DOI:
10.1039/C9FO01149H
(Paper)
Food Funct., 2020,
11, 907-920
Characterising absorption and health-related properties of phytochemicals extracted from Malaysian palm fruit biomass after oil extraction†
Received
30th May 2019
, Accepted 29th December 2019
First published on 31st December 2019
Abstract
After oil extraction, palm fruit biomass contains abundant water-soluble phytochemicals (PCs) with proven bioactivity in regulating oxidative stress and inflammation (OSI). For optimal bioefficacy following oral consumption, the pharmacokinetic plasma peak (Tmax) should be bio-matched with the onset of OSI, which can be predicted from the Phytochemical Absorption Prediction (PCAP) model and methodology. Predicted absorption and potential for regulation of OSI by measures of total phenolic content, antioxidant capacity and hydrogen peroxide production capacity, were applied to characterise eight extracts from mesocarp fibre and kernel shells of oil-depleted palm fruits. Results indicated post-consumption absorption Tmax ranges of 0.5–12 h and 2–6 h for intake in liquid and solid forms, respectively, and generally high antioxidant activity of the extracts. The research supports that PC extracts of palm fruit biomass have broad potential uses for human health as dietary antioxidants in foods, supplements or functional beverages.
Introduction
The production of palm oil (Elaeis guineensis Jacq.) generates large volumes of biomass co-product that can cause environmental stress.1 One hectare of oil palm plantation generates ∼21.63 tonnes per year of ‘waste’ biomass including leaves, mesocarp fibres, kernel shells of palm fruits and a process stream of aqueous liquor which is mostly un-utilised.2,3 Water-soluble phytochemicals extracted from oil palm biomass are rich in polyphenolics and exert a range of useful bioactivities in vitro and in vivo according to animal-based studies, including: antioxidant,4 anti-inflammatory,5 anti-tumour,6 anti-diabetic7 and protection against cardiovascular disease.8 These indications support the opportunity to transform these heavy bio-burden materials into higher value bio-products with broad uses for human health as foods, supplements, nutraceutical and pharmaceutical products.
Palm biomass extracts are rich sources of polyphenolic compounds including phenolic acids, flavonoids and tannins.6,9 Supplementation of a palm fruit extract either as a drink or mixed into the diet for 4–36 weeks reduced diabetes symptoms such as hyperglycaemia and lipaemia, delayed the onset of diabetes and even reversed advancing diabetes in a carbohydrate-induced type 2 diabetes rat model.7,10 The protection provided by the extract was correlated to its polyphenolic content.7 Further, extracts from palm fruits and palm leaves were observe to protect against atherosclerosis in rats, mice and rabbits.5,8,11 The anti-diabetic and anti-atherogenic effects of palm biomass extracts have been linked to their capacity to regulate oxidative stress and inflammation. In a diabetes-induced rat model, a palm leaf extract protected against renal damage by attenuating oxidative stress in the kidney via improvement of antioxidant defence as indicated by increased levels of the cellular antioxidant molecule glutathione.12 Furthermore, in an atherosclerosis-induced mouse model, palm fruit extract reduced concentrations of the pro-inflammatory cytokine interleukin-12 (IL-12) while increasing serum concentrations of the anti-inflammatory cytokine IL-13.5 Further, supplementation of the palm fruit extract resulted in upregulation of the antioxidant genes in the mouse heart including Mgst1 (microsomal glutathione S-transferase) and Gpx1 (glutathione peroxidase 1), important in cellular antioxidant defence.5 The anti-inflammatory activity of polyphenols has been linked to their ability to inactivate the pro-inflammatory NF-κB (nuclear factor kappa-light-chain-enhancer of activated B cells) pathway, resulting in the inhibition of expression of various pro-inflammatory cytokines and chemokines.13 Further, the antioxidant effects of polyphenols could be attributed to their ability to activate Nrf2 (nuclear factor-erythoid-2-related factor 2 (Nrf2), a transcription factor involved in inducing the production of antioxidant enzymes.14
The cellular antioxidant defence system is comprised of non-enzymatic, enzymatic defence and DNA repair systems.15 The non-enzyme antioxidant defence is made up of antioxidant molecules that directly scavenge reactive oxygen species (ROS), and metal-chelators that prevent ROS formation by controlling the level of pro-oxidative free metal ions. Molecules of the non-enzymatic antioxidant defences include vitamin C, vitamin E, uric acid, glutathione, thioredoxin, transferrin, metallothionein and coeruloplasmin.15 The enzymatic antioxidant defence systems remove ROS via enzymatic reactions. Important defence enzymes include superoxide dismutase (SOD), catalase, glutathione reductase, glutathione transferase and glutathione peroxidase.15
The mechanism by which palm phytochemicals improve the cellular antioxidant defence system could be related to their ability to generate stress signals, in particular hydrogen peroxide (H2O2).16 Phytochemicals have been reported to produce H2O2 in cell culture media,17 and in plasma in vitro, with yields of H2O2 production highly correlated with the antioxidant response in plasma of pigs fed with the phytochemicals.18 The observed production of H2O2 by phytochemicals in vitro could result from the electron transfer reactions between phytochemicals, oxygen, ascorbic acid and metal ions such as copper and irons present in cell culture media19,20 and plasma.18,21
Therefore, apart from the well-established direct antioxidant capacity, the ability of phytochemicals to produce H2O2 could be an additional indicator of the indirect antioxidant capacity of phytochemicals via stimulating the cellular antioxidant defence system.
The protective effects of phytochemicals are affected by their bioavailability.22 After consumption, a fraction of the low molecular mass ingested phytochemicals is absorbed into circulation via the small intestine and subsequently, some components are metabolised by the liver, releasing the hepatic metabolites into circulation.23 The fractions that are not absorbed in the small intestine, excluded due to the larger mass and size, travel to the large intestine and are subjected to substantial microbial transformation, releasing the microbial metabolites into circulation.22 Therefore, over time, plasma contains the native phytochemicals and their hepatic and microbial metabolites with each component having a time required to reach maximal plasma concentration (Tmax) from the time of ingestion.22 However, the PCAP model is concerned with and predicts the kinetics only of the passively absorbing fraction of phytochemicals.
T
max represents an important indicator of the in vivo efficacy of PCs to inform optimal ‘bio-matching’ to need.24 Depending on chemical structures, Tmax of phytochemicals can range from <1 h to 48 h.25,26 Understanding Tmax of phytochemicals informs the clinical dosing frequency for optimising health outcomes.27
Recently, an in silico phytochemical absorption prediction (PCAP) model was reported, allowing direct calculation of the in vivo Tmax of the absorbing phytochemical fractions into circulation in their native forms, based on molecular mass and lipophilicity descriptor log
P.24 Further, a liquid chromatography mass spectrometry (LC-MS) method was also developed to characterise phytochemicals for application of the PCAP model to complex, unidentified mixtures.28 This allows high-throughput predictions of the Tmax profiles ‘functional fingerprinting’ of phytochemical mixtures in plant extracts. The aim of this study is to apply these methods to characterise the absorption properties of phytochemicals in palm fruit biomass fractions associated with oil extraction processing, and to characterise their potential for in vivo regulation of oxidative stress.
Materials and methods
Materials
Mesocarp fibres (M) and kernel shells (K) of palm fruit biomass recovered after oil extraction were collected from pooled, representative samples taken on a single day of processing, from four palm mill plants of various regions in Malaysia including: East Mill (EM) and West Mill (WM) of Sime Darby Plantation (Petaling Jaya, Selangor, Malaysia), Jugra Mill (JM) and Sei Ulu Langat Mill (SM) of The Malaysian Palm Oil Board (Kajang, Selangor, Malaysia). The mesocarp fibres were used unmodified and kernel shells were ground to a powder using a Kinematica Polymix PX-MFC 90D hammer mill (Eschbach, Germany).
Chemicals and reagents including methanol, gallic acid, Folin–Ciocalteu reagent, sodium carbonate (Na2CO3), acetonitrile, formic acid, L-histidine, (S)-dihyroorotate, shikimate, 4-pyrodoxate, 3-hydroxybenzyl alcohol, 3-hydroxybenzaldehyde, trans-ciinamate, estradiol-17α, deoxycholate, retinoate, oleic acid, heptadecanoate, freeze-dried human plasma (4% trisodium citrate as anticoagulant), Trolox, tris(hydroxymethyl)aminomethane (Tris), glycine, trisodium citrate, urea, hydrochloric acid (HCl), bathocuprionedisulfonic acid disodium salt hydrate (BCS), copper(II) chloride (CuCl2), hydrogen peroxide (H2O2), sulfuric acid (H2SO4), xylenol orange, ammonium iron(II) sulfate hexahydrate [(NH4)2Fe(SO4)2], and butylated hydroxytoluene (BHT) were from Sigma-Aldrich (St Louis, MO, USA). 96 well plates were Costar 3599 (Corning, New York, USA).
