DOI:
10.1039/C9FO01698H
(Paper)
Food Funct., 2020,
11, 435-447
Lactobacillus rhamnosus from human breast milk shows therapeutic function against foodborne infection by multi-drug resistant Escherichia coli in mice
Received
28th July 2019
, Accepted 14th November 2019
First published on 12th December 2019
Abstract
The emergence of multi-drug resistant (MDR) pathogens greatly challenges the development of new drugs. Probiotics with the ability to inhibit MDR pathogens offer advantages over chemical antibiotics and drugs due to increased safety and fewer side effects. This study reports that Lactobacillus rhamnosus SHA113 (isolated from breast milk) significantly inhibited MDR Escherichia coli both in vitro and in vivo. MDR E. coli caused more severe inflammatory effects. TNF-α and IL-6 levels increased, while the IL-10 content decreased in serum. MDR E. coli caused disturbance in the gut microbial balance, increased the total coliform, decreased lactic acid bacteria in feces, decreased Firmicutes, and increased both Bacteroidetes and Proteobacteria. At the end of the curing treatment, ampicillin (AMP) treatment significantly reduced lactic acid bacteria compared to total coliform in feces and exacerbated the increase of Proteobacteria caused by MDR E. coli. L. rhamnosus SHA113 treatment resulted in a more significant and faster decrease of total coliform in feces and a significant decrease of Proteobacteria in the gut microbiota. The increase of total coliform in feces (caused by MDR E. coli infection) was positively correlated with IL-6 and TNF-α and negatively correlated with IL-10 in serum. However, the increase of lactic acid bacteria in feces (caused by L. rhamnosus SHA113 treatment) was negatively correlated with serum TNF-α, indicating that SHA113 exerted anti-inflammatory effects. These results suggest that L. rhamnosus SHA113 has great potential for inhibiting infections by MDR E. coli and for regulating the gut flora balance.
1. Introduction
The extensive use of broad-spectrum antibiotics has led to an abundance of multi-drug resistant (MDR) pathogens and caused a crisis in drug efficiency for the inhibition of MDR pathogens. The rise of antimicrobial resistance is an urgent public health threat that, in the absence of intervention, may severely limit the effectiveness of common antibiotic treatments.1 The emergence of MDR pathogens increases the infection rate and the difficulty to find a cure. More than 30% of all hospital-acquired infections are caused by Gram-negative bacteria, of which E. coli is responsible for most urinary tract infections.2 Reports from Europe, Asia, and India highlight MDR E. coli as a global problem.3 Hussain et al. reported that MDR E. coli infection in the retail poultry market in India was as high as 68% in broilers and 64% in free-range chickens.4 There is even a high occurrence of MDR pathogens in the intensive care unit (ICU) of Ugandan hospitals.5 The increasing incidence of community-acquired urinary tract infections (UTIs) due to MDR E. coli demonstrates that MDR pathogenic E. coli may be present in food such as poultry or pork and thus infect humans via diet.6
Since the spread of antibiotic resistance among pathogens is much faster than the development of new drugs, MDR pathogens have become a global public health problem and forced people to find new ways to combat MDR pathogens. Currently, many protein kinases,7 polypeptides,8 and quinazoline9 have been developed as antibiotic adjuvants against MDR bacteria.
Several plant extracts and extracts of endogenous bacteria have been found to have the ability to efficiently treat MDR E. coli. For example, berberine, a traditional Chinese medicine, strongly binds to the nucleic acid of pathogenic bacteria and thus shows significant antibacterial effect on MDR E. coli.10 Methyl quercetin, produced by an endophytic fungus from the bark of Eucommia, was also found to efficiently inhibit foodborne MDR E. coli, Staphylococcus aureus, and dendritic Salmonella.11 Procyanidins showed inhibitory effects on the adhesion of MDR E. coli to urinary epithelial cells.12
In addition to natural products, live probiotics, mostly lactic acid bacteria (LAB), also have great potential to inhibit pathogenic bacteria and show potential for controlling MDR E. coli infections.13 The occurrence of abundant living probiotics in the gut microbiota is good for the health of the host.14 At present, the development and application of probiotics have become a hotspot in the research of food and medicine. Probiotics are beneficial in many ways; for example, they protect against the colonization of intestinal pathogens by stimulating epithelial cells to increase mucosal integrity.15 They also affect homeostasis, inflammation, and immunopathology as immunosuppressants or activators.16 Probiotics also provide functional end-products such as organic acids that can be utilized by the host, and effectively compete with pathogens for colonization and disease prevention.17 It has been shown that live probiotic cells and products fermented by them can inhibit pathogenic bacteria, including E. coli, Enterococcus faecalis,18 and Salmonella.19 Among FDA-approved probiotics, Lactobacillus rhamnosus plays an important role in physiological function and health care by regulating the intestinal flora, preventing and treating diarrhea,20 improving immunity and fighting cancers.21L. rhamnosus and its metabolites were found to inhibit six Gram-positive and nine Gram-negative pathogenic bacteria, and eight Candida species in vitro.22In vivo experiments conducted in animals indicated that L. rhamnosus showed good probiotic effects against Salmonella typhimurium infection.23 However, reports on the inhibitory effect of L. rhamnosus on MDR pathogenic bacteria and its influence on the intestinal barrier and intestinal flora are not available.
This study reports the great potential of L. rhamnosus from breast milk24 to inhibit MDR E. coli both in vitro and in vivo, as well as its effects on the intestinal barrier, intestinal permeability, expression of inflammatory factors, and intestinal flora.
2. Materials and methods
2.1. Microorganisms and chemicals
E. coli QBQ009, with resistance to ampicillin (AMP), streptomycin (STR), chloramphenicol (CHL), tetracycline (TE), and nalidixic acid (NAL), was used as a MDR pathogen in this study. It was isolated from a pig pen in Fufeng, Shaanxi province, China, and donated by Prof. Baowei Yang of Northwest A&F University, China. E. coli ATCC25922 was purchased from the American Tissue Culture Collection (ATCC) and was used as a control non-drug resistant pathogen. Both QBQ009 and ATCC25922 were cultured in Luria–Bertani medium (LB) for 12 h, at 37 °C, 200 rpm to prepare live cells in the middle of the logarithmic growth phase.
L. rhamnosus SHA113 (coded as CCTCCM2017839) was previously isolated from the breast milk of healthy women and was stored in the China Typical Microorganism Preservation Center (Wuhan, Hubei province, China) and was used as the probiotic in this study. It was prepared by culturing in deMan, Rogosa, and Sharpe (MRS) broth (Hopebio, Qingdao, China) at 37 °C for 24 h under anaerobic conditions without shaking.
2.2. Inhibitory effect of L. rhamnosus against MDR E. coli in vitro
The standard agar-well diffusion method was used to determine the antibiotic sensitivity of MDR E. coli and the antibacterial activity of L. rhamnosus SHA113 based on previously reported methods11 with slight modifications. For drug resistance capability measurement, E. coli QBQ009 and ATCC25922 were separately inoculated on LB plates at a concentration of 106 CFU mL−1, followed by addition of 100 μL AMP (32 g mL−1), TE (16 g mL−1), norfloxacin (FPA; 8 g mL−1), chloramphenicol (CHL; 32 g mL−1), and aminoglycosides (64 g mL−1) in punched holes (diameter: 8 mm), and the liquid culture of L. rhamnosus SHA113. After cultivation for 24 h, the diameter of the bacteriostatic zone (DIZ, not including the diameter of the well) was measured. Each test was conducted in triplicate.
