DOI:
10.1039/C9FO02238D
(Paper)
Food Funct., 2020,
11, 628-639
Structural analysis and allergenicity assessment of an enzymatically cross-linked bovine α-lactalbumin polymer
Received
24th September 2019
, Accepted 9th November 2019
First published on 9th December 2019
Abstract
Enzymatic cross-linking is frequently used in bio-processing of dairy products since it could change the physiochemical and functional characterization. In our study, bovine α-lactalbumin was cross-linked by polyphenol oxidase from Agaricus bisporus and the changes in the structure, digestibility and allergenicity of α-lactalbumin were explored after cross-linking, and the structural alterations of the polymers were analyzed by circular dichroism spectroscopy, ultraviolet absorption spectroscopy and fluorescence spectroscopy. The digestibility of cross-linked α-lactalbumin was evaluated by simulated digestion in vitro. After that, the allergenicity of α-lactalbumin polymers was evaluated by detection of the specific IgE binding ability using an animal model. The results showed that the secondary and tertiary structures of various α-lactalbumin polymers exhibited a significant variation compared with those of untreated α-lactalbumin, and the cross-linked α-lactalbumin was relatively less susceptible to digestion. Moreover, the allergenicity of cross-linked polymers decreased significantly. These results suggested that there was a direct correlation between a loss of an α-helix and IgE binding to α-lactalbumin, which indicated that enzymatic cross-linking might be an efficient approach to reduce the allergenicity of bovine α-lactalbumin.
1. Introduction
Cow's milk proteins are regarded as a kind of good nutrition for all ages because of their exceptional amino acid profiles.1 However, cow's milk allergy (CMA) is one of the most common causes of food allergy in the world, especially for infants and young children.2,3 Some allergic children would outgrow in the first year of life, but 15% of allergic children still remained allergic.4 CMA is a reaction to cow's milk mediated by IgE and may induce cutaneous, respiratory (rhinitis, asthma, and cough) and gastrointestinal (vomiting, diarrhea, colic, and gastroesophageal reflux) reactions, and even systemic anaphylaxis in some extreme cases.5,6 Several studies on the allergenicity of cow's milk proteins suggested that the main allergenic components in milk are whey proteins and caseins.7 Bovine α-lactalbumin (α-La) accounts for about 25% of whey proteins, and over 75% of CMA patients are sensitive to bovine α-La.8 Therefore, α-La is still a key allergen in milk.
Cross-linking refers to the purpose of increasing the tension and stability of collagen fibers through covalent bonds among the collagen molecules. The enzymes for cross-linking included transglutaminase,9 peroxidase,10 polyphenol oxidase11 and so on. Among them, transglutaminase can improve the specific characteristics of proteins, such as their texture, gel formation, foaming, emulsifying properties and so on.12–16 However, whey proteins may not be easily cross-linked by transglutaminase due to a limited number of binding sites or steric accessibility, while polyphenol oxidase (PPO, EC1.14.18.1) has been widely used to cross-link milk proteins.17–19 PPO, also known as tyrosinase, catalyzes the covalent cross-linking of tyrosine residues9 and is a complex multi-subunit copper-containing oxidoreductase widely distributed in prokaryotes, eukaryotes and fungi.20–22 PPO is generally a complex enzyme, including tyrosinase, laccase, etc., and utilizes phenolic substances in the substrate to oxidize to hydrazine, and then promotes protein cross-linking. Besides improving the food texture, cross-linked food proteins by PPO can beneficially increase digestibility by protease enzymes. The allergenicity of some allergens was decreased by cross-linking with PPO, such as peanut,23 major apple allergen Mal d 124 and β-casein.25 In this sense, the use of PPO was considered as an effective approach to produce hypoallergenic dairy products.
In this work, we aimed to explore the optimal conditions for cross-linking of α-La. Based on that, the structure and allergenicity changes of various polymers from cross-linked α-La were investigated for exploring the relationship of structural alteration and allergenicity.
2. Materials and methods
2.1. Materials
Pepsin from porcine stomach mucosa (Sigma, USA), biotin-labeled goat anti-human IgE (Sigma, USA), o-phenylene diamine (OPD) (Sigma, USA), Coomassie Brilliant Blue R-250 (Solarbio, China), caffeic acid, β-mercaptoethanol, and pancreatin (P7545) were used. Sera from people allergic to cow's milk were acquired from Chongqing Manuik Technology Co. Ltd (Chongqing, China). Other chemical reagents were of analytical grade and were commercially available common reagents.
2.2. Preparation of bovine α-La and polyphenol oxidase
Bovine α-La from raw milk was purified by anion exchange chromatography combined with gel chromatography.26,27 The purity of bovine α-La was assessed by sodium dodecyl sulphate-polyacrylamide gel electrophoresis (SDS-PAGE).
Polyphenol oxidase was isolated from Agaricus bisporus according to our previous study.26 Firstly, 3.0 g of the extracted enzyme powder was dissolved in sodium phosphate buffer (50 mM, pH 7.0) and mixed thoroughly on a vortex shaker and extracted over 60 min at 4 °C. After that, the solution was centrifuged at 8000g for 20 min at 4 °C, and the supernatant was collected as the extracted polyphenol oxidase. The enzyme activity of polyphenol oxidase solution was determined by Gawlik-Dziki's27 method.
2.3. Preparation of cross-linked α-La
As shown in Table 1, different amounts of α-La were dissolved in 1 mL sodium phosphate buffer (50 mM, pH 7.0), respectively. Then the different contents of polyphenol oxidase with 2 mM EDTA and 1 mM caffeic acid were added and mixed. The cross-linking reaction of the mixture was performed for 8 h at 50 °C.
Table 1 Composition and amount of samples for the cross-linking reaction
|
α-La (mg mL−1) |
EDTA (mM) |
Caffeic acid (mM) |
PPO (U mL−1) |
Blank |
5.0 |
2 |
— |
— |
Control |
5.0 |
2 |
1 |
— |
Cross-linking |
1 |
1.0 |
2 |
1 |
1000 |
2 |
2.0 |
3 |
1 |
2000 |
3 |
3.0 |
4 |
1 |
3000 |
4 |
4.0 |
5 |
1 |
4000 |
The cross-linked polymers (dimers, trimers and high polymers) were separated by Native-PAGE with a Mini Protean system (BioRad, USA) using 4% stacking gels and 15% separating gels. The samples were prepared in a loading buffer without SDS and β-mercaptoethanol. The cross-linked polymers (dimers, trimers and polymers) were obtained from gels by cutting the relevant band.
2.4. Spectrum detection of cross-linked α-La
Secondary structures were monitored by using a CD spectropolarimeter (MOS-450/AF-CD, ASCO, Japan). The sample concentration was 0.1 mg mL−1 and each sample was scanned twice. Their secondary structure contents were calculated using JASCO secondary structure software (http://dichroweb.cryst.bbk.ac.uk/html/process.shtml).