Tris-glycine-urea buffer pH 7 contained 0.086 M Tris, 0.09 M glycine, 4 mM trisodium citrate, and 8 M urea, adjusted to pH 7 using 2 M HCl. Ferrous ion oxidation-xylenol orange (FOX) reagent contained 25 mM H2SO4, 0.1 mM xylenol orange, 0.25 mM (NH4)2Fe(SO4)2, and 4 mM BHT in 90% methanol.
Extraction of water-soluble phytochemicals from palm fruit biomass extracts
Extraction of water-soluble phytochemicals from palm fruit biomass was using methodology that was potentially food process and product compatible, as previously described.29 Briefly, mesocarp fibre and kernel shell powder were mixed with Milli Q water (1
:
5 and 1
:
3 ratio w/v, respectively) before autoclaving at 121 °C for 20 min (Hiclave HVE-50, Hirayama, Tokyo, Japan). After cooling to room temperature, the mixture was filtered through 1 μm pore size bag filter (Sefar Filtration Inc., Huntingwood, NSW, Australia). The filtrate was dried in a convection oven (UF 110, Memmert GmbH & Co. KG, Waldbronn, Germany) at 80 °C until reaching a water activity of ∼0.4 at 25 °C. The dried extract (‘whole’ extract) was reconstituted in Milli Q water at a concentration of 10 mg mL−1 and sonicated for 30 min before centrifugation at 5000g for 1.5 h at 22 °C in centrifugal filtration devices (Amicon Ultra-15, 3k MWCO, Merck Millipore, Carrigtwohill, County Cork, Ireland) to collect the fraction with molecular mass <3 kDa, before freeze-drying. All samples were stored at −20 °C prior to analysis.
Proximate composition analysis of palm fruit biomass extracts
Proximate composition of palm fruit biomass extracts were determined as described previously.28 Total nitrogen was analysed at the Water Studies Centre (Monash University, Clayton, Victoria, Australia) by an ANCA GSL2 elemental analyser interfaced to a Hydra 20-22 continuous-flow isotope ratio mass-spectrometer (Sercon Ltd, UK). Crude protein content was calculated from total nitrogen using the nitrogen-to-protein conversion factor of 6.25.30 Mineral analysis was determined by inductively coupled plasma optical emission spectroscopy (ICP-OES) after microwave digestion with nitric acid. Total ash content was estimated as the sum of all minerals in the extract. Water activity was measured using a Aqualab water activity meter (model 3 TE, Pullman, WA, USA) at 25 °C. All analyses were performed in duplicate.
Total phenolic content of palm fruit biomass extracts
Total phenolic content of palm fruit biomass extracts was quantified using a modified Folin–Ciocalteu spectrophotometric methodology31 as described previously28 and expressed as gallic acid equivalent (GAE) using a gallic acid standard calibration curve over the range of 0–500 μg mL−1 in 20% methanol.
Prediction of human absorption kinetics of palm fruit biomass extracts
Prediction of human absorption kinetics i.e., ‘functional fingerprints’ of palm fruit biomass extracts was performed using the reported liquid chromatography mass spectrometry (LC-MS) analysis and the statistical model method,28 with some modifications. In brief, palm fruit biomass extract was reconstituted in 20% methanol at a concentration of 20 mg mL−1 and 1 μL was injected into an Agilent LC-MS system comprised of Agilent 1200 series high performance LC system and Agilent 6520 quadruple time-of-flight (QTOF) MS system (Santa Clara, CA, USA). Chromatography was carried out on an Agilent InfinityLab Poroshell 120 EC-C18, 2.1 × 100 mm, 2.7 μm column with an Agilent Poroshell 120 EC-C18, 2.1 mm guard column at a flow rate of 400 μL min−1 for 20 min at 40 °C. Mobile phases included (A) 0.1% formic acid in deionised water and (B) 0.1% formic acid in acetonitrile. A gradient LC method was conducted as follows, 5–30% mobile phase B for 5 min, followed by 30–100% mobile phase B for 5 min and then a 5 min hold, followed by a 5 min re-equilibration at 5% mobile phase B. The MS was conducted in positive or negative mode with the following conditions (positive/negative, respectively): nebuliser pressure 30/45 psi, gas flow-rate 10 L min−1, gas temperature 300 °C, capillary voltage 4000/−3500 V, fragmentor 150 and skimmer 65 V. Molecular feature extraction (MFE) was operated using Agilent MassHunter Qualitative analysis (version B.07.00) and MassHunter Profinder (version B.06.00) and was restricted to the 1000 largest features and 1–2 charge states. Allowed ion species: +H, +Na, +K, +NH4, (positive mode); −H, +Cl, +HCOO (negative mode) and neutral losses: H2O, H3PO4, CO2, C6H12O6.
Retention time obtained from the LC-MS analysis was converted to lipophilicity descriptor log
P using a calibration curve prepared from 11 standards: L-histidine, (S)-dihyroorotate, shikimate, 4-pyrodoxate, 3-hydroxybenzyl alcohol, 3-hydroxybenzaldehyde, trans-cinamate, estradiol-17α, deoxycholate, retinoate, oleic acid and heptadecanoate.
Molecular features with a molecular mass range of 122–1270 Da was selected and their predicted Tmax was calculated from log
P and molecular mass using the PCAP models, with different formulas applied for intakes as liquid (i.e., drink) and solid (i.e., food) forms.24 Molecular features within the extract were sorted into ‘primary metabolites’ and ‘secondary metabolites’ as also described previously.28 The functional fingerprint of palm fruit biomass extract was generated by plotting the predicted Tmax and corresponding relative abundance of molecular features, within the extract.
In vitro total antioxidant and prooxidant capacity of palm fruit biomass extract
In vitro analysis of total antioxidant and prooxidant capacity of palm fruit biomass extract was measured in human plasma as previously described,18 with gallic acid as positive control because gallic acid has been found in its native form in plasma of both rats32 and humans33 after ingestion. Briefly, freeze-dried human plasma was reconstituted in Milli Q water according to supplier instruction, and aliquots were stored frozen until use. Gallic acid standard and palm fruit biomass extracts were added to thawed plasma to final concentrations of 0–0.5 mg GAE per mL, incubated at 37 °C for 1 h, diluted 1
:
5 with Tris-glycine-urea buffer before measurements of total antioxidant and prooxidant capacity. Analyses were performed in duplicate.
Total antioxidant capacity was analysed using the cupric reducing antioxidant capacity (CUPRAC) assay,34 and expressed as Trolox equivalent antioxidant capacity (TEAC). In a 96-well plate, equal volumes (50 μL) of 7.5 mM BCS, 10 mM CuCl2 and Tris-glycine-urea buffer were added to each well, followed by 100 μL of sample (blank, standard, or diluted plasma). After incubation at 22 °C for 1 h, absorbance was measured at 485 nm. Results were expressed as TEAC based on a calibration curve of Trolox standard with concentrations of 0–100 μM after blank subtraction. Plasma TEAC yields (nmol μmol−1 GAE) were the slope of linear regression of plasma TEAC as a function of GAE concentrations.
Prooxidant capacity was measured as hydrogen peroxide production using the FOX assay.35 10 μL of methanol was added to 90 μL of samples (blank, standard or diluted plasma), followed by 900 μL of FOX reagent and vortexed for 5 s. The mixture was incubated at 22 °C for 30 min prior to centrifugation at 15
000g for 30 s at 22 °C, followed by reading absorbance of the supernatant at 560 nm. Concentrations of plasma H2O2 were determined based on a calibration curve of H2O2 standard with concentrations of 0–90 μM after blank subtraction. Yields of H2O2 production in vitro (nmol μmol−1 GAE) were the slope of linear regression of H2O2 concentration as a function of GAE concentrations. Analyses were performed in duplicate.
Data analysis
Curve-fitting and analyses including two-tailed t-test, Pearson's correlation analyses were performed using SigmaPlot for Windows Version 13.0 (Systat Software Inc., Chicago, IL, USA).