To evaluate the antibacterial activity of L. rhamnosus SHA113 against E. coli QBQ009 in a state of co-existence, they were separately cultivated (with an inoculum size of 106 CFU mL−1) in a mixture of LB and MRS liquid media (at a ratio of 1
:
1) for 12 h before they were collected after centrifugation and transferred together to fresh mixture medium. E. coli QBQ009 alone transferred to fresh mixture medium was used as a control to investigate the influence of the medium. All inoculates were continuously cultivated at 37 °C. During cultivation, the amount of living E. coli was tested at 0 h, 8 h, 12 h, 14 h, 16 h, 20 h, and 24 h using McConkey Agar medium (Hopebio, Qingdao, China).
2.3. Inhibitory effect of L. rhamnosus against MDR E. coli infection in mice
The animal experiment was approved by the Animal Protection and Use Committee of Northwest Polytechnic University (license number: SCXXK 2017003). Experiments were conducted according to the animal protection and guidelines of the National Institutes of Health. Sixty-three adult male Kunming (KM) mice (18–22 g) were purchased from the Experimental Animal Center of Xi'an Jiaotong University. During the experimental period, the mice were kept in the Animal Experiment Center of Northwest Polytechnic University at room temperature (22 ± 2 °C), a relative humidity of 50%, and a light and dark cycle of 12 h each. During the experiments, healthy male mice were randomly divided into seven groups with nine mice per group. Fig. 1 shows the experimental scheme.
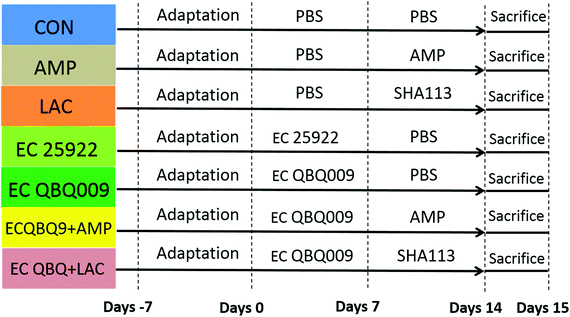 |
| Fig. 1 Time points and treatments of the study. Abbreviations: CON, feeding PBS; LAC, feeding L. rhamnosus SH A113 (109 cfu mL−1) at a dose of 10 ml per kg BW per day; AMP, feeding ampicillin at a dose of 10 ml kg−1 per day; EC 25922, feeding E. coli 25922 (108 cfu mL−1) at a dose of 10 ml per kg BW per day; EC QBQ009, feeding MDR E. coli QBQ009 (108 cfu mL−1) at a dose of 10 ml per kg BW per day; EC QBQ + LAC, feeding MDR E. coli QBQ009 (108 cfu mL−1) at a dose of 10 ml per kg BW per day together with L. rhamnosus SH A113 (109 cfu mL−1) at a dose of 10 ml per kg BW per day; EC QBQ + AMP, feeding MDR E. coli QBQ009 (108 cfu mL−1) at a dose of 10 ml per kg BW per day together with ampicillin at a dose of 10 mL per kg BW per day. | |
The experiments were conducted for 14 days including the infection period and the treatment period. The arrangement is detailed in Fig. 1. The control group was fed phosphate buffer (PBS, pH = 7.4) throughout the whole experiment.
2.4. Measurements of animal parameters
Body weight, bacterial amounts in feces, and levels of immunological factors and intestinal microbiota were tested for all groups. Mechanisms for more significant inhibitory effect of L. rhamnosus SHA113 against MDR E. coli compared to AMP were analyzed according to the changes in intestinal permeability, expression of intestinal adhesion proteins, and necropsy and histopathological characteristics of the following groups: control, EC QBQ, LAC, EC QBQ + LAC, and EC QBQ + AMP.
2.4.1. Daily body weight and bacterial amount in feces.
The body weight of the animals was recorded every day; fresh feces were collected and the total number of coliform colonies and lactic acid bacteria was determined using McCabe selective culture medium and M17 selective culture medium, respectively. The mental state, occurrence of diarrhea, and blood in the stool of mice were also observed and recorded.
2.4.2. Organ index after sacrifice.
Postmortem examination was performed to preliminarily examine the external surface, the thoracic and abdominal cavities, and their contents. The spleen, kidneys, and liver were weighed shortly after dissection.
2.4.3. Levels of immunological factors in serum.
On the 15th day, the mice were deprived of food and water for 7 h before they were sacrificed. Blood samples were collected from the eyes before the mice were killed via cervical decapitation. The blood samples were immediately placed in a centrifuge tube in a 37 °C water bath pot for 1 to 2 h before they were stored at 4 °C for 3–4 h. After the retraction of the blood clot, serum was obtained after centrifugation at 3000 rpm for 15 min as the supernatant. Then, interleukin-6 (IL-6), interleukin-10 (IL-10), and TNF-α contents in the serum were tested using ELISA kits (Thermo Fisher Scientific, Shanghai, China) according to the operating instructions and ref. 25.
2.4.4. Measurement of the gut microbiota in the cecum.
Six mice were randomly selected from each group to collect their cecum contents and extract their DNA. Frozen DNA samples were stored at −20 °C and sent to Personalbio (Shanghai, China) for sequencing. For each DNA sample, the V3 and V4 fragments of 16S rDNA were analyzed by PCR with denaturation gradient gel electrophoresis (DGGE). DNA fragments were sequenced using the Illumina MiSeq platform.26 The screening, processing, and quality control of the original sequencing data were performed by Personalbio (Shanghai, China).
For the alpha diversity of the gut microbiota, the quantitative insights into microbial ecology (QIIME) software for microbial community analysis was used to merge the obtained high-quality sequences at a level of 97% sequence similarity and divide the operational taxonomic units (OTUs). The highest abundance sequence of each OTU matrix was selected as the representative sequence of the OTU.27
For the beta diversity of the gut microbiota, after linear transformation, PCA analysis projected the original high-dimensional data (such as the OTU abundance matrix of the flora) into the spatial coordinate system (namely the principal component) with lower dimensions through a combination of linear transformations. The goal was to achieve dimensionality reduction and data structure simplification, and show the natural distribution of the samples.28
2.5. Statistical analysis
Figures were drawn using GraphPad, Prism6, or R3.5.3. Significance analysis was conducted by one-way analysis of variance (ANOVA) and Student's t-test using SPSS19.0 (Chicago, IL, United States). All data are shown as means ± standard errors of the mean (SEM), and P < 0.05 indicated statistically significant differences.
3. Results and discussion
3.1. Inhibitory effect of L. rhamnosus on MDR E. coli in vitro
As shown in Fig. 2A, EC QBQ009 showed resistance to AMP (32 g mL−1), TE (16 g mL−1), and FPA (8 g mL−1), while EC 25922 was only resistant to TE and FPA, but not to AMP. Therefore, AMP was selected for use in further experiments to investigate the drug-resistance capability of EC QBQ009.