UV absorption spectral analysis was performed using an ultraviolet and visible spectrophotometer (TU-1901, Beijing Puxi, China). All the samples were homogeneously mixed and scanned from 280 to 350 nm at room temperature (25 °C).
The unfolding extent of cross-linked α-La polymers was characterized using tryptophan fluorescence with a spectrophotofluorometer (F-4500, Hitachi, Japan). Tryptophan emission was recorded between 300 nm and 400 nm upon excitation at 295 nm with slit widths of 5 nm.
The changes of the surface hydrophobicity of α-La polymers were measured using a 1-anilinonaphthalene-8-sulfonate (ANS) as a hydrophobic fluorescence probe (Sigma, USA). The excitation wavelength was 390 nm, and the emission wavelength was from 400 nm to 650 nm at a scanning speed of 1200 nm min−1.
2.5.
In vitro digestion by a simulated gastric fluid (SGF) and a simulated intestinal fluid (SIF)
A simulated gastric fluid (SGF) and a simulated intestinal fluid (SIF) were prepared according to US Pharmacopeia.28 The SGF in vitro digestion was performed as previously described.29 The digestion solutions included 180 μL of PPO-treated α-La (1 mg mL−1), 60 μL of 0.4 M HCl with 8 g L−1 of NaCl and 6.8 g L−1 of pepsin at pH 1.2. The digestion reaction was carried out at 37 °C with continuous shaking. During the digestion process, an aliquot of the digestion solution (16 μL) was withdrawn at 0 s, 2 min, 5 min, 30 min, 60 min, 120 min and 180 min, respectively. At the same time, the same reaction solutions without pepsin was performed as the control group. Finally the digested products were detected by Tricine-SDS-PAGE.
The SIF buffer was 10 mg mL−1 of pancreatin in 50 mM KH2PO4, pH 7.5. An amount of 60 μL of SIF solution was transferred into a 1.5 mL microcentrifuge tube, followed by incubation for 10 min in a water bath at 37 °C. Then, 180 μL of the α-La control (or PPO-treated α-La) at a concentration of 1 mg mL−1 was added into each microcentrifuge tube for digestion reaction at 37 °C with continuous shaking, followed by collecting an aliquot (18 μL) at 0 s, 2 min, 10 min, 30 min, 60 min, 120 min, 180 min and 240 min, respectively. Then, 4 mM 4-(2-aminoethyl) benzene sulfonyl fluoride hydrochloride (AEBSF) was added to the digestion product for stopping the reaction on ice. Finally, the samples were analyzed using Tricine-SDS-PAGE.
2.6. Detection of human IgE binding capacity to cross-linked α-La
All the experiments for the detection of human sera were performed in compliance with the NCH guidelines from China (NHC Publication No. 11, Rev. 2016) and approved by the Medical Research Ethics Committee from the Second Affiliated Hospital of Nanchang University (Nanchang, China).
Ten specific sera from CMA patients were pooled and used for detection of IgE binding ability. The information about patients is listed in Table 2.
Table 2 Information about CMA patients
Patient no. |
Gender |
Age (months) |
Symptoms |
Specific IgE level (IU ml−1) |
ND – not done.
|
1 |
M |
1 |
Leukocytosis |
17.5–50 |
2 |
M |
10 |
Eczema |
17.5–50 |
3 |
M |
6 |
Eczema |
3.5–17.5 |
4 |
M |
9 |
Serum urticaria |
3.5–17.5 |
5 |
M |
9 |
Eczema |
3.5–17.5 |
6 |
M |
12 |
Asthmatic bronchitis |
2.4 |
7 |
M |
36 |
NDa |
2.1 |
8 |
M |
48 |
NDa |
2.7 |
9 |
M |
11 |
NDa |
1.8 |
10 |
M |
24 |
NDa |
3.7 |
A 96-well plate was coated with 100 μL of purified α-La (5 μg mL−1) and incubated overnight at 4 °C. The plate was washed with PBST and blocked for 1 h at 37 °C. With regard to inhibition, pre-incubation of equal volume (70 μL) diluted sera of patients (1
:
30) and various concentrations of the control (native α-La) or cross-linked polymers was performed for 1 h at 37 °C. A volume of 100 μL of each pre-incubated sample was transferred to each coated well for 1 h at 37 °C. After washing with PBST, 100 μL of biotin-labeled goat anti-human IgE (1
:
5000, KPL, USA) was added to each well for 1 h of incubation at 37 °C. Then a volume of 100 μL of streptavidin conjugated with horseradish peroxidase (1
:
60, Neo Bioscience, China) was added and incubated at 37 °C for 1 h. After further thorough washing of the plates three times again, the color was developed with 100 μL of OPD and H2O2 as enzyme substrates. The reaction was stopped with 50 μL of 2 M H2SO4 and the absorbance was measured at 490 nm using a plate reader (Bio-Rad, USA). As a negative control, PBS instead of sera was added in the well before pre-incubation.
Results were obtained by means of multiple regressions, with the percentage of the binding ratio as the dependent variable and the concentration (log transformed) of the α-La or cross-linked α-La polymer as the predictor variables in the model. The IgE binding rate of α-La or cross-linking α-La polymers was calculated as follows:
Binding rate (%) = (1 − ODsample/OD0) × 100% |
OD
sample: the optical density of antisera with an inhibitor; OD
0: the optical density of antisera without an inhibitor.
2.7. Allergenicity evaluation by immunization of Balb/c mice
Female Balb/c mice (aged 5–6 weeks) were purchased from the Institute for Laboratory Animal Center of Nanchang University (Nanchang, China; Permission number “SYXK(Gan) 2015-0001”). All experiments were performed in strict accordance with the NCH guidelines from China for the care and use of laboratory animals (NHC Publication No.11, Rev. 2016) and approved by the Animal Care Committee of Nanchang University (Nanchang, China).
The mice weighing 17 to 20 g were separated into five groups as shown in Table 3. They were acclimated for 1 week before immunization. In the first sensitization, the mice in groups D and E were intraperitoneally injected with 50 μg native α-La (mixed with complete Freund's adjuvant, USA) and cross-linked polymers (mixed with complete Freund's adjuvant) on day 7, respectively. In the negative control group, the mice in groups A and B were immunized intraperitoneally with normal saline and complete Freund's adjuvant (negative control group) and the injected volume was 0.2 mL for each mouse. At the same time, the mice of group C were immunized with PPO in order to eliminate the effect on enzymes. In a further immunization process, the immunization schedule was identical, and the post-sensitization continued for three times in every week from day 14. Sera were collected on days 14, 21, 28 and 35, and stored at −20 °C until further assays.