Results
Composition of palm fruit biomass extracts
Extraction of water-soluble phytochemicals from palm fruit biomass using autoclave and filtration resulted in recoveries of 0.6–2.86% with merocarp fibres having higher recovery compared to kernel shells (Table 1). Fractionation yielded 44.85–65.83% of extracted solids with molecular weight less than 3 kDa. Total nitrogen of the extracts ranged from 0.99 to 2.13%, corresponding to crude protein of 6.19–13.34% (Table 1). Total ash of the extracts was 6.91–9.56% with potassium, magnesium, phosphorous, sulphur and calcium being the main elements (Tables 1 and 2). Total phenolic contents were 40.83–179.23 mg GAE per g for the whole extracts and 40.75–127.02 mg GAE per g for the <3 kDa fractions. Fractionation of the extracts did not result in significant enrichment or depletion in total phenolic contents, except for the WM-M, SM-M, and JM-K samples (Table 1).
Table 1 Recovery yields and composition of palm fruit biomass extracts. Data represent the mean of analysis in duplicate ± standard error
Sample type |
Sample ID |
Solids recovery of extracts from raw materials (%, w/w) |
Solids fractionation of extracts by size (%, w/w) |
Total nitrogena (%) |
Crude proteinb (%) |
Total ashc (%) |
Total phenolic content (mg GAE per g) |
<3 kDa |
>3 kDa |
Whole |
Whole |
Whole |
Whole |
<3 kDa |
Sum of organic plus inorganic nitrogen.
Based on total nitrogen × 6.25.
Calculated from sum of minerals analysis (Table 2).
Significantly different to the corresponding whole extract (p ≤ 0.05, two-tailed t-test).
|
Mesocarp |
EM-M |
2.02 |
52.69 |
44.86 |
1.78 ± 0.01 |
11.10 ± 0.06 |
8.48 ± 0.19 |
52.08 ± 3.54 |
53.81 ± 0.32 |
WM-M |
2.26 |
55.34 |
47.14 |
1.45 ± 0.02 |
9.03 ± 0.14 |
7.97 ± 0.14 |
60.03 ± 3.56 |
43.91d ± 0.32 |
JM-M |
2.22 |
50.18 |
43.11 |
2.13 ± 0.01 |
13.34 ± 0.07 |
8.77 ± 0.02 |
43.64 ± 1.71 |
40.75 ± 0.32 |
SM-M |
2.86 |
65.68 |
40.18 |
1.92 ± 0.00 |
11.98 ± 0.01 |
8.51 ± 0.25 |
40.83 ± 0.32 |
43.40d ± 0.32 |
Kernel |
EM-K |
1.64 |
65.83 |
38.76 |
1.36 ± 0.00 |
8.48 ± 0.00 |
8.46 ± 0.04 |
45.39 ± 2.92 |
47.96 ± 0.97 |
WM-K |
1.31 |
63.07 |
37.76 |
1.18 ± 0.01 |
7.36 ± 0.09 |
6.91 ± 0.16 |
86.31 ± 6.17 |
44.68 ± 9.41 |
JM-K |
0.82 |
44.85 |
40.08 |
0.99 ± 0.01 |
6.19 ± 0.05 |
9.56 ± 0.22 |
179.23 ± 9.42 |
122.01d ± 1.62 |
SM-K |
0.60 |
49.39 |
41.02 |
1.06 ± 0.00 |
6.64 ± 0.01 |
8.12 ± 0.05 |
122.11 ± 5.53 |
127.02 ± 4.86 |
Table 2 Mineral analysis of whole palm biomass extracts. Data represent the mean of analysis in duplicate ± standard error
Sample ID |
Ca (μg g−1) |
K (μg g−1) |
Mg (μg g−1) |
Na (μg g−1) |
S (μg g−1) |
Al (μg g−1) |
As (μg g−1) |
B (μg g−1) |
Cd (μg g−1) |
Co (μg g−1) |
Cr (μg g−1) |
Cu (μg g−1) |
Fe (μg g−1) |
Mn (μg g−1) |
Mo (μg g−1) |
Ni (μg g−1) |
P (μg g−1) |
Pb (μg g−1) |
Sb (μg g−1) |
Se (μg g−1) |
Ti (μg g−1) |
Zn (μg g−1) |
EM-M |
4875 ± 125 |
58 000 ± 1300 |
9415 ± 245 |
334.7 ± 4.5 |
4880 ± 130 |
628.3 ± 4.2 |
<5 |
56.2 ± 1.4 |
<5 |
<5 |
<5 |
17.9 ± 0.2 |
553.9 ± 0.3 |
195.6 ± 3.3 |
<5 |
<5 |
5795 ± 115 |
<5 |
<10 |
<5 |
<5 |
91.8 ± 2.8 |
WM-M |
4305 ± 5 |
53 400 ± 700 |
9375 ± 485 |
316.0 ± 8.7 |
4300 ± 40 |
411.8 ± 45.6 |
<5 |
52.1 ± 0.2 |
<5 |
<5 |
<5 |
11.3 ± 0.1 |
481.1 ± 28.9 |
203.9 ± 3.9 |
<5 |
<5 |
6705 ± 55 |
<5 |
<10 |
<5 |
<5 |
118.9 ± 2.0 |
JM-M |
4080 ± 20 |
59 950 ± 150 |
9730 ± 10 |
429.8 ± 1.8 |
5665 ± 45 |
361.3 ± 5.2 |
<5 |
49.6 ± 0.2 |
<5 |
<5 |
<5 |
32.2 ± 0.6 |
321.3 ± 1.2 |
116.3 ± 0.0 |
<5 |
≤5 |
6920 ± 10 |
<5 |
<10 |
<5 |
<5 |
65.0 ± 0.3 |
SM-M |
6210 ± 90 |
56 050 ± 750 |
10 500 ± 1500 |
379.9 ± 4.9 |
4885 ± 65 |
421.9 ± 9.6 |
<5 |
50.1 ± 0.6 |
<5 |
<5 |
<5 |
16.8 ± 0.1 |
285.2 ± 8.9 |
114.1 ± 0.1 |
<5 |
<5 |
6065 ± 35 |
<5 |
<10 |
<5 |
<5 |
80.0 ± 0.8 |
EM-K |
4675 ± 5 |
56 100 ± 100 |
9050 ± 230 |
367.7 ± 4.3 |
4115 ± 25 |
241.9 ± 20.2 |
<5 |
66.3 ± 0.6 |
<5 |
<5 |
<5 |
<5 |
886.9 ± 17.5 |
338.5 ± 3.0 |
<5 |
<5 |
8655 ± 35 |
<5 |
<10 |
<5 |
<5 |
92.4 ± 2.1 |
WM-K |
3245 ± 95 |
47 150 ± 1250 |
7245 ± 55 |
347.8 ± 9.1 |
3535 ± 65 |
407.8 ± 11.2 |
<5 |
59.3 ± 0.7 |
<5 |
<5 |
7.5 ± 0.2 |
6.5 ± 0.4 |
659.9 ± 5.9 |
239.1 ± 1.1 |
<5 |
6.9 ± 0.2 |
6070 ± 100 |
<5 |
<10 |
<5 |
<5 |
102.8 ± 3.2 |
JM-K |
2470 ± 50 |
76 850 ± 1950 |
5660 ± 20 |
1450.0 ± 30.0 |
3150 ± 70 |
419.0 ± 27.1 |
<5 |
101.0 ± 1.9 |
<5 |
<5 |
20.8 ± 1.1 |
13.1 ± 2.3 |
536.9 ± 24.7 |
136.6 ± 0.3 |
<5 |
16.0 ± 1.4 |
4775 ± 65 |
<5 |
<10 |
<5 |
<5 |
53.4 ± 0.5 |
SM-K |
3860 ± 10 |
59 350 ± 350 |
5860 ± 80 |
1015.0 ± 5.0 |
3125 ± 25 |
376.2 ± 13.8 |
<5 |
100.5 ± 0.5 |
<5 |
<5 |
21.5 ± 0.2 |
8.9 ± 0.3 |
448.3 ± 2.1 |
229.5 ± 0.1 |
<5 |
15.5 ± 0.1 |
6770 ± 30 |
<5 |
<10 |
<5 |
<5 |
89.2 ± 0.7 |
Predicted human absorption as ‘functional fingerprints’ of palm fruit biomass extracts
Predicted human absorption as ‘functional fingerprints’ of palm fruit biomass extracts was performed using a statistical model coupled with LC-MS analysis. Retention times of the molecular features obtained from the LC-MS analysis were converted into the hydrophobicity descriptor log
P using the calibration curve of eleven standards with R2 of 0.98 (Fig. 1A). Molecular features acquired from the LC-MS analysis were sorted into primary and secondary metabolites (herein referred to as phytochemicals). This sorting method was deemed valid based on a significant correlation (Pearson's correlation coefficient 0.69, p ≤ 0.05) between the total phenolic content and LC-MS relative quantification of secondary metabolites expressed as total peak area (Fig. 1B).