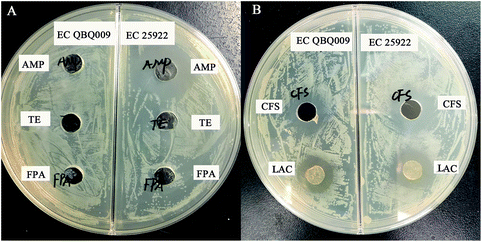 |
| Fig. 2 Inhibitory effect of L. rhamnosus SHA113 and ampicillin (AMP) on AMP-resistant and non-AMP-resistant E. coli. (A) Inhibitory effects of different antibiotics on E. coli strains (106 cfu mL−1). (B) Inhibitory effect of the cell-free broth and cells of L. rhamnosus on E. coli strains. Abbreviations: AMP, ampicillin (32 g ml−1); TE, tetracycline (16 g ml−1); FPA, norfloxacin (8 g ml−1); CFS, cell-free broth of L. rhamnosus SHA 113 culture; LAC, live cells of L. rhamnosus SHA113. | |
Both cells and cell-free culture broth of L. rhamnosus SHA113 significantly inhibited the growth of AMP-resistant E. coli QBQ009 and non-AMP-resistant E. coli ATCC25922 (Fig. 2B).
To verify the inhibitory effect of L. rhamnosus SHA113 on E. coli QBQ009 in liquid culture, a mixture of liquid LB and MRS media was used to cultivate E. coli QBQ009 alone and together with L. rhamnosus SHA113 after transfer to fresh medium. As a result, the cooccurrence of L. rhamnosus SHA113 significantly decreased the amount of live E. coli QBQ009 compared to E. coli QBQ009 without L. rhamnosus SHA113 (Fig. 3). This indicated that the presence of L. rhamnosus SHA113 cells significantly reduced the viability and even killed the cells of MDR E. coli QBQ009.
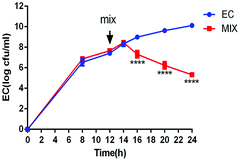 |
| Fig. 3 Inhibitory effect of L. rhamnosus SHA113 cells on MDR E. coli QBQ009. **** indicates that the data were significantly different from that of the EC group at p < 0.0001 (n = 3). EC, systems inoculated only with E. coli QBQ009; MIX, systems containing both E. coli QBQ009 and L. rhamnosus SHA113. The medium was the mixture of LB and MRS media at a ratio of 1 : 1. Transfer of E. coli QBQ009 cells to fresh medium and the addition of L. rhamnosus SHA113 cells occurred at 12 h. | |
3.2. Pathogenic effects of MDR E. coli
It has been reported that pathogenic E. coli infection can cause intestinal flora disorder and diarrhea in mice. According to the preliminary experiments, 1 × 108 cfu mL−1 of E. coli caused more significant diarrhea and a series of adverse reactions compared to other doses (1 × 106 cfu mL−1 and 1 × 107 cfu mL−1). Therefore, both E. coli QBQ009 and ATCC25922 were used at the dose of 1 × 108 cfu mL−1 in this study. As a result, both E. coli QBQ009 and ATCC25922 infections caused obvious signs of mental malaise, capillary inversion, slow movement, diarrhea, and even hematochezia after infection for 3 days.
3.2.1.
E. coli infection caused reduction of body weight and decrease of lactic acid bacteria in feces and induced inflammation.
Fig. 4A shows that the body weight of mice increased in all groups. However, both E. coli QBQ009 and ATCC 25922 infections reduced the body weight increase compared to healthy mice during the whole experimental period, although this effect was not significant.
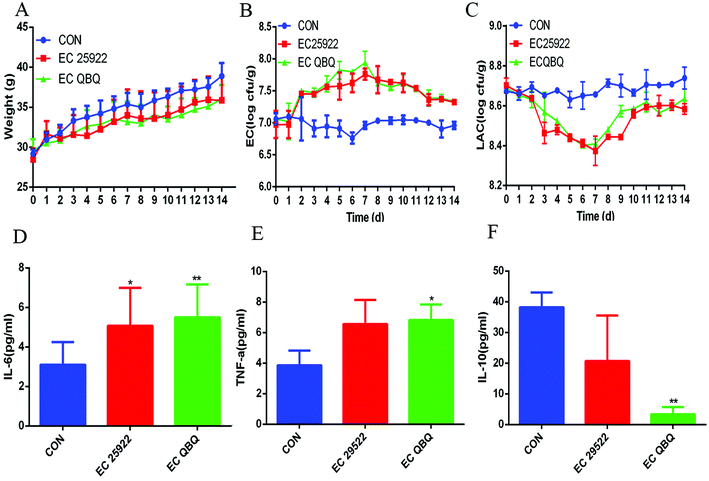 |
| Fig. 4 Body weight, live coliform, and lactic acid bacteria in feces and inflammatory cytokines in serum. (A) Body weight; (B) total coliform; (C) total amount of detected lactic acid bacteria; (D) IL-6 in serum; (E) TNF-α in serum; and (F) IL-10 in serum. Data are expressed as mean ± SEM (n = 6), *P < 0.05, **P < 0.01, and ***P < 0.001. All data were compared with the control group (CON). EC 25922 and EC QBQ indicate the infection of non-AMP resistant E. coli 25922 and AMP-resistant E. coli QBQ 009, respectively. This is the same in other figures. | |
Compared to the control group, the amount of total coliform in feces was significantly higher, while the amount of lactic acid bacteria was significantly lower in all E. coli infected groups. The amount of total coliform in feces increased during the E. coli feeding period (the first 7 days) and decreased after stopping the E. coli feeding in the following 7 days (Fig. 4B). In contrast, the amount of lactic acid bacteria in feces decreased during the E. coli feeding period and increased after stopping the E. coli feeding (Fig. 4C). The decrease of total coliform and increase of total lactic acid bacteria after stopping E. coli infection indicated that the gut microbiota could spontaneously repair the malfunction of and imbalance in the microbiota caused by E. coli infection even without any directed intervention.
However, the inflammation and gut microbiota imbalance were not completely repaired during the automatic repair process. As shown in Fig. 4D–F and 5, after automatic repairing for 7 days, both MDR E. coli QBQ009 and ATCC 25922 infected groups still showed significant inflammatory signals, such as increased IL-6 and TNF-α levels and decreased IL-10 levels. In comparison, AMP-resistant E. coli QBQ009 caused a more significant decrease in IL-10 levels than non-AMP-resistant E. coli ATCC25922. This was consistent with previous reports where bacterial infections could cause abnormalities in inflammatory cytokines, including TNF-α, IL-6, and IL-10, through innate and acquired immunity.29
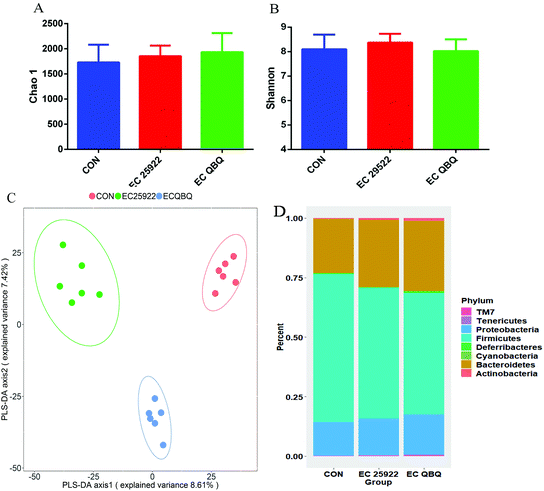 |
| Fig. 5 Effect of E. coli infection on intestinal flora. (A) The Chao-1 index and (B) Shannon index of the cecum microflora. (C) PLS-DA (Partial Least Squares Discriminant Analysis) of the community composition at the phylum level. (D) Abundance of the horizontal community at the phylum level (n = 6). | |
3.2.2.