Table 3 Immunization protocol for sensitization of Balb/c mice
Group |
Antigen |
Mouse number |
|
A |
0.9%NaCl |
8 |
B |
Adjuvant |
8 |
C |
PPO |
8 |
D |
50 μg ALA |
8 |
E |
50 μg cross-linked protein |
8 |
2.8. Measurement of antigen specific IgE and IgG1 in ELISA
The titers of specific IgE and IgG1 were measured by indirect ELISA. The protocol followed the method of van Esch30 with some modifications. Briefly, 96-well microtiter plates were coated with 0.5 μg α-La in 100 μL carbonate buffer (pH 9.6) overnight at 4 °C. After incubation, the plates were washed three times with PBST (PBS–0.05% Tween) and blocked with 3% gelatin for 1 h at 37 °C. After washing, the sera were diluted to 1
:
20 for detecting IgE and 1
:
2000 for IgG1 and then they were incubated with α-La for 1 h at 37 °C. Diluted horseradish peroxidase-conjugated anti-mouse IgE and IgG1 were added at a ratio of 1
:
6000 and incubated for 1 h at 37 °C. The color was developed with OPD after washing three times. The reaction was stopped with H2SO4, and the absorbance was measured at 490 nm using a microplate reader.
2.9. Plasma histamine assay
Plasma histamine levels were assayed using a histamine immunoassay kit according to the manufacturer's instructions. All assays were performed in duplicate.
2.10. Splenic cell culture and spleen cell proliferation
The mice were sacrificed after thirty minutes of last immunization and the spleens from each group were removed and pooled. Splenocytes were prepared by crushing the spleens, filtering through nylon sieve cells, and pyrolyzing red blood cells under the aseptic conditions. Cells were suspended in complete cell culture medium (RPMI 1640 containing 10% FBS) and centrifuged for 5 min at 2000 rpm. Splenocytes were stimulated with different concentrations of α-La and cross-linked polymers, respectively, and the supernatants were collected after incubation for 72 h.
To each well in the microtitre plate was added 10 μL MTT solution (0.5%, w/v), and then maintained at 37 °C under a humidified atmosphere with 5% CO2 for 4 h. After incubating and centrifuging for 5 min at 2000 rpm, the supernatant from each well was collected and 100 μL DMSO was added to fully dissolve the crystals. The absorbance values were recorded using an ELISA plate reader at 490 nm.
2.11. Determination of the cytokine level
The contents of IFN-γ, IL-4, IL-5 and IL-13 in the culture supernatants were determined by using a cytokine ELISA kit. The cytokine levels were calculated by reference to standard curves of known amounts of IFN-γ, IL-4, IL-5 and IL-13.
2.12. Statistical analysis
Appropriate data were analyzed for statistical significance using ANOVA procedures (SPSS for Windows XP, Microsoft Corp, version 13.0, Chicago, IL, USA) and Fisher's least significant difference (LSD, p < 0.05) test.
3. Results
3.1. Purity of α-La and activity of PPO
The purity of the prepared α-La was analyzed by SDS-PAGE (Fig. 1A). It was found that the purity of the prepared α-La was more than 90% by calculating with software Quantity One, which was purer than that of commercial α-La (Sigma, USA, purity >85%, band 1 in Fig. 1A). The extracted enzyme was lyophilized into powders as shown in Fig. 1B and stored at −20 °C for further analysis. The extracted enzyme powder (5 g) was dissolved in pre-cooled 100 mL phosphate buffer (50 mmol L−1, pH 7.0) as shown in Fig. 1C, and the enzyme activity of the PPO solution was 6000 U mL−1.
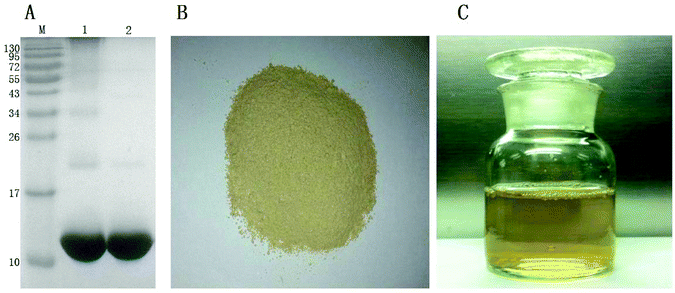 |
| Fig. 1 SDS-PAGE patterns of prepared α-La (A) and prepared PPO (B: PPO powder; and C: PPO solution). Lane M, prestained marker; lane 1, commercial α-La (Sigma, USA, purity >85%). Lane 2, purified α-La; the loading amount of each α-La sample was 40 μg. | |
3.2. Optimum conditions for preparation of cross-linked α-La
The amount of dimers was regarded as an indicator of cross-linking and the result is shown in Fig. 2. There were no obvious differences in the polymer content with the change in the ionic strength ranging from 0.1 to 200 mM (Fig. 2A). As seen in Fig. 2B, the more the enzymes added, the more the polymers with high molecular weights. However, the polymer content did not increase any more when the activity of PPO reached 1000 U, which indicated that the substrate might be exhausted with 1000 U PPO. With respect to the reaction time, it was found that the substrate decreased gradually with the increase in the reaction time within 8 h (Fig. 2C). Regarding pH, it was observed that most polymers were generated at pH 7 (Fig. 2D). It is worth noting that, there was no polymer at low temperature (20 °C), and most polymers were produced at 50 °C (Fig. 2E). As the amount of caffeic acid increased, the contents of polymers increased until they reached 1 mmol ml−1. Based on the above parameters, the optimum conditions for cross-linking were as follows: 1 mM α-La was dissolved in 1 mM PBS with the addition of 1000 U enzyme and 1 mmol ml−1 caffeic acid for 8 h at 50 °C.
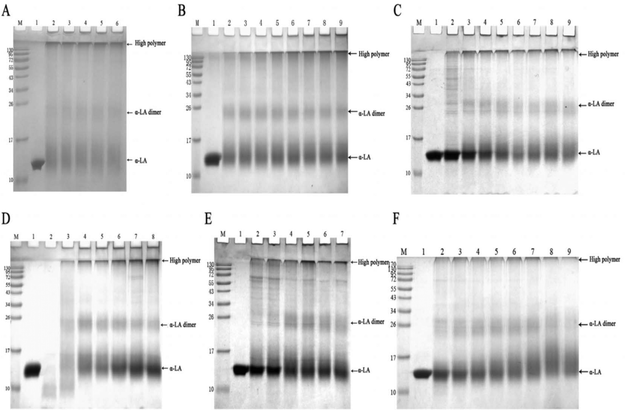 |
| Fig. 2 Cross-linking of α-La with different parameters. M: marker; lane 1: α-La; A: ionic strength: lanes 2–7: 0.1, 10, 50, 100 and 200 mM; B: enzyme activity: lanes 2–7: 100, 200, 400, 600, 800, 1000, 1200 and 1500 U mL−1; C: reaction time; lanes 2–9: 0, 1, 2, 4, 6, 8, 12 and 24 h. D: pH; lanes 2–8: pH 4, 5, 6, 7, 8, 9 and 10; E: reaction temperatures; lanes 2–8: 20, 30, 40, 50, 60 and 70 °C; F: caffeic acid concentrations; lanes 2–9: 0, 0.1, 0.2, 0.5, 1.0, 2.0, 5.0 and 10 mmol mL−1. | |
3.3. Spectrum detection of cross-linked α-La
The secondary structures of cross-linked α-La polymers were analyzed using a far-UV CD spectrum. As shown in Fig. 3A and B, we could find that the spectrum of untreated α-La had a strong positive peak at 192 nm with obvious negative peaks at 208 nm and 222 nm, which showed that the α-helix in the control group was dominant with a percentage of 29.29% followed by random coil (27.96%). While the secondary structure of high polymers formed by cross-linking underwent significant changes, the α-helix decreased to 10% and the β-sheet increased from 20% to 32%; however, the random coil and β-turns showed no obvious changes. Compared with the control, only dimers had the same crossing site with the X axis. The curves of the monomer, trimer and polymer had an obvious shift, which meant that the dimer was the most ordered form and the other polymers had a disordered structure. Therefore, the content of random coils in dimers was the least. The spectrum of the high polymers had almost no positive peak at 192 nm and fluctuated at the specific peaks of the α-helix and β-sheet, and there was more than one intersection with the X-axis. It indicated that the amount of the α-helix in its secondary structure of high polymers was extremely low and the contents of β-sheets and random coils were significantly increased and dominated.