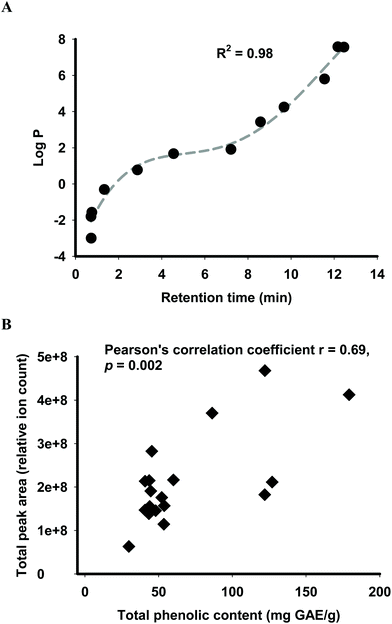 |
| Fig. 1 Translation of physicochemical data obtained from LC-MS analysis into predicted phytochemical absorption ‘functional fingerprints’ of palm biomass extracts. (A) Calibration of retention time of standards analysed by LC-MS for determination of log P of individual phytochemicals. (B) Relationship between total phenolic content and relative abundance of total secondary metabolites in palm biomass extracts, determined as LC-MS total peak area (relative ion count). | |
The functional fingerprints of sixteen extracts from palm fruit biomass samples were presented as peak area (relative ion count) versus their predicted Tmax for intakes as liquid (Fig. 2 and 4) or solid forms (Fig. 3 and 5). The primary metabolites of all extracts displayed Tmax ranges of 0.4–2.8 h (Fig. 2 and 4), and 1.8–3.3 h (Fig. 3 and 5) in drink form and solid form, respectively. In terms of phytochemical Tmax, whole extracts from both mesocarp fibres and kernel shells had similar Tmax ranges of 0.4–12 h (Fig. 2 and 4A–D), and 1.6–6.4 h (Fig. 3 and 5A–D) in drink form and solid form, respectively. The low molecular weight fractions of <3 kDa had shorter Tmax ranges compared to the whole extracts with Tmax of 0.4–6 h (Fig. 3 and 5E–H) and 1.6–5.5 h (Fig. 2 and 4E–H) for intakes as drink form and solid form, respectively.
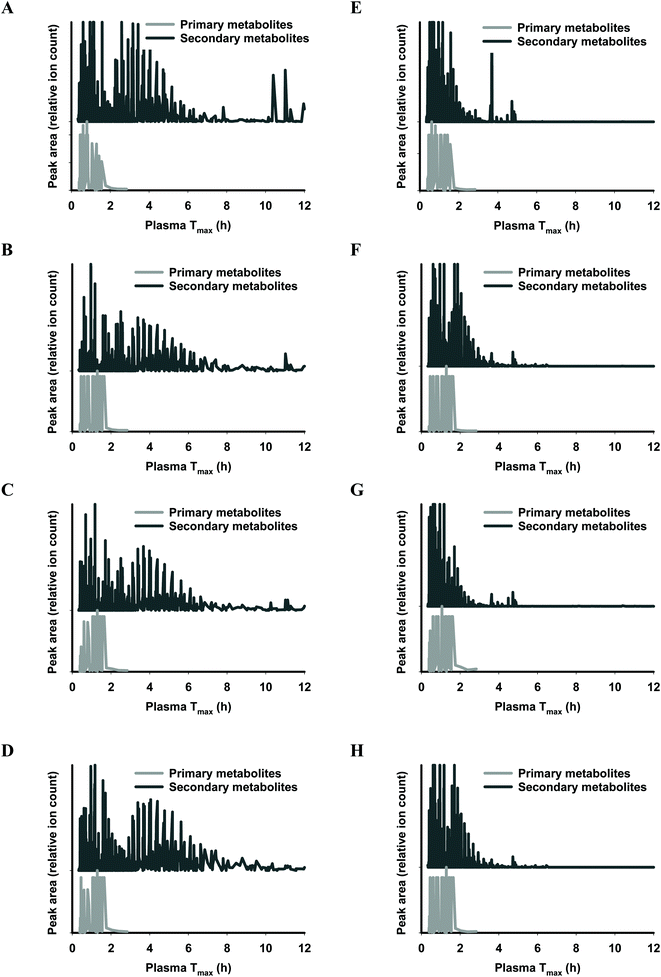 |
| Fig. 2 Predicted ‘functional fingerprint’ of palm mesocarp biomass extracted phytochemicals, for liquid (drink) intake. Molecular mass and log P obtained from LC-MS were used to calculate plasma Tmax (h) of individual primary and secondary metabolites for (A) EM-M whole, (B) WM-M whole, (C) JM-M whole, (D) SM-M whole; and for mass fractions <3 kDa: (E) EM-M <3 kDa, (F) WM-M <3 kDa, (G) JM-M <3 kDa, and (H) SM-M <3 kDa. | |
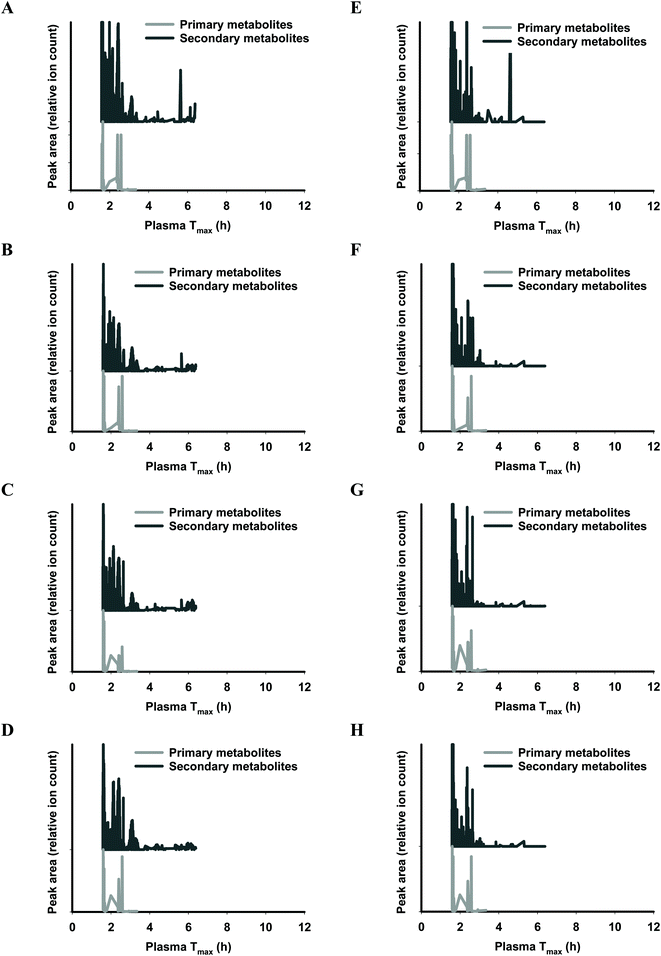 |
| Fig. 3 Predicted ‘functional fingerprint’ of palm mesocarp biomass extracts if consumed in solid form. Molecular mass and log P obtained from LC-MS were used to calculate plasma Tmax (h) of individual primary and secondary metabolites for (A) EM-M whole, (B) WM-M whole, (C) JM-M whole, (D) SM-M whole; and for mass fractions <3 kDa: (E) EM-M <3 kDa, (F) WM-M <3 kDa, (G) JM-M <3 kDa, and (H) SM-M <3 kDa. | |
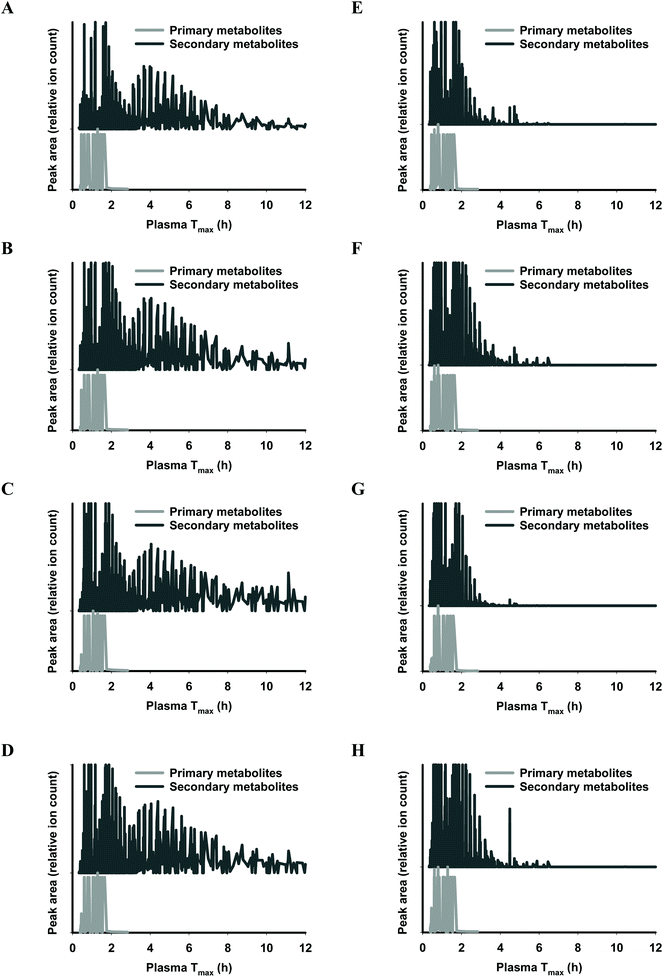 |
| Fig. 4 Predicted ‘functional fingerprint’ of palm kernel biomass extracts if consumed in drink form. Molecular mass and log P obtained from LC-MS were used to calculate plasma Tmax (h) of individual primary and secondary metabolites for (A) EM-K whole, (B) WM-K whole, (C) JM-K whole, (D) SM-K whole; and for mass fractions <3 kDa: (E) EM-K <3 kDa, (F) WM-K <3 kDa, (G) JM-K <3 kDa, and (H) SM-K <3 kDa. | |
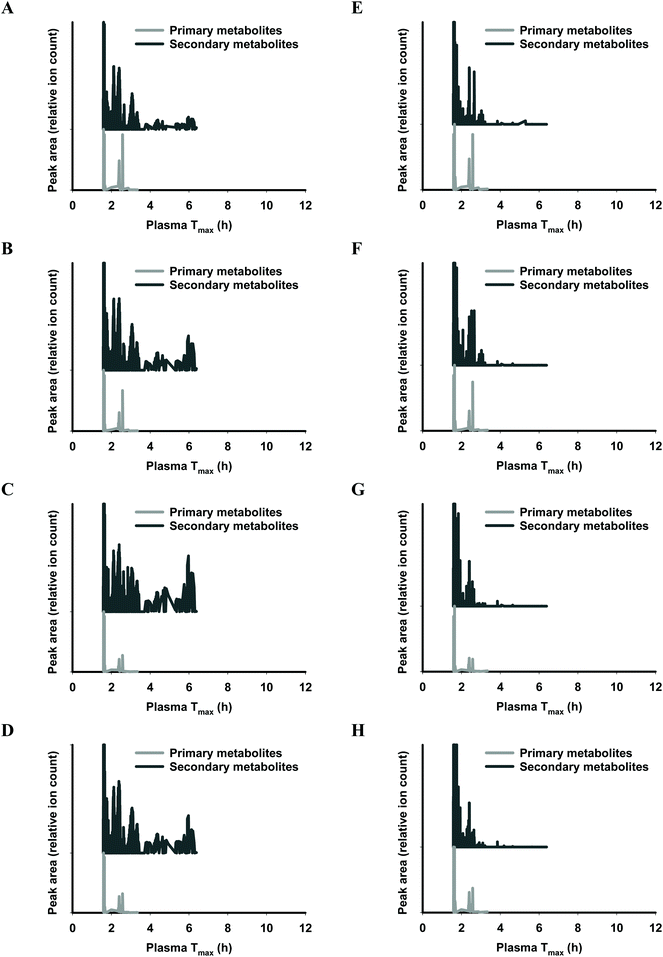 |
| Fig. 5 Predicted ‘functional fingerprint’ of palm kernel biomass extracts if consumed in solid form. Molecular mass and log P obtained from LC-MS were used to calculate plasma Tmax (h) of individual primary and secondary metabolites for (A) EM-K whole, (B) WM-K whole, (C) JM-K whole, (D) SM-K whole; and for mass fractions <3 kDa: (E) EM-K <3 kDa, (F) WM-K <3 kDa, (G) JM-K <3 kDa, and (H) SM-K <3 kDa. | |
Total antioxidant and prooxidant capacity of palm fruit biomass extracts in human plasma in vitro