E. coli infection changes the intestinal flora in the cecum.
Chao1 and Shannon index analyses showed that E. coli QBQ009 and ATCC 25922 infection caused slight increases and slight changes in the homogeneous degree of intestinal microflora richness, but the effect was not significant (Fig. 5A and B). The beta diversity of the intestinal flora differed significantly among the CON, EC, QBQ, and EC 25922 groups, indicating that E. coli infection caused a significant change in the microbial distribution at the genus level. The shift of microbial distribution caused by E. coli QBQ009 infection differed significantly from that caused by ATCC 25922 infection.
PLS-DA analysis is based on the Partial Least Squares regression model as a supervised pattern recognition method that can discriminate community structure data based on the given sample distribution/grouping information. PLS-DA reordered the samples in the new low-dimensional coordinate system by looking for the maximum covariance of the species abundance matrix and the given sample distribution/grouping information. Using R software, the PLS-DA discrimination model was constructed according to the species abundance matrix and sample grouping data. Variable importance in projection coefficients of each species was also calculated (the VIP value should be >1, and the larger the value, the greater the contribution of this species to inter-group differences). This suggests that the community structure of the microbiome in the E. coli infected mice differed significantly from that in the control group.
At the phylum level, E. coli infection decreased the abundance of Firmicutes and increased the abundances of Bacteroidetes and Proteobacteria in the microbial community compared to the control group. Infection with E. coli QBQ 009 led to the same trends, but caused more significant changes with regard to these aspects compared to ATCC 25922. In terms of classification, most lactic acid bacteria belong to Firmicutes, while E. coli belongs to Proteobacteria. Therefore, such changes were consistent with the increase of total coliform and the decrease of lactic acid bacteria in feces. This also illustrated that E. coli infection could shift both the fecal and cecum microflora. Overall, AMP-resistant MDR E. coli QBQ 009 tended to cause greater changes than non-AMP resistant E. coli 25922, although these effects were not significant.
3.3. Limitation of AMP in curing MDR E. coli infection
According to the results of the in vitro experiments, MDR E. coli QBQ009 was resistant to AMP, while E. coli ATCC 25922 was not. To verify the limits of AMP on MDR E. coli infection in vivo, AMP was used to treat MDR E. coli QBQ 009 infection in mice.
3.3.1. AMP treatment increased body weight, reduced lactic acid bacteria in feces, did not reduce total coliform, and caused anti-inflammatory effects.
As shown in Fig. 6, compared to healthy mice, oral intake of AMP slightly influenced body weight, caused a slight decrease of total coliform and a significant decrease of lactic acid bacteria in feces, significantly decreased IL-6 and slightly decreased IL-10, while increasing the TNF-α level in serum. This indicates its effects on inflammation induction and effects on the activities of immune cells. This implies that residual AMP in foods has potential harmful effects and causes disorders of the immune system gut microbial balance in healthy animals.
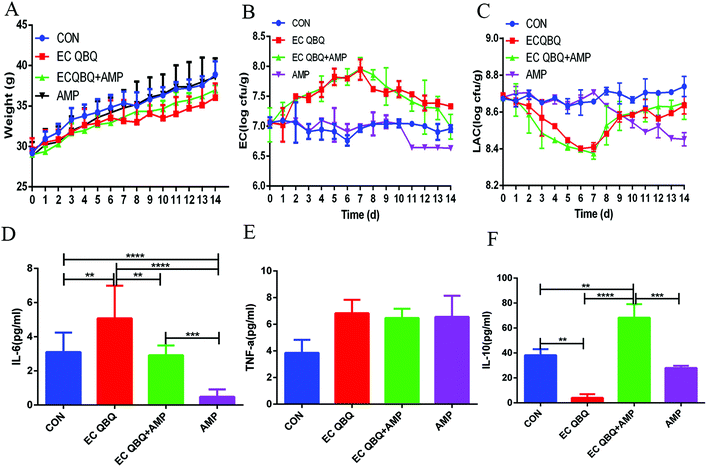 |
| Fig. 6 Body weight, detected total coliform, and lactic acid bacteria in feces, and inflammatory cytokines in serum. (A) Body weight; (B) detected total viable coliform and (C) lactic acid bacteria in serum. IL-6 (D), TNF-α (E), and IL-10 (F) levels in serum. Data represent mean ± SEM (n = 6), *P < 0.05, **P < 0.01, and ***P < 0.001. | |
For MDR E. coli infected groups, treatment with AMP could not reduce total coliform at most treatment stages but significantly reduced the amount of lactic acid bacteria immediately after treatment. At the end of the curing treatment, the IL-6 level in serum almost decreased to the same level as that of the healthy control, while the IL-10 and TNF-α levels were still higher than those of healthy mice. These results indicate that AMP treatment could not cure the MDR E. coli infection although it caused anti-inflammatory effects.
3.3.2. Effects of AMP on the intestinal flora.
Fig. 7 indicates that oral intake of AMP exerted a slight influence on the richness and humongous distribution of gut microbiota richness (Fig. 7A and B). Similar changes of microbial distribution were found at the genus level to those observed in E. coli infection (Fig. 7C) in healthy mice. Compared to MDR E. coli infected mice, treatment with AMP reduced the Chao1 and Shannon indexes, but these effects were not significant, and greatly shifted the microbial distribution at the genus level (Fig. 7D).