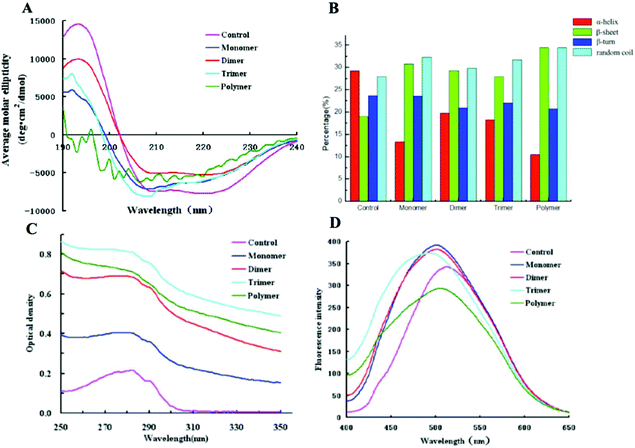 |
| Fig. 3 Spectral detection of cross-linked α-La polymers. A: Far-UV CD; B: secondary structure contents; C: UV spectrum; and D: extrinsic fluorescence. | |
The UV spectrum of the enzymatically cross-linked α-La polymers is shown in Fig. 3C. As we know, all the proteins displayed peaks near 280 nm due to tryptophan (Trp), tyrosine (Tyr) and phenylalanine (Phe). In our study, the maximum absorption peak of the different polymers increased with the degree of cross-linking and the intensity of the maximum absorption peak from the trimer which indicated that cross-linking led to the unfolding of polymer structures since chromophoric groups including Trp, Tyr, and Phe were exposed at the surface of the molecule. However, due to the increasing extent of cross-linking, the polymer may form a more compact spherical structure and some of the chromophoric groups may reembed inside the molecule, which would result in a decline of the absorbance value.
The surface hydrophobicity of the enzymatically cross-linked α-La polymers was determined using ANS-fluorescence as shown in Fig. 3D. Amino acids lysine and arginine interacted with ANS, which could dramatically influence the fluorescence parameters.28 Compared to the control, the λmax of the monomer, dimer and trimer underwent a blue shift of approximately 10–18 nm from 511 nm to 493 nm which indicated that the cross-linking led to more hydrophobic groups of the fluorophores being exposed and the polar groups masked. Interestingly, a red shift and a decrease in the intrinsic fluorescence intensity of λmax were observed in the fluorescence spectra of polymers which suggested that the combination of the ANS and hydrophobic groups inside protein molecules was hampered, resulting in the surface hydrophobicity decrease.
3.4. Digestion stability of different polymers after cross-linking
Simulated gastric digestion in vitro of α-La and its polymers were analyzed by Tricine-SDS-PAGE. As seen in Fig. 4A, the untreated α-La was easily digested with the gastric fluid at 37 °C, and completely degraded into small peptides after 2 h of digestion. Two peptides with molecular weights of about 5 kDa and 3.5–5 kDa are shown in Fig. 4A. Even when the digestion time continues to increase, the contents of these two peptides do not change, indicating that these peptides are too stable to be degraded by proteases. The dimer was also substantially completely degraded after digestion for 1 h and the peptides with a molecular weight of less than 10 kDa and two peptides in the vicinity of 3.5–5 kDa are shown in Fig. 4B. The peptides with a molecular weight of about 5 kDa were not easily digested by pepsin; however, the amount of peptides was gradually reduced when the digestion time prolonged. For the trimer, the situation was totally different since it was stable and no obvious peptides appeared after digestion for 3 h. At the same time, it was found that the band at about 5 kDa also gradually increased with the increasing of the digestion time, and the amount did not change after 2 h digestion, indicating that the trimer was more resistant to gastric digestion. The high polymers formed by cross-linking α-La were digested in vitro with the stimulated gastric fluid, as shown in Fig. 4D. It was found that only a small number of high polymers was degraded after 30 minutes of digestion. Moreover, most of the high polymers were not degraded even after 3 h of digestion.
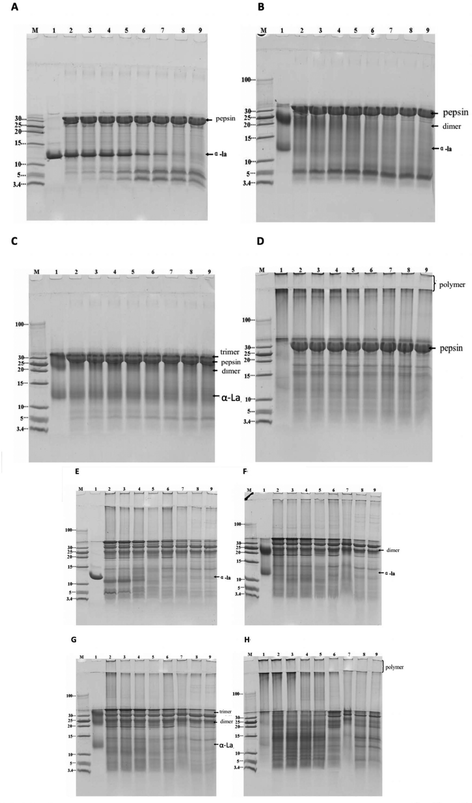 |
| Fig. 4 Tricine-SDS-PAGE result of digested bovine α-La and its cross-linked polymers by simulated gastric and intestinal fluids in vitro. A–D: simulated gastric fluid digestion in vitro; E–F: simulated intestinal fluid digestion in vitro. A and E: untreated α-La; B and F: dimer; C and G: trimer; D and H: polymer; M: marker; lanes 1–9 in A–D: untreated α-La, aliquot that is digested for 0 s, 2 min, 5 min, 10 min, 30 min, 60 min, 120 min and 180 min, respectively; and lanes 1–9 in E–F: untreated α-La, aliquot that is digested for 0 s, 2 min, 10 min, 30 min, 60 min, 120 min, 180 min and 240 min, respectively. | |
Tricine-SDS-PAGE analysis of α-La and its polymers digested by the in vitro simulated intestinal fluid is shown in Fig. 4E–H. From Fig. 4E, it is found that untreated α-La was easily digested by the intestinal fluid. When the sample was mixed with intestinal fluids, hydrolysis occurred at once; after digestion for 30 minutes, it was completely hydrolyzed to peptides with a molecular weight of less than 10 kDa, and large peptides were also hydrolyzed to a smaller peptide or amino acid (less than 3.4 kDa). The results of simulated intestinal fluid digestion in vitro of the dimer and trimer are shown in Fig. 4F and G, respectively. From the change of electrophoretic bands, it was found that the dimers and trimers were resistant to intestinal fluid digestion, and they were gradually hydrolyzed after digestion for 30 min. The dimer was completely degraded after 2 h of digestion, whereas the trimer was completely degraded after 3 h of digestion. Only a slight change occurred after 1 h of digestion of the polymers, but only a small amount of the dimer remained after 4 h of digestion.
