, M. Sander, W. A. Arnold and K. McNeill, Hydroxyl radical formation upon oxidation of reduced humic acids by oxygen in the dark, Environ. Sci. Technol., 2012, 46, 1590–1597 CrossRef CAS PubMed.
- M. Minella, E. De Laurentiis, V. Maurino, C. Minero and D. Vione, Dark production of hydroxyl radicals by aeration of anoxic lake water, Sci. Total Environ., 2015, 527–528, 322–327 CrossRef CAS PubMed.
- S. A. Murphy, B. M. Solomon, S. Meng, J. M. Copeland, T. J. Shaw and J. L. Ferry, Geochemical production of reactive oxygen species from biogeochemically reduced Fe, Environ. Sci. Technol., 2014, 48, 3815–3821 CrossRef CAS PubMed.
- S. A. Murphy, S. Meng, B. M. Solomon, D. M. C. Dias, T. J. Shaw and J. L. Ferry, Hydrous ferric oxides in sediment catalyze formation of reactive oxygen species during sulfide oxidation, Frontiers in Marine Science, 2016, 3, 227 CrossRef.
- J. N. Grossman and T. F. Kahan, Hydroxyl radical formation from bacteria-assisted Fenton chemistry at neutral pH under environmentally relevant conditions, Environ. Chem., 2016, 13, 757–766 CrossRef CAS.
- S. E. Page, G. W. Kling, M. Sander, K. H. Harrold, J. R. Logan, K. McNeill and R. M. Cory, Dark formation of hydroxyl radical in arctic soil and surface waters, Environ. Sci. Technol., 2013, 47, 12860–12867 CrossRef CAS PubMed.
- A. Trusiak, L. A. Treibergs, G. W. Kling and R. M. Cory, The role of iron and reactive oxygen species in the production of CO2 in arctic soil waters, Geochim. Cosmochim. Acta, 2018, 224, 80–95 CrossRef CAS.
- J. M. Diaz, C. M. Hansel, B. M. Voelker, C. M. Mendes, P. F. Andeer and T. Zhang, Widespread production of extracellular superoxide by heterotrophic bacteria, Science, 2013, 340, 1223–1226 CrossRef CAS PubMed.
- K. M. Sutherland, A. Coe, R. J. Gast, S. Plummer, C. P. Suffridge, J. M. Diaz, J. S. Bowman, S. D. Wankel and C. M. Hansel, Extracellular superoxide production by key microbes in the global ocean, Limnol. Oceanogr., 2019, 64, 2679–2693 CrossRef CAS.
- T. Zhang, C. M. Hansel, B. M. Voelker and C. H. Lamborg, Extensive
dark biological production of reactive oxygen species in brackish and freshwater ponds, Environ. Sci. Technol., 2016, 50, 2983–2993 CrossRef CAS PubMed.
- T. D. Waite, R. Szymczak, Q. I. Espey and M. J. Furnas, Diel variations in iron speciation in northern Australian shelf waters, Mar. Chem., 1995, 50, 79–91 CrossRef CAS.
- P. L. Croot, P. Laan, J. Nishioka, V. Strass, B. Cisewski, M. Boye, K. R. Timmermans, R. G. Bellerby, L. Goldson, P. Nightingale and H. J. W. de Baar, Spatial and temporal distribution of Fe(II) and H2O2 during EisenEx, an open ocean mescoscale iron enrichment, Mar. Chem., 2005, 95, 65–88 CrossRef CAS.
- C. M. Hansel, T. G. Ferdelman and B. M. Tebo, Cryptic cross-linkages among biogeochemical cycles: novel insights from reactive intermediates, Elements, 2015, 11, 409–414 CrossRef CAS.
- R. J. Schneider, K. L. Roe, C. M. Hansel and B. M. Voelker, Species-level variability in extracellular production rates of reactive oxygen species by diatoms, Front. Chem., 2016, 4, 5 Search PubMed.
|
This journal is © The Royal Society of Chemistry 2020 |
Click here to see how this site uses Cookies. View our privacy policy here.