In vitro total antioxidant and prooxidant capacity of the sixteen palm fruit biomass samples were analysed in human plasma, via measurement of plasma Trolox equivalent antioxidant capacity (TEAC) and plasma concentrations of H2O2. Test sample solids were standardised for total phenolics by the results of the Folin–Ciocalteu assay method. The Folin–Ciocalteu assay chemistry is known to be reactive with ascorbic acid,36 posing a potential source of error in measurement of total phenolics. However, results from LC-MS analysis indicated that there was no ascorbic acid present in any extracts and therefore no contribution by this species in these results. Addition of gallic acid and the extracts to human plasma at concentrations of 0–0.5 mg GAE per mL resulted in linear increases of both plasma TEAC and plasma H2O2 with slopes of the linear regression representing yields of increase as nmol μmol−1 GAE. Highest TEAC yields were observed for the <3 kDa fraction of WM-K, whole fraction of EM-K, and whole fraction of SM-M whilst highest H2O2 production yields were observed for gallic acid standard, the <3 kDa fraction of JM-K, and the whole fraction of JM-M (Fig. 6). Fractionation of the extracts did not result in significant changes in either plasma TEAC or plasma H2O2 yields, except for WM-K and SM-M (Fig. 6). No significant relationship between plasma TEAC and plasma H2O2 yields was observed (Pearson's correlation coefficient r = 0.194, p = 0.456).
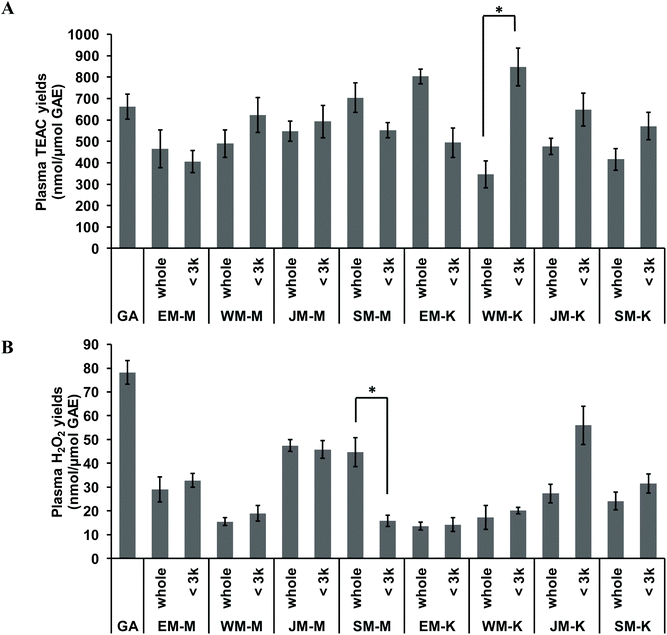 |
| Fig. 6
In vitro total antioxidant and prooxidant capacity of palm fruit biomass extracts measured in human plasma. Plasma Trolox equivalent antioxidant capacity (TEAC) and plasma levels of hydrogen peroxide (H2O2) were analysed in vitro. Gallic acid standards and palm biomass extracts were directly spiked into human plasma at concentrations of 0.05–0.5 mg GAE per mL. Increased plasma TEAC and plasma H2O2 levels followed linear regressions with slopes representing: (A) plasma TEAC yields and, (B) plasma H2O2 yields. Data represent the mean of analysis in duplicate ± standard error. Significant difference between whole extract and <3 kDa fraction was indicated by “*” (p ≤ 0.05, two-tailed t-test). | |
Discussion
Oil-depleted palm fruit biomass as a source of bioactive phytochemicals
This study characterised extracts from oil-depleted palm fruit biomass for their health-related bioactivity potential, focusing on the phytochemical fraction. A selection of chemical analyses and functional assays were conducted including: partial proximate analyses, total phenolic content, in vitro total antioxidant and pro-oxidant capacities and prediction of human absorption properties. Dried extracts contained up to approximately 20% crude protein and ash (Table 1), inferring that the balance of solids were soluble carbohydrates by considering that lipids had been previously removed. The experimentally prepared extracts contained total phenolic contents of 40.75–179.23 mg GAE per g (Table 1), which were relatively higher than levels reported in other oil palm biomass fractions, such as palm fruit extract (18.2 mg GAE per g)37 and defatted palm kernel meal (28.9 mg GAE per g).38 In particular, two of the kernel shell extracts had total phenolic acid contents comparable to that of green tea (218.74 mg GAE per g),28 which is recognised as a rich source of dietary phytochemicals.