3.5. IgE binding capacity of enzymatically cross-linked α-La polymers
The IgE binding ability of cross-linked α-La was evaluated by competitive inhibition ELISA with the sera of CMA patients (Fig. 5). Compared with the control group, the IC50 values for the dimer, trimer and polymer were 19.5 μg mL−1 (14.4 times), 15.14 μg mL−1 (11.2 times), and 3.35 μg mL−1 (2.5 times), respectively. Therefore, the IC50 for the dimer was the highest and that of the polymer was the lowest. This means that the ability of antibody binding to the dimer decreased greatly.
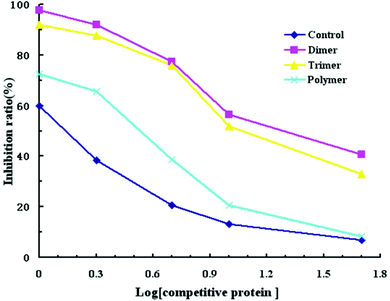 |
| Fig. 5 Human serum IgE-binding to untreated bovine α-La and cross-linked products. | |
3.6. Immune response of sensitized Balb/c mice and histamine levels in mouse plasma
The titer of the IgG antibody was detected by ELISA. As shown in Fig. 6A, the titer of the IgG antibody in the α-La group reached 1 × 105 after immunization. From Fig. 6A, we can find that untreated α-La produced IgG with higher titers compared with the cross-linking group, except for No. 2. It should be noted that untreated proteins induced similar antibody titers in the six groups which indicated that the immunization protocol was accurate and scientific. However, for the cross-linking groups, the titers from different mice were significantly distinguished. Moreover, the titers in the mouse groups (No. 1, 3, 4, 6 and 8) were only one-tenth of that in the mice immunized with untreated protein. Even though the titers were a little higher in the groups of No. 5 and No. 7, they were just two-fifth compared with the untreated group. All these data suggested that IgG titers from cross-linked groups obviously decreased.
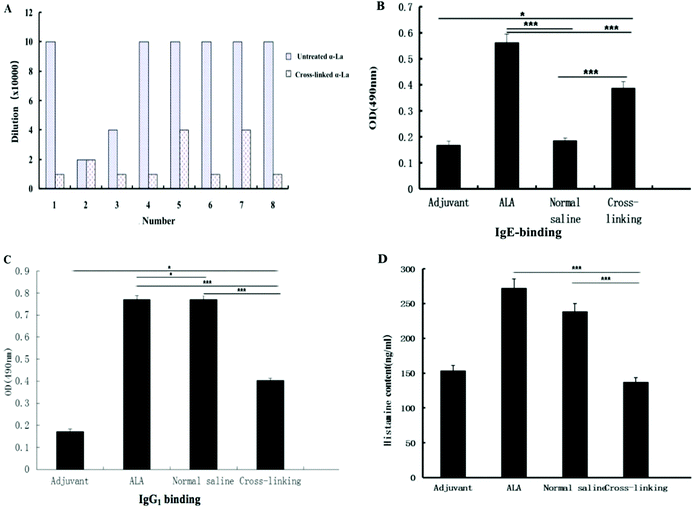 |
| Fig. 6 Specific antibody binding ability and histamine contents of Balb/c mice by immunization of different α-La polymers. A, B and C: IgG, IgE and IgG1 levels; and D: histamine contents. ***: P < 0.01, **: P < 0.05, *: P < 0.1. | |
Furthermore, levels of antigen-specific IgE and IgG1 antibodies were measured by the indirect ELISA. As shown in Fig. 6B and C, the specific IgE level with the same dilution in the cross-linking group was significantly lower (P < 0.01) than that of the untreated α-La group. In the same method, the specific IgG1 level in the cross-linking group was also lower than that of the untreated α-La group with a dilution of 1
:
2000. Moreover, IgE and IgG1 levels in both untreated α-La and cross-linking groups were obviously different from those of the saline control and adjuvant group.
The level of histamine in plasma is an important marker of allergic reaction. As shown in Fig. 6D, the histamine level in the untreated α-La group was significantly higher (P < 0.01) than that of the cross-linking group, which indicated that cross-linking really alleviated the allergic reaction.
3.7. Spleen cell proliferation immunized by bovine α-La and the cross-linking group
As is known, IgE mediated allergic reactions are caused by the imbalance of Th1/Th2, and excessive polarization of Th2 cells can lead to allergic reactions. To explore the effect of polyphenol oxidase on the antigenicity of α-La, the spleen was cultured and stimulated with different concentrations of α-La and the cross-linked product. After 72 h culture, the cell proliferation level was detected as shown in Fig. 7. The levels of spleen cell proliferation were different between untreated α-La and cross-linking groups when the spleen was stimulated by untreated α-La. There was a significant difference (P < 0.01) only in the α-La group when the α-La concentration was 200 μg mL−1, while there were also significant differences (P < 0.01) in both groups when the stimulated α-La concentration was 1000 μg mL−1. Differently, the cell proliferation was obvious in the cross-linking group when the enzyme stimulation concentration was 200 μg mL−1. While the enzyme concentration was 40 μg mL−1, the cell proliferation showed no such apparent change.
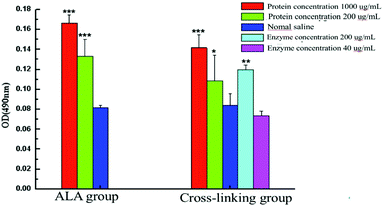 |
| Fig. 7 Proliferation of stimulated spleen cells in vitro after stimulation with different concentrations of the protein and enzyme. ***: P < 0.01, **: P < 0.05, *: P < 0.1. | |
3.8. Effect of cross-linking on the level of cytokines
As shown in Fig. 8, cytokines IL-4, IL-5, IL-13 and IFN-γ related to food allergy were detected after the stimulation of spleen cells in vitro. Compared with the untreated α-La group, the release levels of IL-4 and IL-5 in the cross-linking group were significantly reduced when the stimulated protein concentration was 1000 μg mL−1, while the level of IL-13 notably declined when the concentration was 200 μg mL−1. However, the amounts of IL-4, IL-5 and IL-13 showed no obvious difference when the concentration was 50 μg mL−1. Meanwhile, compared with the saline group, the secreted IL-4, IL-5 and IL-13 were upregulated with all the three concentrations in the α-La group, but only at a concentration of 1000 μg mL−1, the contents of IL-4, IL-5 and IL-13 were significantly increased in the cross-linking group. In addition, the release of IFN-γ showed no obvious change both in untreated α-La and cross-linking groups under three stimulated protein concentrations.