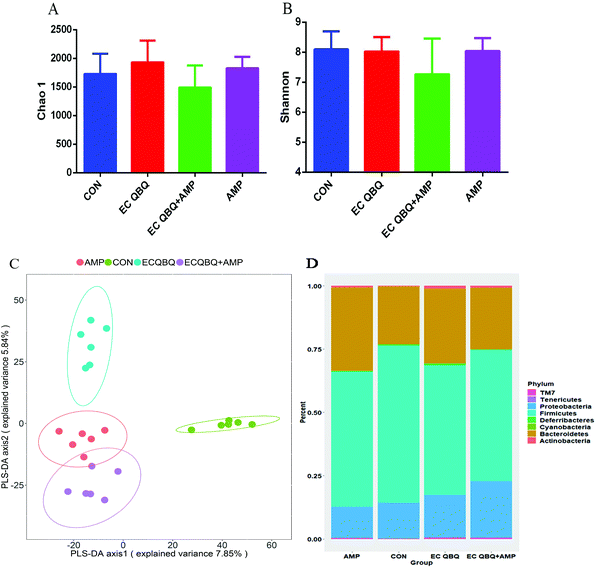 |
| Fig. 7 Effect of AMP on the intestinal flora. Alpha diversity of the intestinal microflora. (A) Chao-1 index and (B) Shannon index of mouse cecum microflora. Beta diversity of the gut microbiota: (C) PLS-DA (Partial Least Squares Discriminant Analysis) of the community composition at the phylum level. (D) Abundance of the horizontal community at the phylum level (n = 6). | |
At the phylum level, AMP administration reduced the increase of Bacteroidetes, but extended the increase in the abundance of Proteobacteria caused by MDR E. coli infection, without influencing the abundance of Firmicutes. In healthy mice, oral intake of AMP decreased the abundances of Firmicutes and Proteobacteria and increased the abundance of Bacteroidetes in the intestinal microflora (Fig. 7D). The decrease in the abundance of Firmicutes in healthy mice and the promotion of Proteobacteria abundance in MDR E. coli infected mice are not favorable for animal health. Overall, AMP treatment did not efficiently repair gut microbiota disorders caused by MDR E. coli infection and exerted adverse effects on the gut microbiota balance of healthy mice.
Therefore, it can be concluded that AMP posed significant limitations and disadvantages with regard to inhibiting MDR E. coli, mainly because it could not inhibit the development of coliform in the gut and exerted undesirable influences that reduced the diversity of intestinal flora and caused intestinal flora disorder (although it exerted anti-inflammatory effects).
All these results were consistent with previous reports that indicated that antibiotics are a very powerful factor causing imbalance of the intestinal microbiota.30 The use of antibiotics can reduce the diversity and abundance of the intestinal flora, thus leading to the reduction of competitive rejection ability, and indirect destruction of the community structure. Consequently, it interferes with the interaction between microbial species and the complementary system of nutrient metabolism pathways, which leads to extensive fluctuations of the intestinal environment.31
3.4. Efficient inhibition of MDR E. coli infection by L. rhamnosus
3.4.1. Effects of L. rhamnosus on body weight, facial bacteria, and inflammatory cytokines in serum.
Before the formal animal experiments, the ability of L. rhamnosus SHA113 to inhibit MDR E. coli infection (1 × 108 cfu mL−1) was determined at different doses of 1 × 106 cfu mL−1, 1 × 107 cfu mL−1, 1 × 108 cfu mL−1 and 1 × 109 cfu m−1. As a result, the dose of 1 × 109 cfu mL−1 showed the most significant inhibitory effects on E. coli infection. Therefore, it was used in the animal experiments.
Fig. 8 shows that, for healthy mice, feeding L. rhamnosus SHA 113 did not significantly influence body weight and serum cytokine levels (P > 0.05), but significantly reduced total coliform and increased LAB in feces. This was consistent with the inhibitory effect of L. rhamnosus SHA 113 on E. coli QBQ 009 in vitro.
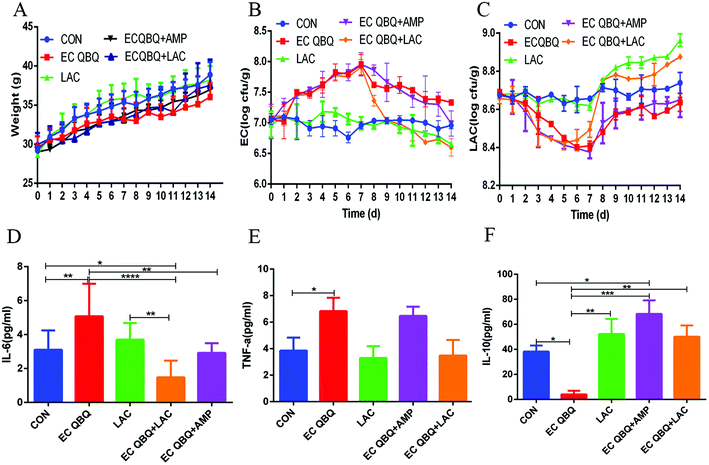 |
| Fig. 8 Effect of L. rhamnosus SHA 113 on body weight, fecal bacteria, and inflammatory cytokines in the serum of MDR E. coli infected mice. (A) Body weight, (B) total coliform in feces, and (C) lactic acid bacteria in feces; (D) IL-6, (E) TNF-α, and (F) IL-10 levels in serum. Data represent mean ± SEM (n = 6), *P < 0.05, **P < 0.01, and ***P < 0.001. | |
It has been proposed that supplementation with probiotics might be a practical way to reduce chemotherapy-induced disorders, and several studies have shown that probiotics exert a protective effect on dysregulation.32 In this study, feeding L. rhamnosus SHA 113 slightly reversed the decrease of body weight, quickly and significantly reversed the increase of total coliform, and decreased LAB in feces caused by E. coli QBQ 009 infection. Feeding L. rhamnosus SHA 113 also efficiently reduced the IL-6 and TNF-α levels and increased the IL-10 levels in the serum of MDR E. coli infected mice, thus showing significant anti-inflammatory effects.
Overall, compared to AMP treatment, feeding L. rhamnosus SHA 113 achieved better efficiency of therapeutic effects with regard to body weight, total coliform, and inflammatory cytokines in serum, bringing these levels closer to those of healthy mice.
In addition, during the experiments, the signs of mental malaise, capillary inversion, slow movement, diarrhea, and even hematochezia after E. coli infection were reversed to a certain extent after AMP administration, but more significantly by L. rhamnosus SHA113. This was consistent with reports on other probiotics, e.g., Saccharomyces boulardii significantly reduced weight loss and diarrhea severity caused by enterotoxigenic E. coli.33 In addition, healthy mice fed with L. rhamnosus SHA113 retained their normal state of mind during the whole experiment.
Furthermore, the correlation coefficients between fecal bacteria and inflammatory cytokines in serum indicated that infection with E. coli (causing an increase of total coliform in feces, EC-FB) induced significant increases of the inflammatory cytokines IL-6 and TNF-α, indicating the occurrence of inflammation. However, feeding L. rhamnosus SHA113 (which caused an increase of LAB in feces, LAC-FB) significantly decreased the TNF-α level in serum, thus showing anti-inflammatory effects (Fig. 9).
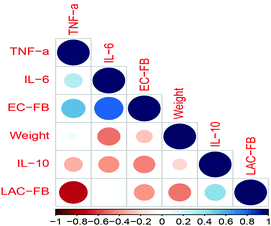 |
| Fig. 9 Correlation between fecal bacteria and inflammatory cytokines in serum. | |
3.4.2. Ability of L. rhamnosus to reverse spleen enlargement caused by E. coli infection.
Table 1 shows that E. coli ATCC 25922 and QBQ 009 infection caused significant increases of the spleen index, more significantly by QBQ 009, indicating that MDR E. coli QBQ 009 infection tended to cause more severe damage to the function and structure of the spleen. Treatment with L. rhamnosus SHA113 could significantly reverse the increase of the spleen index caused by MDR E. coli infection, while AMP could not. Feeding AMP and L. rhamnosus SHA113 to healthy mice caused a slight decrease of the liver index but the effect was not significant. Antimicrobial agents, such as the compound EMTAHDCA, are commonly used to treat splenomegaly in mice by down-regulating the cell cycle, cell metabolism, signaling, transcription, and transporter proteins in the spleen.34 However, the mechanisms with which L. rhamnosus SHA113 cures splenomegaly caused by E. coli infection are still not clear to date.