For potential dietary applications of any palm fruit biomass, the levels of ash and specific profiles of minerals and metals is an important criterion of safety. In particular, the presence of toxic heavy metals such as Pb and Cd in the aqueous stream of palm vegetation liquor raises concerns about using this biomass stream for human consumption.39 The palm fruit biomass extracts in the present study contained Pb and Cd at <5 μg g−1 (Table 2), which can stay below the tolerable adult limits with appropriate dosing (1.2 μg per kg body weight per day and 25 μg per kg body weight per month, respectively).40 Likewise, levels of Mn and Zn do not pose a risk for exceeding tolerable adult levels (11 and 40 mg day−1, respectively). Therefore, depending on the dosage and intake pattern, the palm fruit biomass extracts in the present study are not expected to present a health risk associated with intake of heavy metal.
Palm fruit biomass extracts were characterised for their ‘functional fingerprints’, which predict time of maximal plasma absorption (Tmax) of the phytochemicals in extracts after consumption, based on molecular mass, lipophilicity descriptor log
P and dietary intake forms.24,28 The prediction of Tmax reported herein reflects the initial passive absorption of phytochemicals into the plasma in their native forms and not their metabolites after passing through the liver or the unabsorbed fraction that are transported to and metabolised in, the gut. However, it is acknowledged that the hepatic and microbial metabolites may have the ability to regulate oxidative stress and inflammation similarly to their parent compounds.41–43
After autoclave-assisted aqueous extraction, the extracts, referred to as ‘whole extracts’, were subjected to molecular weight (MW) fractionation to obtain fractions with MW less than 3 kDa, referred to as ‘<3 kDa’ fraction. Despite having minimal impacts on the total phenolic content (Table 1) and the antioxidant capacity of the extracts (Fig. 6), MW fractionation had significant effect on the functional fingerprints of the secondary metabolites in the extracts (Fig. 2–5). The whole extracts displayed Tmax ranges of 0.4–12 h and 1.6–6.4 h whilst the fractions <3 kDa showed shorter Tmax ranges of 0.4–6 h and 1.6–5.5 h, for intakes in liquid and solid form, respectively. These differences in Tmax ranges are due to the removal of the compounds with higher molecular mass and higher hydrophobicity such as tannins during membrane fractionation. Considering that all molecular features detected by LC-MS analysis were truncated to the MW range of 122–1270 Da (according to the prediction range of the functional fingerprint model), and the membrane fractionation MWCO was 3 kDa, the components in the whole extracts with MW < 3 kDa that did not pass the membrane (i.e., the ‘retentate’) appeared to have been retained due to shape, charge or hydrophobicity properties.44 For all samples, the retentate had higher MW (median 583.43 Da) and higher hydrophobicity (log
P median 5.13) compared to the permeate fractions <3 kDa (median MW 288.07 Da, log
P 1.55) and the whole extracts (median MW 324.14 Da, log
P 1.64) (Fig. S1†). This trend was consistent across substrates, retentates and permeates for all individual palm fruit biomass samples (Fig. S2–S5†).
The functional fingerprints of palm fruit biomass extracts reported herein displayed a broader range of Tmax compared to Tmax values calculated from the reported phytochemical compositional profiles of palm fruit samples. Water-soluble extracts of palm fruit biomass were reported to contain protocatechuic acid, p-hydroxybenzoic acid and caffeoylshikimic acids.37 These main phytochemicals collectively produce calculated Tmax ranges of 0.6–0.8 h and 1.6–1.9 h, for intakes in liquid and solid forms, respectively.24 In the current study, membrane permeates <3 kDa of palm fruit biomass extracts had Tmax ranges of 0.4–6 h and 1.6–5.5 h for intakes as drink and solid forms, respectively. These differences in Tmax ranges highlight the advantage of the functional fingerprinting method, which involves an untargeted approach that can account for all phytochemicals in extracts, and does not focus on a narrow selection of compounds identified by available standards. As such, this research presents a comprehensive functional fingerprint of the rich phytochemical mixture available within oil-depleted palm fruit biomass.
Potential bioefficacy of oil-depleted palm fruit biomass
As tested in human plasma, palm fruit biomass samples produced a dose-dependent increase in Trolox-equivalent antioxidant capacity (TEAC), providing a measure of their potential to directly scavenge reactive oxygen species (ROS) and thereby exert a protective effect by reducing oxidative stress.45 Similar effects have been reported for black tea,46 apple polyphenols,47 red cabbage and grape skin extracts.18
In vitro measures of total antioxidant activity are not consistently correlated to in vivo efficacy of antioxidants.18,46,47 This reflects the low bioavailability of phytochemicals in vivo and the complexity of in vivo signalling and defence systems. Alternately, rather than direct antioxidant activity, it is now widely understood that phytochemicals exert health effects by promoting stress signalling that stimulates the cellular antioxidant defence system of the host.48–50 One of the stress signals produced by phytochemicals has been suggested to be various ROS, including H2O2.51 Therefore, the in vitro capacity of palm fruit biomass phytochemicals to produce H2O2 was measured. Similar to the in vitro TEAC, test samples standardised to total phenolics, produced linear increases in H2O2 in plasma, with yields of 13.56–78.18 nmol H2O2 per μmol GAE (Fig. 6B). Considering the low bioavailability of phytochemicals (human plasma concentrations of phytochemicals in vivo are in the nM to low μM range),52 the yields of H2O2 production by palm fruit phytochemicals observed in the present study are likely to be in a required low range for biological signalling. At high concentrations (≥100 μM), H2O2 are deleterious for cells and responsible for the cytotoxic effects of phytochemicals in cell culture studies.16,17,53 However, at low concentrations (≤50 μM), H2O2 production can be beneficial by stimulating cellular antioxidant defences of the host.54 In support, low levels of H2O2 were reported to enhance wound healing in keratinocytes55 and in mice.56 Likewise, regular exercise and intermittent fasting have been proposed to promote health via the production of low levels of ROS (such as H2O2), that promote adaptive responses to protect against molecular damage and to enhance longevity.51,57 Further research is required to understand the specific concentrations of H2O2 that confer toxic versus beneficial effects, and then to also understand the required intakes of dietary phytochemicals to optimise health benefits.
The present study has characterised key physicochemical and functional properties of palm fruit biomass substrates that can form the basis of their translation into health-promoting foods, supplements, nutraceutical and pharmaceutical products. Application of functional fingerprinting methodology provides insight into fractionation in order to achieve tailored ranges of absorption and strategic bio-matching to lifestyle-related cycles of oxidative stress and inflammation. The research provides a basis to inform further clinical research in order to compare efficacy of palm fruit biomass extracts with other proven phytochemical-rich foods.
Abbreviations
CUPRAC | Cupric reducing antioxidant capacity |
GAE | Gallic acid equivalent |
ICP-OES | Inductively coupled plasma optical emission spectroscopy |
IL | Interleukin |
LC-MS | Liquid chromatography mass spectrometry |
MFE | Molecular feature extraction |
MWCO | Molecular weight cut off |
OSI | Oxidative stress and inflammation |
PC | Phytochemical |
PCAP | Phytochemical absorption prediction |
QTOF | Quadruple time-of-flight |
ROS | Reactive oxygen species |
SOD | Superoxide dismutase |
TEAC | Trolox equivalent antioxidant capacity |
T
max
| Time of pharmacokinetic plasma peak |
Conflicts of interest
Authors declare no conflict of interests.
Acknowledgements
This project was funded by Faculty of Science Cross-Campus Initiative Fund, Monash University. Donation of palm fruit biomass samples from Sime Darby Plantation and The Malaysian Palm Oil Board, and technical assistance by Scott Blundell, Wei Wen Wong, Teng Sin Ooi and Amirah Ibrahim are gratefully acknowledged.