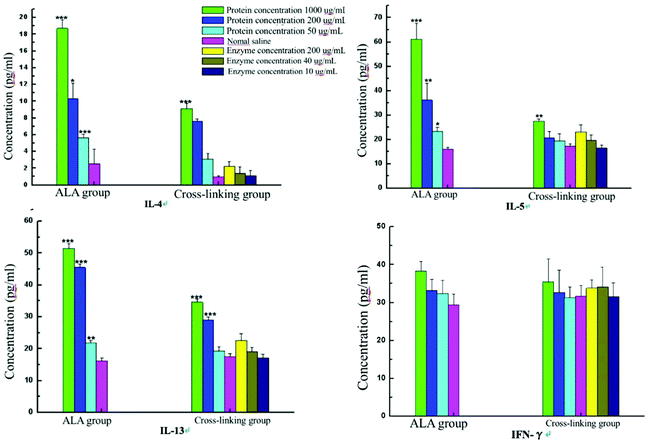 |
| Fig. 8 Cytokine content changes of sera from Balb/c mice after stimulation with different concentrations of the protein and enzyme. | |
4. Discussion
At present, there are roughly the following methods for reducing the antigenicity of bovine α-La and cow's milk: ultraviolet radiation,29 oxidation and heat treatment,31 high-hydrostatic pressure,32 sonication,33 high-intensity pulsed electric field,34 hydrolysis35 and so on. For cross-linking of milk proteins, there are several enzymes including glutaraldehyde,36 graphene oxide nanosheets,37 a zinc oxide nanostructure,38 and horseradish peroxidase39 which are usually given priority for use. Moreover, most of the previous reports mainly focused on foaming properties40 and other physical properties.37,38 Compared with other physically and chemically processed food proteins, enzymatically cross-linked food proteins have attracted much attention in recent years.41,42 The modifications of proteins using enzymatic and chemical reagents have been widely examined and they are powerful strategies for improving the functional properties of macromolecules.43,44 Polyphenol oxidase, also known as tyrosinase, catalyzes the covalent cross-linking of tyrosine residues.9
The polyphenol oxidase crude enzyme solution used in this study is a complex enzyme system containing polyphenol oxidases that catalyze covalent binding. Thus, the SDS-PAGE pattern showed the cross-linked high polymers (Fig. 2). A single factor was explored in this research to optimize polyphenol oxidase-catalyzed cross-linking of α-La, including the reaction time, temperature, pH, enzyme activity and caffeic acid concentration. From Fig. 2, we could find that after the cross-linking reaction under optimal conditions, the high polymers with molecular weights of more than 200 kDa were still at the top of the lane and could not be separated. This shows that the increase of the protein concentration in the reaction system has little effect on the cross-linking reaction. The separation of high polymers should be explored in further study.
In our research, the conformational structure of the enzymatically cross-linked polymers was investigated by CD spectroscopy, UV absorption spectroscopy and fluorescence spectroscopy. Compared with untreated α-La, the secondary structure of the enzymatically cross-linked polymers displayed significant changes. Among them, the secondary structure of the trimer and polymer became more unordered than that of the dimer. Meanwhile, the transfer of the UV spectra from bovine α-La suggested that hydrophobic amino acids of the proteins were partially exposed to an aqueous solvent and the protein structure had partially unfolded. Likewise, the spectra of ANS fluorescence fully illustrated that cross-linking could induce unfolding of α-La and cause the hydrophobic groups and regions inside the molecules to be exposed outside, thus increasing the surface hydrophobicity of the whole proteins. However, due to the high degree of cross-linking, the unfolded polymer was unstable so that it would partially aggregate which allowed the reconstruction of some native conformational epitopes,45 resulting in the surface hydrophobicity and increased sensitization. Unfolding of the protein structure would lead to exposure of linear antigenic epitopes, while the conformational epitopes would be destructed. In this work, compared to untreated α-La, the IgE-binding ability of the enzymatically cross-linked α-La polymers was significantly declined, especially for the dimer and trimer. Due to reaggregation of the unfolded polymer, some conformational epitopes were also generated so that the IC50 of the polymer is lower than those of the dimer and trimer.
With in-depth research on food allergies, peptic stability assessment is also a method of assessing the potential allergenicity and has gradually become an evaluation indicator of food allergens.8 In this study, the gastrointestinal digestion method in vitro proposed by the United States Pharmacopeia was used to evaluate the digestibility of bovine milk α-La after cross-linking. It is well-known that food allergens must first undergo gastrointestinal digestion and it is very important to evaluate the sensitization of the digested peptides. In this experiment, the IgE binding ability of the dimer was significantly reduced after it was digested by gastric and intestinal fluids. The allergenicity of the cross-linked product was lower than that of untreated α-La. This may be the original enzymatic hydrolysis site of polymerization wrapped inside the molecule or involved in the cross-linking reaction and thus affected the digestion reaction. The structure of the trimer also showed unfolding and was loose; so the allergenicity of its digestion product is lower than that of untreated α-La. Moreover, as cross-linking occurs, the steric hindrance becomes stronger, which hinders enzymatic hydrolysis. Due to the compact structure of the polymer, only a small amount of the polymer is digested by the gastric fluid, and many linear epitopes and conformational epitopes still remained; so the residual allergenicity was high. As the digestion time increased, the large molecular peptides that were digested were further degraded to reduce their potential allergenicity. Therefore, the IgE binding capacity of the polymer after simulated intestinal fluid digestion is lower than that after gastric fluid digestion.
Although there are many research studies on protein cross-linking, there are few research studies focused on reducing the allergenicity of proteins. Some of the work in our groups has suggested reduction of the allergenicity of protein after cross-linking issues. For example, in Tong's study, it was demonstrated that caffeic acid-assisted PPO-catalyzed cross-linking significantly reduced the potential allergenicity of ovalbumin but could not eliminate it.46 Furthermore, they found that enzymatic cross-linking may reduce antigenicity and potential allergenicity could be considered as a mediator in the presence of caffeic acid. The changes in modified ovalbumin were likely to be due to some changes in the conformation of ovalbumin.47 In Wu's study, the results suggested that cross-linking could mask allergen epitopes and decrease the allergenicity of Ara h 2.48 In the present study, we used PPO-catalyzed cross-linking of α-La to investigate the relationship of the structure change and allergenicity of α-La. A valid conclusion was reached that the structural changes in the enzymatically cross-linked α-La and reduction of allergenicity were firmly related.