Table 1 Organ indexes of mice
|
Liver (%) |
Kidney (%) |
Spleen (%) |
Values are expressed as mean ± SD (n = 6). Different letters indicate significant differences in the same column at the level of p < 0.05 (n = 6). |
CON |
4.41 ± 0.21abc |
1.54 ± 0.16 |
0.28 ± 0.03b |
AMP |
4.24 ± 0.06bc |
1.57 ± 0.13 |
0.29 ± 0.04b |
LAC |
4.21 ± 0.42bc |
1.57 ± 0.11 |
0.27 ± 0.09b |
EC 25922 |
4.43 ± 0.18abc |
1.53 ± 0.11 |
0.33 ± 0.05ab |
EC QBQ009 |
4.80 ± 0.43a |
1.58 ± 0.16 |
0.42 ± 0.09a |
EC QBQ + AMP |
4.73 ± 0.32ab |
1.60 ± 0.17 |
0.31 ± 0.078ab |
ECQBQ + LAC |
4.60 ± 0.22abc |
1.68 ± 0.18 |
0.28 ± 0.05b |
The results of E. coli infection effects on organ indexes are consistent with previous reports. It was reported that E. coli infection causes enlargement of the spleen35 and liver injury,36 and even threatened human health. Systemic Salmonella infection was also found to cause prolonged splenomegaly in mice or human hosts.37 Lactic acid bacteria were reported to alleviate liver injury caused by bacterial infection38 and acute toxicity39 to a certain extent. However, this was not found in the present study for L. rhamnosus SHA113.
Furthermore, the livers of healthy mice and those being fed with only L. rhamnosus SHA113 appeared normal with a deep red color, but were obviously lighter and yellow in E. coli QBQ 009 infected mice, indicating functional damage. This could be reversed by treatment with AMP and L. rhamnosus SHA113 (Fig. 10).
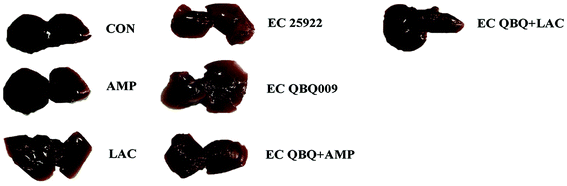 |
| Fig. 10 Livers of mice in different groups. | |
In addition, E. coli QBQ009 infection also increased white blood cells (WBC) and the neutrophil percentage of white blood cells (NE%WBC) in blood. Treatment with AMP and L. rhamnosus SHA113 reversed these changes to near the level of healthy mice, although the effects were not significant. This was consistent with the anti-inflammatory effect of AMP and L. rhamnosus SHA113. No obvious differences were found in other blood cells among different groups (Table 2).
Table 2 Comparison of hematology results of different groups after 14 days of feeding
|
Control |
AMP |
LAC |
EC 25922 |
EC QBQ |
EC QBQ + AMP |
EC QBQ + LAC |
Values are expressed as mean ± SD (n = 6). Abbreviations: WBC, white blood cell; RBC, red blood cell; HGB, hemoglobin concentration; MCV, mean blood cell volume; PLT, platelet count; NE%, neutrophil percentage in WBC; LY%, lymphocyte percentage in WBC; MO%, monocyte percentage in WBC; NE#, absolute value of neutrophils; LY#, lymphocyte absolute value. |
WBC (109 L−1) |
2.93 ± 0.30 |
3.27 ± 0.40 |
3.20 ± 0.81 |
3.05 ± 0.99 |
4.03 ± 0.89 |
4.31 ± 1.24 |
3.31 ± 0.68 |
NE# (109 L−1) |
0.16 ± 0.04 |
0.18 ± 0.09 |
0.18 ± 0.05 |
0.19 ± 0.07 |
0.21 ± 0.07 |
0.19 ± 0.07 |
0.16 ± 0.02 |
LY# (109 L−1) |
2.36 ± 0.25 |
2.59 ± 0.26 |
2.72 ± 0.73 |
1.87 ± 0.41 |
3.12 ± 0.87 |
3.45 ± 1.05 |
2.68 ± 0.60 |
NE (%WBC) |
5.28 ± 1.15 |
6.85 ± 1.45 |
5.60 ± 0.82 |
5.53 ± 1.02 |
6.45 ± 1.94 |
4.66 ± 2.06 |
4.96 ± 1.28 |
LY (%WBC) |
80.66 ± 2.89 |
76.20 ± 5.00 |
84.68 ± 4.09 |
74.4 ± 8.18 |
72.88 ± 6.27 |
79.66 ± 3.95 |
81.16 ± 7.99 |
MO (%WBC) |
0.34 ± 0.20 |
0.25 ± 0.25 |
0.58 ± 0.73 |
0.38 ± 0.32 |
0.43 ± 0.23 |
0.08 ± 0.10 |
0.32 ± 0.22 |
RBC (1012 L−1) |
10.42 ± 0.69 |
10.83 ± 0.12 |
10.21 ± 1.27 |
9.73 ± 2.03 |
10.70 ± 0.78 |
10.24 ± 0.48 |
10.12 ± 0.19 |
MCV (fL) |
52.90 ± 1.35 |
51.97 ± 1.33 |
53.62 ± 1.74 |
51.08 ± 1.23 |
52.28 ± 1.45 |
51.64 ± 1.55 |
52.20 ± 0.96 |
HGB (g L−1) |
154.60 ± 9.22 |
163.67 ± 5.25 |
156.40 ± 18.12 |
142.83 ± 31 |
160.00 ± 11.87 |
152.00 ± 9.53 |
153.40 ± 4.22 |
PLT (109 L−1) |
710.33 ± 103.51 |
699.33 ± 173.94 |
933.75 ± 166.68 |
855 ± 53.24 |
945.50 ± 194.22 |
744.83 ± 127.20 |
873.00 ± 35.92 |
3.4.3. Feeding L. rhamnosus benefits the composition of the gut microbiota.
Fig. 11 shows that for healthy mice, feeding L. rhamnosus SHA113 did not significantly influence the Chao1 and Shannon indexes. However, it caused a slight shift of the community composition of the gut microbiota, greatly increased the abundance of Bacteroidetes, and decreased the abundance of Proteobacteria. This agrees with the results of the detected decrease of total coliform in feces caused by feeding L. rhamnosus. However, the decrease of Firmicutes was not consistent with the increase of lactic acid bacteria detected in feces caused by feeding L. rhamnosus. This might be due to the interaction between increased Bacteroidetes that inhibited bacteria that belong to Firmicutes, which requires further study.