References
- C. Ofori-Boateng and K. T. Lee, Sustainable utilization of oil palm wastes for bioactive phytochemicals for the benefit of the oil palm and nutraceutical industries, Phytochem. Rev., 2013, 12, 173–190 CrossRef CAS.
- R. Oluwafemi, Palm kernel Cake (PKC) utilization in monogastric animal feeding – implications for sustainable livestock development, Internet J. Vet. Med., 2009, 6, 1–5 Search PubMed.
-
J. H. Schmidt, PhD thesis, Aalborg University, 2007.
- M. Abeywardena, I. Runnie, M. Nizar, M. Suhaila and R. Head, Polyphenol-enriched extract of oil palm fronds (Elaeis guineensis) promotes vascular relaxation via endothelium-dependent mechanisms, Asia Pac. J. Clin. Nutr., 2002, 11, S467–S472 CrossRef CAS.
- S. S. Leow, S. D. Sekaran, K. Sundram, Y. Tan and R. Sambanthamurthi, Oil palm phenolics attenuate changes caused by an atherogenic diet in mice, Eur. J. Nutr., 2013, 52, 443–456 CrossRef CAS.
- R. Sambanthamurthi, Y. Tan, K. Sundram, K. C. Hayes, M. Abeywardena, S. S. Leow, S. D. Sekaran, T. G. Sambandan, C. Rha, A. J. Sinskey, K. Subramaniam, S. Fairus and M. B. Wahid, Positive outcomes of oil palm phenolics on degenerative diseases in animal models, Br. J. Nutr., 2011, 106, 1664–1675 CrossRef CAS.
- J. Bolsinger, A. Pronczuk, R. Sambanthamurthi and K. C. Hayes, Anti-diabetic effects of palm fruit juice in the Nile rat (Arvicanthis niloticus), J. Nutr. Sci., 2014, 3, e5 CrossRef.
- C. A. C. Idris, T. Karupaiah, K. Sundram, Y. A. Tan, N. Balasundram, S.-S. Leow, N. S. Nasruddin and R. Sambanthamurthi, Oil palm phenolics and vitamin E reduce atherosclerosis in rabbits, J. Funct. Foods, 2014, 7, 541–550 CrossRef.
- S. Sasidharan, R. Nilawatyi, R. Xavier, L. Y. Latha and R. Amala, Wound healing potential of Elaeis guineensis Jacq leaves in an infected albino rat model, Molecules, 2010, 15, 3186–3199 CrossRef PubMed.
- S. S. Leow, J. Bolsinger, A. Pronczuk, K. C. Hayes and R. Sambanthamurthi, Hepatic transcriptome implications for palm fruit juice deterrence of type 2 diabetes mellitus in young male Nile rats, Genes Nutr., 2016, 11, 29 CrossRef.
- J. M. Jaffri, S. Mohamed, I. N. Ahmad, N. M. Mustapha, Y. A. Manap and N. Rohimi, Effects of catechin-rich oil palm leaf extract on normal and hypertensive rats’ kidney and liver, Food Chem., 2011, 128, 433–441 CrossRef CAS.
- V. Rajavel, M. Z. Abdul Sattar, M. A. Abdulla, N. M. Kassim and N. A. Abdullah, Chronic administration of oil palm (Elaeis guineensis) leaves extract attenuates hyperglycaemic-induced oxidative stress and improves renal histopathology and function in experimental diabetes, Evid. Based Complement. Alternat. Med., 2012, 2012, 195367 Search PubMed.
- N. Yahfoufi, N. Alsadi, M. Jambi and C. Matar, The Immunomodulatory and Anti-Inflammatory Role of Polyphenols, Nutrients, 2018, 10, 1618 CrossRef.
- Y. Zhou, Z. Jiang, H. Lu, Z. Xu, R. Tong, J. Shi and G. Jia, Recent advances of natural polyphenols activators for Keap1-Nrf2 signaling pathway, Chem. Biodivers., 2019, 16, e1900400 CAS.
- W. L. Lee, J. Y. Huang and L. F. Shyur, Phytoagents for cancer management: regulation of nucleic acid oxidation, ROS, and related mechanisms, Oxid. Med. Cell. Longevity, 2013, 2013, 925804 Search PubMed.
- B. Halliwell and M. Whiteman, Measuring reactive species and oxidative damage in vivo and in cell culture: how should you do it and what do the results mean?, Br. J. Pharmacol., 2004, 142, 231–255 CrossRef CAS.
- L. H. Long, M. V. Clement and B. Halliwell, Artifacts in cell culture: rapid generation of hydrogen peroxide on addition of (−)-epigallocatechin, (−)-epigallocatechin gallate, (+)-catechin, and quercetin to commonly used cell culture media, Biochem. Biophys. Res. Commun., 2000, 273, 50–53 CrossRef CAS PubMed.
- S. N. B. Selby-Pham, J. J. Cottrell, F. Dunshea, K. Ng, L. E. Bennett and K. Howell, Dietary phytochemicals promote health by enhancing antioxidant defence in a pig model, Nutrients, 2017, 2017, 758 CrossRef.
- L. M. Wee, L. H. Long, M. Whiteman and B. Halliwell, Factors affecting the ascorbate- and phenolic-dependent generation of hydrogen peroxide in Dulbecco's Modified Eagles Medium, Free Radic. Res., 2003, 37, 1123–1130 CrossRef CAS.
- L. F. Zheng, Q. Y. Wei, Y. J. Cai, J. G. Fang, B. Zhou, L. Yang and Z. L. Liu, DNA damage induced by resveratrol and its synthetic analogues in the presence of Cu(II) ions: mechanism and structure-activity relationship, Free Radical Biol. Med., 2006, 41, 1807–1816 CrossRef CAS PubMed.
- S. Tautkus, A. Irnius, D. Speiciene, J. Barkauskas and A. Kareiva, Investigation of distribution of heavy metals between blood plasma and blood cells, Ann. Chim., 2007, 97, 1139–1142 CrossRef CAS.
- B. Holst and G. Williamson, Nutrients and phytochemicals: from bioavailability to bioefficacy beyond antioxidants, Curr. Opin. Biotechnol., 2008, 19, 73–82 CrossRef CAS PubMed.
- M. D'Archivio, C. Filesi, R. Varì, B. Scazzocchio and R. Masella, Bioavailability of the polyphenols: status and controversies, Int. J. Mol. Sci., 2010, 11, 1321–1342 CrossRef PubMed.
- S. N. B. Selby-Pham, R. B. Miller, K. Howell, F. Dunshea and L. E. Bennett, Physicochemical properties of dietary phytochemicals can predict their passive absorption in the human small intestine, Sci. Rep., 2017, 7, 1931 CrossRef PubMed.
- A. Stalmach, S. Troufflard, M. Serafini and A. Crozier, Absorption, metabolism and excretion of Choladi green tea flavan-3-ols by humans, Mol. Nutr. Food Res., 2009, 53, S44–S53 CrossRef PubMed.
- D. M. Gustin, K. A. Rodvold, J. A. Sosman, V. Diwadkar-Navsariwala, M. Stacewicz-Sapuntzakis, M. Viana, J. A. Crowell, J. Murray, P. Tiller and P. E. Bowen, Single-dose pharmacokinetic study of lycopene delivered in a well-defined food-based lycopene delivery system (tomato paste-oil mixture) in healthy adult male subjects, Cancer Epidemiol. Biomarkers Prev., 2004, 13, 850–860 CAS.
- Y. Huang, E. Park, I. Edirisinghe and B. M. Burton-Freeman, Maximizing the health effects of strawberry anthocyanins: understanding the influence of the consumption timing variable, Food Funct., 2016, 7, 4745–4752 RSC.
- S. N. B. Selby-Pham, K. S. Howell, F. R. Dunshea, J. Ludbey, A. Lutz and L. E. Bennett, Statistical modelling coupled with LC-MS analysis to predict human intestinal absorption of phytochemical mixtures, Food Chem., 2018, 245, 353–363 CrossRef CAS.
-
R. Sambanthamurthi, Y. A. Tan and K. Sundram, Treatment of vegetation liquors delivered from oil-bearing fruit, US Patent, US7387802B2, 2008 Search PubMed.
- F. W. Sosulski and G. I. Imafidon, Amino acid composition and nitrogen-to-protein conversion factors for animal and plant foods, J. Agric. Food Chem., 1990, 38, 1351–1356 CrossRef CAS.