In our work, it is the first time that the enzymatically cross-linked α-La polymers and the relationship between their structure alteration and allergenicity have been explored. PPO could effectively catalyze α-La cross-linking, leading to the formation of the α-La dimer, trimer and polymer. The secondary and tertiary structures of cross-linked α-La polymers were adequately destroyed, and thus the allergenicity decreased obviously. In contrast to untreated α-La, cross-linked α-La was relatively less susceptible to digestion by pepsin and the simulated intestinal fluid and had a lower IgE binding capacity. With respect to different polymers, the enzymatic cross-linking played an important role in reducing the allergenicity of the dimer and trimer. In a word, the structural alterations and allergenicity decline co-existed in the enzymatically cross-linked α-La.
Conflicts of interest
All authors declare no conflict of interest.
Acknowledgements
The work was supported by the National Natural Science Foundation of China (No. 31171716 and 31760431), and Jiangxi Province Funding Program for Outstanding Youth (20162BCB23016).
References
- P. L. Lakshman, S. Tachibana, H. Toyama, T. Taira, T. Suganuma, W. Suntornsuk and M. Yasuda, Application of an acid proteinase from Monascus purpureus to reduce antigenicity of bovine milk whey protein, J. Ind. Microbiol. Biotechnol., 2011, 38, 1485–1492 CrossRef PubMed.
- K. M. Saarinen, K. Juntunen-Backman, A.-L. Järvenpää, P. Kuitunen, L. Lope, M. Renlund, M. Siivola and E. Savilahti, Supplementary feeding in maternity hospitals and the risk of cow's milk allergy: A prospective study of 6,209 infants, J. Allergy Clin. Immunol., 1999, 104, 457–461 CrossRef CAS.
- H. A. Sampson, Part1: Immunopathogenesis and clinical disorders, J. Allergy Clin. Immunol., 1999, 103, 717–728 CrossRef CAS.
- A. Host, Frequency of cow's milk allergy in childhood, Ann. Allergy, Asthma, Immunol., 2002, 89, 33–37 CrossRef.
- L. Monaci, V. Tregoat, A. J. van Hengel and E. Anklam, Milk allergens, their characteristics and their detection in food: A review, Eur. Food Res. Technol., 2006, 223, 149–179 CrossRef CAS.
- J. Sastre, Molecular diagnosis in allergy, Clin. Exp. Allergy, 2010, 40, 1442–1460 CrossRef CAS PubMed.
- G. Bu, Y. Luo, F. Chen, K. Liu and T. Zhu, Milk processing as a tool to reduce cow's milk allergenicity: a mini-review, Dairy Sci. Technol., 2013, 93, 211–223 CrossRef CAS PubMed.
- H. M. Farrell, R. Jimenez-Flores, G. T. Bleck, E. M. Brown, J. E. Butler, L. K. Creamer, C. L. Hicks, C. M. Hollar, K. F. Ng-Kwai-Hang and H. E. Swaisgood, Nomenclature of the proteins of cows’ milk–sixth revision, J. Dairy Sci., 2010, 63, 243–251 Search PubMed.
- C. S. Greenberg, P. J. Birckbichler and R. H. Rice, Transglutaminases: multifunctional cross-linking enzymes that stabilize tissues, FASEB J., 1991, 5, 3071–3077 CrossRef CAS.
- S. C. Fry, Cross-linking of matrix polymers in the growing cell walls of angiosperms, Annu. Rev. Plant Physiol., 1986, 37, 165–186 CrossRef CAS.
- G. MATHEIS and J. R. Whitaker, Modification of proteins by polyphenol oxidase and peroxidase and their products, J. Food Biochem., 1984, 8, 137–162 CrossRef CAS.
- B. O. Kennedy, M. Auty and P. Kelly, Effect of transglutaminase-induced protein cross-linking on the processing and subsequent melting profiles of ice cream, Int. Dairy Fed. [Spec. Issue] S.I., 2004, 414–414 Search PubMed.
- P. C. Lorenzen, Renneting properties of transglutaminase-treated milk, Milchwissenschaft, 2000, 55, 433–437 CAS.
- M. M. O'Sullivan, P. C. Lorenzen, J. E. O'Connell, A. L. Kelly, E. Schlimme and P. F. Fox, Short communication: influence of transglutaminase on the heat stability of milk, J. Dairy Sci., 2001, 84, 1331–1334 CrossRef.
- L. A. Burzio, V. A. Burzio, J. Pardo and L. O. Burzio, In vitro polymerization of mussel polyphenolic proteins catalyzed by mushroom tyrosinase, Comp. Biochem. Physiol., Part B: Biochem. Mol. Biol., 2000, 126, 383–389 CrossRef CAS.
- M. K. Dabbous, Inter-and intramolecular cross-linking in tyrosinase-treated tropocollagen, J. Biol. Chem., 1966, 241, 5307–5312 CAS.
- E. Selinheimo, P. Lampila, M.-L. Mattinen and J. Buchert, Formation of protein– oligosaccharide conjugates by laccase and tyrosinase, J. Agric. Food Chem., 2008, 56, 3118–3128 CrossRef CAS.
- S. Y. Chung, Y. Kato and E. T. Champagne, Polyphenol oxidase/caffeic acid may reduce the allergenic properties of peanut allergens, J. Sci. Food Agric., 2005, 85, 2631–2637 CrossRef CAS.
- A. Garcia, J. H. Wichers and H. J. Wichers, Decrease of the IgE-binding by Mal d 1, the major apple allergen, by means of polyphenol oxidase and peroxidase treatments, Food Chem., 2007, 103, 94–100 CrossRef CAS.
- H. Claus and H. Decker, Bacterial tyrosinases, Syst. Appl. Microbiol., 2006, 29, 3–14 CrossRef CAS PubMed.
- G. Faccio, K. Kruus, M. Saloheimo and L. Thöny-Meyer, Bacterial tyrosinases and their applications, Process Biochem., 2012, 47, 1749–1760 CrossRef CAS.
- S. Halaouli, M. Asther, J. C. Sigoillot, M. Hamdi and A. Lomascolo, Fungal tyrosinases: new prospects in molecular characteristics, bioengineering and biotechnological applications, J. Appl. Microbiol., 2006, 100, 219–232 CrossRef CAS PubMed.
- D. Stanic, E. Monogioudi, E. Dilek, J. Radosavljevic, M. Atanaskovic-Markovic, O. Vuckovic, L. Raija, M. Mattinen, J. Buchert and T. Cirkovic Velickovic, Digestibility and allergenicity assessment of enzymatically cross-linked β-casein, Mol. Nutr. Food Res., 2010, 54, 1273–1284 CrossRef CAS PubMed.
- X. Li, Z. Luo, H. Chen and Y. Cao, Isolation and antigenicity evaluation of β-lactoglobulinfrom buffalo milk, Afr. J. Biotechnol., 2008, 7, 2258–2264 CAS.