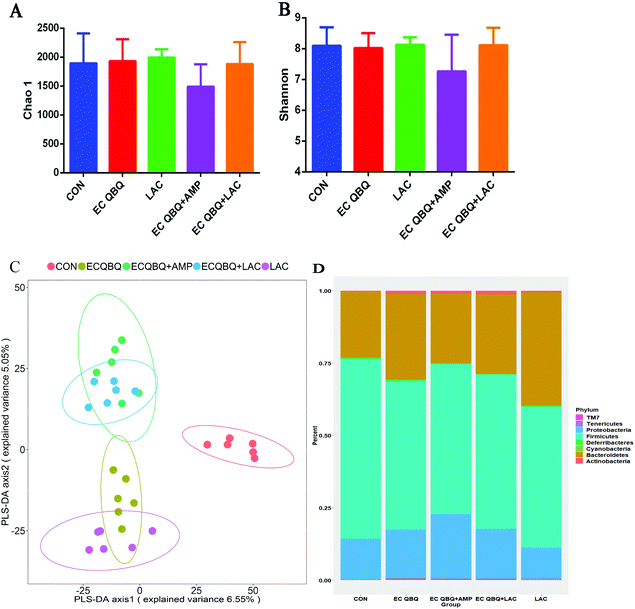 |
| Fig. 11 Effect of L. rhamnosus on the intestinal flora. Alpha diversity of the intestinal microflora. (A) Chao-1 index of mouse cecum microflora. (B) Shannon index of mouse cecum microflora. (C) PLS-DA (Partial Least Squares Discriminant Analysis) of the community composition at the phylum level. (D) Analysis of composition abundance of the horizontal community at the genus level (n = 6). | |
For MDR E. coli infected mice, feeding L. rhamnosus significantly reversed the increase of Bacteroidetes and decrease of Firmicutes caused by MDR E. coli infection. However, AMP treatment caused a decrease in the α-diversity of the intestinal flora and extended the increase in the abundance of Proteobacteria caused by MDR E. coli infection, without influencing the abundance of Firmicutes. This indicated that AMP treatment could not inhibit, but promote pathogen (which mainly belong to Proteobacteria) infection. Treatment with L. rhamnosus SHA 113 significantly cured the pathogen infection and reversed the inflammatory effects almost to the level of healthy mice.
4. Conclusion
According to previous experiments carried out in vitro, some lactic acid bacteria (including Lactobacillus rhamnosus) showed significant antimicrobial activity against multi drug-resistant urinary tract pathogens, including E. coli.40 In our previous reports, other L. rhamnosus strains isolated from human milk also had the ability to inhibit standard E. coli.41 In our preliminary experiments, these strains also showed lower ability to inhibit multi-drug resistant E. coli than SHA113. Therefore, only SHA113 was used in this study. Besides, H. Szajewska and M. Kolodziej reported that Lactobacillus rhamnosus GG is effective for the prevention of antibiotic-associated diarrhoea in children and adults.42 However, this result is different from our results that focused on E. coli infection in the intestinal tract.
AMP-resistant MDR E. coli can cause a similar increase of total coliform and decrease of lactic acid bacteria in feces and more severe immune disorders, inflammation, and intestinal flora disorders than non-AMP-resistant MDR E. coli. AMP could not effectively reduce the abundance of total coliform in feces, but promoted the increase in the abundance of Proteobacteria in the gut microbiota. In contrast, feeding L. rhamnosus SHA113 efficiently and quickly reduced the amount of total coliform, increased the amount of lactic acid bacteria in feces, reversed spleen enlargement and inflammatory effects caused by AMP-resistant MDR E. coli QBQ 009, and increased the abundance of Bacteroidetes in the gut microbiota. Overall, L. rhamnosus SHA113 is more powerful than AMP to cure AMP-resistant MDR E. coli infection and repair imbalances of the gut microbiota.
Conflicts of interest
There are no conflicts to declare.
Acknowledgements
We acknowledge funding by the China Agriculture Research System (CARS-29-jg-3), the Key Research and Development Plan of Shaanxi Province (2017ZDXL-NY-0304, 2019ZDLNY01-02-02), the National Key R&D Program of China (2017YFE0105300), the China Postdoctoral Science Foundation (No. 2017M620471), the Natural Science Foundation of Shaanxi Province (No. 2018JQ3054), and the Postdoctoral Research Project of Shaanxi Province (No. 2017BSHEDZZ103).
References
- K. L. Schwartz and S. K. Morris, Travel and the spread of drug-resistant bacteria, Curr. Infect. Dis. Rep., 2018, 20(9), 29 CrossRef PubMed.
- G. R. Dwivedi,
et al., Synergy of clavine alkaloid ‘chanoclavine’ with tetracycline against multi-drug-resistant E. coli, J. Biomol. Struct. Dyn., 2019, 37(5), 1307–1325 CrossRef CAS PubMed.
- G. R. Dwivedi,
et al., Gallic acid-based indanone derivative interacts synergistically with tetracycline by inhibiting efflux pump in multidrug resistant E. coli, Appl. Microbiol. Biotechnol., 2016, 100(5), 2311–2325 CrossRef CAS PubMed.
- A. Hussain,
et al., Risk of transmission of antimicrobial resistant Escherichia coli from commercial broiler and free-range retail chicken in India, Front. Microbiol., 2017, 8, 2120 CrossRef PubMed.
- P. Agaba,
et al., Nosocomial bacterial infections and their antimicrobial susceptibility patterns among patients in Ugandan intensive care units: a cross sectional study, BMC Res. Notes, 2017, 10(1), 349 CrossRef PubMed.
- A. R. Manges,
et al., Retail meat consumption and the acquisition of antimicrobial resistant Escherichia coli causing urinary tract infections: A case-control study, Foodborne Pathog. Dis., 2007, 4(4), 419–431 CrossRef CAS PubMed.
- S. Kant,
et al., A novel STK1-targeted small-molecule as an “antibiotic resistance breaker” against multidrug-resistant Staphylococcus aureus, Sci. Rep., 2017, 7, 5067 CrossRef PubMed.
- M. Riool,
et al., Controlled release of LL-37-derived synthetic antimicrobial and anti-biofilm peptides SAAP-145 and SAAP-276 prevents experimental biomaterial-associated Staphylococcus aureus infection, Adv. Funct. Mater., 2017, 27(20), 1606623 CrossRef.
- R. Fleeman,
et al., Characterizing the antimicrobial activity of N(2),N(4)-disubstituted quinazoline-2,4-diamines toward multidrug-resistant Acinetobacter baumannii, Antimicrob. Agents Chemother., 2017, 61(6), e0059-17 CrossRef PubMed.
- S. Bandyopadhyay,
et al., Potential antibacterial activity of berberine against multi drug resistant enterovirulent Escherichia coli isolated from yaks (Poephagus grunniens) with haemorrhagic diarrhoea, Asian Pac. J. Trop. Med., 2013, 6(4), 315–319 CrossRef CAS PubMed.
- X. X. Zhao,
et al., Biosynthesis of antibacterial compound against multidrug resistant foodborne pathogens by Phomopsis sp XP-8, Food Control, 2019, 95, 223–231 CrossRef CAS.
- A. Gupta,
et al., Inhibition of adherence of multi-drug resistant E. coli by proanthocyanidin, Urol. Res., 2012, 40(2), 143–150 CrossRef CAS PubMed.
- D. Abedi,
et al., In vitro anti-bacterial and anti-adherence effects of Lactobacillus delbrueckii subsp bulgaricus on Escherichia coli, Results Pharma Sci., 2013, 8(4), 260–268 CAS.