- V. L. Singleton and J. A. Rossi, Colorimetry of total phenolics with phosphomolybdic-phosphotungstic acid reagents, Am. J. Enol. Vitic., 1965, 16, 144–158 CAS.
- Z. Yu, F. Song, Y. C. Jin, W. M. Zhang, Y. Zhang, E. J. Liu, D. Zhou, L. L. Bi, Q. Yang, H. Li, B. L. Zhang and S. W. Wang, Comparative pharmacokinetics of gallic acid after oral administration of gallic acid monohydrate in normal and isoproterenol-induced myocardial infarcted rats, Front. Pharmacol., 2018, 9, 328 CrossRef PubMed.
- S. Shahrzad, K. Aoyagi, A. Winter, A. Koyama and I. Bitsch, Pharmacokinetics of gallic acid and its relative bioavailability from tea in healthy humans, J. Nutr., 2001, 131, 1207–1210 CrossRef CAS.
- S. S. Marques, L. M. Magalhaes, I. V. Toth and M. A. Segundo, Insights on antioxidant assays for biological samples based on the reduction of copper complexes-the importance of analytical conditions, Int. J. Mol. Sci., 2014, 15, 11387–11402 CrossRef.
-
S. P. Wolff, in Methods in Enzymology, Academic Press, 1994, vol. 233, pp. 182–189 Search PubMed.
- J. D. Everette, Q. M. Bryant, A. M. Green, Y. A. Abbey, G. W. Wangila and R. B. Walker, Thorough study of reactivity of various compound classes toward the Folin−Ciocalteu reagent, J. Agric. Food Chem., 2010, 58, 8139–8144 CrossRef CAS PubMed.
- R. Sambanthamurthi, Y. Tan, K. Sundram, M. Abeywardena, T. G. Sambandan, C. Rha, A. J. Sinskey, K. Subramaniam, S. S. Leow, K. C. Hayes and M. B. Wahid, Oil palm vegetation liquor: a new source of phenolic bioactives, Br. J. Nutr., 2011, 106, 1655–1663 CrossRef CAS PubMed.
- S. K. Chang, A. Ismail, T. Yanagita, N. M. Esa and M. T. H. Baharuldin, Biochemical characterisation of the soluble proteins, protein isolates and hydrolysates from oil palm (Elaeis guineensis) kernel, Food Biosci., 2014, 7, 1–10 CrossRef CAS.
- P. F. Rupani, R. P. Singh, M. H. Ibrahim and N. Esa, Review of current palm oil mill effluent (POME) treatment methods: vermicomposting as a sustainable practice, World Appl. Sci. J., 2010, 10, 1190–1201 CAS.
-
FSANZ, The 25th Australian total diet study, Food Standards Australia New Zealand, Canberra, 2019 Search PubMed.
- S. Donnini, F. Finetti, L. Lusini, L. Morbidelli, V. Cheynier, D. Barron, G. Williamson, J. Waltenberger and M. Ziche, Divergent effects of quercetin conjugates on angiogenesis, Br. J. Nutr., 2006, 95, 1016–1023 CrossRef CAS PubMed.
- T. Koga and M. Meydani, Effect of plasma metabolites of (+)-catechin and quercetin on monocyte adhesion to human aortic endothelial cells, Am. J. Clin. Nutr., 2001, 73, 941–948 CrossRef CAS PubMed.
- S. C. Forester and A. L. Waterhouse, Gut metabolites of anthocyanins, gallic acid, 3-O-methylgallic acid, and 2,4,6-trihydroxybenzaldehyde, inhibit cell proliferation of Caco-2 cells, J. Agric. Food Chem., 2010, 58, 5320–5327 CrossRef CAS PubMed.
- A. Cassano, C. Conidi, R. Ruby-Figueroa and R. Castro-Munoz, Nanofiltration and tight ultrafiltration membranes for the recovery of polyphenols from agro-food by-products, Int. J. Mol. Sci., 2018, 19, 351 CrossRef.
- R. Apak, K. Guclu, B. Demirata, M. Ozyurek, S. E. Celik, B. Bektasoglu, K. I. Berker and D. Ozyurt, Comparative evaluation of various total antioxidant capacity assays applied to phenolic compounds with the CUPRAC assay, Molecules, 2007, 12, 1496–1547 CrossRef CAS.
- A. Cherubini, M. F. Beal and B. Frei, Black tea increases the resistance of human plasma to lipid peroxidation in vitro, but not ex vivo, Free Radical Biol. Med., 1999, 27, 381–387 CrossRef CAS PubMed.
- S. B. Lotito and B. Frei, Relevance of apple polyphenols as antioxidants in human plasma: contrasting in vitro and in vivo effects, Free Radical Biol. Med., 2004, 36, 201–211 CAS.
- J. E. Drew, Cellular defense system gene expression profiling of human whole blood: opportunities to predict health benefits in response to diet, Adv. Nutr., 2012, 3, 499–505 CrossRef CAS PubMed.
- J. W. Finley, A. N. Kong, K. J. Hintze, E. H. Jeffery, L. L. Ji and X. G. Lei, Antioxidants in foods: state of the science important to the food industry, J. Agric. Food Chem., 2011, 59, 6837–6846 CrossRef CAS PubMed.
- A. N. Kong, E. Owuor, R. Yu, V. Hebbar, C. Chen, R. Hu and S. Mandlekar, Induction of xenobiotic enzymes by the MAP kinase pathway and the antioxidant or electrophile response element (ARE/EpRE), Drug Metab. Rev., 2001, 33, 255–271 CrossRef CAS PubMed.
- P. C. Tapia, Sublethal mitochondrial stress with an attendant stoichiometric augmentation of reactive oxygen species may precipitate many of the beneficial alterations in cellular physiology produced by caloric restriction, intermittent fasting, exercise and dietary phytonutrients: “Mitohormesis” for health and vitality, Med. Hypotheses, 2006, 66, 832–843 CrossRef CAS PubMed.
- D. Del Rio, A. Rodriguez-Mateos, J. P. Spencer, M. Tognolini, G. Borges and A. Crozier, Dietary (poly)phenolics in human health: structures, bioavailability, and evidence of protective effects against chronic diseases, Antioxid. Redox Signaling, 2013, 18, 1818–1892 CrossRef CAS PubMed.
- P. C. Chai, L. H. Long and B. Halliwell, Contribution of hydrogen peroxide to the cytotoxicity of green tea and red wines, Biochem. Biophys. Res. Commun., 2003, 304, 650–654 CrossRef CAS.
- N. Ohguro, M. Fukuda, T. Sasabe and Y. Tano, Concentration dependent effects of hydrogen peroxide on lens epithelial cells, Br. J. Ophthalmol., 1999, 83, 1064–1068 CrossRef CAS PubMed.
- A. E. Loo and B. Halliwell, Effects of hydrogen peroxide in a keratinocyte-fibroblast co-culture model of wound healing, Biochem. Biophys. Res. Commun., 2012, 423, 253–258 CrossRef CAS PubMed.
- A. E. Loo, Y. T. Wong, R. Ho, M. Wasser, T. Du, W. T. Ng and B. Halliwell, Effects of hydrogen peroxide on wound healing in mice in relation to oxidative damage, PLoS One, 2012, 7, e49215 CrossRef CAS PubMed.
- Z. Radak, H. Y. Chung and S. Goto, Exercise and hormesis: oxidative stress-related adaptation for successful aging, Biogerontology, 2005, 6, 71–75 CrossRef CAS PubMed.
Footnote |
† Electronic supplementary information (ESI) available: Comparison of molecular features detected by LC-MS in whole extract, permeate (fraction that passed the 3 kDa MWCO membrane) and retentate (fraction that did not pass the 3 kDa MWCO membrane) of all samples (Fig. S1); comparison of molecular features detected by LC-MS in whole extract, permeate and retentate of (A) EM-M, and (B) WM-M (Fig. S2); comparison of molecular features detected by LC-MS in whole extract, permeate and retentate of (A) JM-M, and (B) SM-M (Fig. S3); comparison of molecular features detected by LC-MS in whole extract, permeate and retentate of (A) EM-K, and (B) WM-K (Fig. S4); comparison of molecular features detected by LC-MS in whole extract, permeate and retentate of (A) JM-K, and (B) SM-K (Fig. S5). See DOI: 10.1039/c9fo01149h |
|
This journal is © The Royal Society of Chemistry 2020 |
Click here to see how this site uses Cookies. View our privacy policy here.