- M. Salami, R. Yousefi, M. R. Ehsani, S. H. Razavi, J.-M. Chobert, T. Haertlé, A. A. Saboury, M. S. Atri, A. Niasari-Naslaji and F. Ahmad, Enzymatic digestion and antioxidant activity of the native and molten globule states of camel α-lactalbumin : Possible significance for use in infant formula, Int. Dairy J., 2009, 19, 518–523 CrossRef CAS.
- J. Van Leeuwen and H. J. Wichers, Tyrosinase activity and isoform composition in separate tissues during development of Agaricus bisporus fruit bodies, Mycol. Res., 1999, 103, 413–418 CrossRef CAS.
- U. Gawlik-Dziki, U. Złotek and M. Świeca, Characterization of polyphenol oxidase from butter lettuce (Lactuca sativa var. capitata L.), Food Chem., 2008, 107, 129–135 CrossRef CAS.
- O. K. Gasymov and B. J. Glasgow, ANS fluorescence: potential to augment the identification of the external binding sites of proteins, Biochim. Biophys. Acta, Proteins Proteomics, 2007, 1774, 403–411 CrossRef CAS PubMed.
- V. A. Borzova, K. A. Markossian, K. O. Muranov, N. B. Polyansky, S. Y. Kleymenov and B. I. Kurganov, Quantification of anti-aggregation activity of UV-irradiated α-crystallin, Int. J. Biol. Macromol., 2015, 73, 84–91 CrossRef CAS PubMed.
- B. C. van Esch, K. Knipping, P. Jeurink, S. van der Heide, A. E. Dubois, L. E. Willemsen, J. Garssen and L. M. Knippels, In vivo and in vitro evaluation of the residual allergenicity of partially hydrolysed infant formulas, Toxicol. Lett., 2011, 201, 264–269 CrossRef CAS PubMed.
- A. C. Kramer, A. Torreggiani and M. J. Davies, Effect of Oxidation and Protein Unfolding on Cross-Linking of β-Lactoglobulin and α-Lactalbumin, J. Agric. Food Chem., 2017, 65, 10258–10269 CrossRef CAS PubMed.
- A. J. Trujillo, M. Capellas and J. Saldo,
et al. Applications of high-hydrostatic pressure on milk and dairy products, Innovative Food Sci. Emerging Technol., 2002, 3, 295–307 CrossRef.
- M. J. Taylor and T. Richardson, Antioxidant activity of skim milk: effect of sonication, J. Dairy Sci., 1980, 63, 1938–1942 CrossRef CAS.
- I. Odriozola-Serrano, S. Bendicho-Porta and O. Martin-Belloso, Comparative study on shelf life of whole milk processed by high-intensity pulsed electric field or heat treatment, J. Dairy Sci., 2006, 89, 905–911 CrossRef CAS.
- T. H. Jung, S. S. Yun, W. J. Lee, J. W. Kim, H. K. Ha, M. Yoo, H. J. Hwang, W. M. Jeon and K. S. Han, Hydrolysis by alcalase improves hypoallergenic properties of goat milk protein, Korean J. Food Sci. Anim. Resour., 2016, 36, 516–522 CrossRef PubMed.
- I. J. Arroyo-Maya, H. Hernández-Sánchez, E. Jiménez-Cruz, M. Camarillo-Cadena and A. Hernández-Arana, α-Lactalbumin nanoparticles prepared by desolvation and cross-linking: Structure and stability of the assembled protein, Biophys. Chem., 2014, 193, 27–34 CrossRef PubMed.
- S. Mahanta and S. Paul, Bovine α-lactalbumin functionalized graphene oxide nano-sheet exhibits enhanced biocompatibility: A rational strategy for graphene-based targeted cancer therapy, Colloids Surf., B, 2015, 134, 178–187 CrossRef CAS PubMed.
- S. Mahanta, S. Prathap, D. K. Ban and S. Paul, Protein functionalization of ZnO nanostructure exhibits selective and enhanced toxicity to breast cancer cells through oxidative stress-based cell death mechanism, J. Photochem. Photobiol., B, 2017, 173, 376–388 CrossRef CAS PubMed.
- S. K. Dhayal, S. Sforza, P. A. Wierenga and H. Gruppen, Peroxidase induced oligo-tyrosine cross-links during polymerization of α-lactalbumin, Biochim. Biophys. Acta, Proteins Proteomics, 2015, 1854, 1898–1905 CrossRef CAS PubMed.
- S. K. Dhayal, R. J. Delahaije, R. J. de Vries, H. Gruppen and P. A. Wierenga, Enzymatic cross-linking of α-lactalbumin to produce nanoparticles with increased foam stability, Soft Matter, 2015, 11, 7888–7898 RSC.
- J. A. Gerrard, Protein – protein crosslinking in food: methods, consequences, applications, Trends Food Sci. Technol., 2002, 13, 391–399 CrossRef CAS.
- W. Jin-ju, Emulsify capacity and stability of cross-linked casein with polyphenol oxidase from Agaricus bisporus [in Chinese], Food Sci., 2010, 15 Search PubMed.
- M. J. Bae, H. S. Shin, E. K. Kim, J. Kim and D. H. Shon, Oral administration of chitin and chitosan prevents peanut-induced anaphylaxis in a murine food allergy model, Int. J. Biol. Macromol., 2013, 61, 164–168 CrossRef CAS PubMed.
- N. J. Plundrich, M. Kulis, B. L. White, M. H. Grace, R. Guo, A. W. Burks, J. P. Davis and M. A. Lila, Novel strategy to create hypoallergenic peanut protein–polyphenol edible matrices for oral immunotherapy, J. Agric. Food Chem., 2014, 62, 7010–7021 CrossRef CAS PubMed.
- P. J. Davis and S. C. Williams, Protein modification by thermal processing, Allergy, 1998, 53, 102–105 CrossRef CAS PubMed.
- P. Tong, S. Chen, J. Gao, X. Li, Z. Wu, A. Yang, J. Yuan and H. Chen, Caffeic acid-assisted cross-linking catalyzed by polyphenol oxidase decreases the allergenicity of ovalbumin in a Balb/c mouse model, Food Chem. Toxicol., 2018, 111, 275–283 CrossRef CAS PubMed.
- K. Liu, S. Chen, H. Chen, P. Tong and J. Gao, Cross-linked ovalbumin catalyzed by polyphenol oxidase: Preparation, structure and potential allergenicity, Int. J. Biol. Macromol., 2018, 107, 2057–2064 CrossRef CAS PubMed.
- Z. Wu, J. Lian, Y. Han, N. Zhou, X. Li, A. Yang, P. Tong and H. Chen, Crosslinking of peanut allergen Ara h 2 by polyphenol oxidase: digestibility and potential allergenicity assessment, J. Sci. Food Agric., 2016, 96, 3567–3574 CrossRef CAS PubMed.
|
This journal is © The Royal Society of Chemistry 2020 |
Click here to see how this site uses Cookies. View our privacy policy here.