- F. L. Fong,
et al., Mechanism of Action of Probiotic Bacteria on Intestinal and Systemic Immunities and Antigen-Presenting Cells, Int. Rev. Immunol., 2016, 35(3), 179–188 CrossRef CAS PubMed.
- B. Yousefi,
et al., Probiotics importance and their immunomodulatory properties, J. Cell. Physiol., 2019, 234(6), 8008–8018 CrossRef CAS PubMed.
- R. Ashraf and N. P. Shah, Immune system stimulation by probiotic microorganisms, Crit. Rev. Food Sci. Nutr., 2014, 54(7), 938–956 CrossRef CAS PubMed.
- Z. H. Gao,
et al., Inhibitory Effect of Lactic Acid Bacteria on Foodborne Pathogens: A Review, J. Food Prot., 2019, 82(3), 441–453 CrossRef PubMed.
- C. M. C. Chapman,
et al., Comparative in vitro inhibition of urinary tract pathogens by single- and multi-strain probiotics, Eur. J. Nutr., 2013, 52(6), 1669–1677 CrossRef CAS PubMed.
- M. Peng, G. Reichmann and D. Biswas, Lactobacillus casei and its byproducts alter the virulence factors of foodborne bacterial pathogens, J. Funct. Foods, 2015, 15, 418–428 CrossRef CAS.
- H. Szajewska and M. Kolodziej, Systematic review with meta-analysis: Lactobacillus rhamnosus GG in the prevention of antibiotic-associated diarrhoea in children and adults, Aliment. Pharmacol. Ther., 2015, 42(10), 1149–1157 CrossRef CAS PubMed.
- M. Linsalata,
et al., Lactobacillus rhamnosus GG Influences Polyamine Metabolism in HGC-27 Gastric Cancer Cell Line: A Strategy Toward Nutritional Approach to Chemoprevention of Gastric Cancer, Curr. Pharm. Des., 2010, 16(7), 847–853 CrossRef CAS PubMed.
- M. M. Coman,
et al., In vitro evaluation of antimicrobial activity of Lactobacillus rhamnosus IMC 501((R)), Lactobacillus paracasei IMC 502((R)) and SYNBIO((R)) against pathogens, J. Appl. Microbiol., 2014, 117(2), 518–527 CrossRef CAS PubMed.
- T. Setyawardani,
et al., The potential of Lactobacillus rhamnosus and Lactobacillus plantarum isolated from goat's milk in inhibiting Salmonella typhimurium ATCC 14028 infections in rats, Int. Food Res. J., 2017, 24(6), 2625–2631 CAS.
- M. S. Riaz Rajoka,
et al., Identification, characterization, and probiotic potential of Lactobacillus rhamnosus isolated from human milk, LWT–Food Sci. Technol., 2017, 84, 271–280 CrossRef CAS.
- S. Korani,
et al., The effect of human recombinant tumor necrosis factor receptor-2 on reducing inflammatory of collagen-induced arthritis in Balb/c mice, Iran. J. Biotechnol., 2019, 17(1), 30–36 CrossRef PubMed.
- T. Magoc and S. L. Salzberg, FLASH: fast length adjustment of short reads to improve genome assemblies, Bioinformatics, 2011, 27(21), 2957–2963 CrossRef CAS PubMed.
- H. B. Zhao,
et al., Potential of iturins as functional agents: safe, probiotic, and cytotoxic to cancer cells, Food Funct., 2018, 9(11), 5580–5587 RSC.
- A. Ramette, Multivariate analyses in microbial ecology, FEMS Microbiol. Ecol., 2007, 62(2), 142–160 CrossRef CAS PubMed.
- C. C. Tsai, Y. H. Hung and L. C. Chou, Evaluation of lactic acid bacteria on the inhibition of Vibrio parahaemolyticus infection and its application to food systems, Molecules, 2018, 23(5), 1238 CrossRef PubMed.
- A. Langdon, N. Crook and G. Dantas, The effects of antibiotics on the microbiome throughout development and alternative approaches for therapeutic modulation, Genome Med., 2016, 8(1), 39 CrossRef PubMed.
- M. Y. Yoon and S. S. Yoon, Disruption of the Gut Ecosystem by Antibiotics, Yonsei Med. J., 2018, 59(1), 4–12 CrossRef CAS PubMed.
- Y. Wang,
et al., The administration of Escherichia coli Nissle 1917 ameliorates irinotecan-induced intestinal barrier dysfunction and gut microbial dysbiosis in mice, Life Sci., 2019, UNSP116529 CrossRef PubMed.
- J. F. Bisson,
et al., Preventive effects of different probiotic formulations on travelers’ diarrhea model in wistar rats: preventive effects of probiotics on TD, Dig. Dis Sci., 2010, 55(4), 911–919 CrossRef PubMed.
- Niveshika,
et al., Cyanobacterial bioactive compound EMTAHDCA recovers splenomegaly, affects protein profile of E. coli and spleen of lymphoma bearing mice, Mol. Biol. Rep., 2019, 46(3), 2617–2629 CrossRef CAS PubMed.
- L. R. Tomar,
et al., Splenic abscess: Plasmodium vivax with secondary Escherichia coli infection, Trop. Doct., 2015, 45(2), 143–145 CrossRef PubMed.
- X. F. Qiu,
et al., A novel strategy for antimicrobial agent: Role of exogenous carbon monoxide on suppressing Escherichia coli vitality and toxicity, Pak. J. Pharm. Sci., 2015, 28(1), 281–292 CAS.
- A. Jackson,
et al., Innate immune activation during Salmonella infection initiates extramedullary erythropoiesis and splenomegaly, J. Immunol., 2010, 185(10), 6198–6204 CrossRef CAS PubMed.
- S. R. Quilodran-Vega,
et al., Isolation of lactic acid bacteria from swine milk and characterization of potential probiotic strains with antagonistic effects against swine-associated gastrointestinal pathogens, Can. J. Microbiol., 2016, 62(6), 514–524 CrossRef CAS PubMed.
- N. G. Allam,
et al., Protective Efficacy of Streptococcus Thermophilus Against Acute Cadmium Toxicity in Mice, Iran. J. Pharm. Res., 2018, 17(2), 695–707 CAS.
- A. Manzoor,
et al., Efficacy of locally isolated lactic acid bacteria against antibiotic-resistant uropathogens, Jundishapur J. Microbiol., 2016, 9(1), e18952 Search PubMed.
- M. S. R. Rajoka,
et al., Identification, characterization, and probiotic potential of Lactobacillus rhamnosus isolated from human milk, LWT–Food Sci. Technol., 2017, 84, 271–280 CrossRef.
- H. Szajewska and M. Kolodziej, Systematic review with meta-analysis: Lactobacillus rhamnosus GG in the prevention of antibiotic-associated diarrhoea in children and adults, Aliment. Pharmacol. Ther., 2015, 42(10), 1149–1157 CrossRef CAS PubMed.
Footnote |
† These authors contributed equally to this work and should be considered as co-first authors. |
|
This journal is © The Royal Society of Chemistry 2020 |
Click here to see how this site uses Cookies. View our privacy policy here.