DOI:
10.1039/C9LC00820A
(Paper)
Lab Chip, 2020,
20, 120-125
Acoustic mixing in a dome-shaped chamber-based SAW (DC-SAW) device†
Received
16th August 2019
, Accepted 8th November 2019
First published on 8th November 2019
Abstract
The use of an open droplet system for surface acoustic wave (SAW)-based applications has been limited by droplet instability at high input power. This study introduces a dome-shaped chamber-based SAW (DC-SAW) device for the first time, which can be fabricated simply using a single adhesive tape and a drop of ultraviolet-curable material without soft lithography processes. The dome-shaped chamber device with a contact angle of 68° enables the maximizing of the effect of SAW transmitted at a refraction angle of roughly 22°, negating the droplet instability. The DC-SAW device was applied to acoustic mixing to estimate its capability. Acoustic mixing of two different fluids (i.e., deionized water and fluorescent particle suspension) was demonstrated in the dome-shaped chamber device. Moreover, the effect of flow rate and applied voltage on mixing performance was estimated. With the decreasing flow rate and increasing applied voltage, mixing performance was enhanced. At an applied voltage of 20 V, mixing indices were higher than 0.9 at a total flow rate of 300 μl min−1.
Introduction
In recent years, surface acoustic wave (SAW) technologies have gained significant attention in bio-chemical analyses and clinical diagnoses.1 Among microfluidic techniques, a SAW-based device has distinctive advantages, including non-invasive control of fluids and particles, high energy efficiency, fast operation, and easy integration of simple electrodes networked with other microfluidic systems.2 Therefore, SAW technologies have been applied to droplet-based microfluidics3–6 and continuous flow-based systems.7–11
When SAW is generated by applying radio frequency (RF) power, acoustic streaming is induced in a droplet, which can be used for various applications, including micromixing,4,12 particle patterning,12 particle concentration,12,13 particle separation,14 droplet jetting,6,15 and droplet atomization.3,16,17 Recently, SAW technologies have been applied to molecular analysis.18,19 However, applications of SAW technologies using the open droplet have limitations. When input power is higher than a critical value, the SAW pressure gradient becomes larger than the surface tension of the droplet. Thus, droplet displacement, jetting, and even evaporation are induced because of droplet instability. This negatively impacts the use of the droplet in various applications. To address the abovementioned limitations of open droplet systems, an enclosed fluid system for particle/cell manipulation in a small volume has been developed.20 However, there is a SAW-less effective area near the chamber wall when SAW refracts into the liquid at the refraction angle, so called ‘Rayleigh angle’.3,7 Owing to the refraction angle, SAW may have low manipulation efficiency near the walls of the enclosed system.
Micromixing has become more important for diverse applications including biochemical researches, chemical syntheses, and clinical diagnoses.12,21–24 However, in microfluidic devices, rapid mixing is difficult to achieve because of low Reynolds numbers (Re). Mixing in a microchannel depends only on molecular diffusion during laminar flow. Therefore, various microfluidic approaches have been developed for efficient homogeneous mixing, which utilizes passive methods, such as twisted,25 folding,26 and wedged channel structures,27 as well as active methods, such as electrokinetic,28,29 magnetic,30 thermal,31 and optical-based mixing.32 Despite the advantages of not using any external forces, passive methods require elaborate channel designs and complex fabrication processes to achieve high mixing efficiency. Meanwhile, via external force actuation, active mixing methods can reduce the mixing time with high mixing efficiency.
Recently, acoustic-based mixing methods have gained much attention because of their advantages of non-invasiveness and simplicity.11,33–42 These techniques utilize a bulk piezoelectric transducer (PZT)-induced chaotic advection or SAW-induced acoustic streaming. However, an oscillating part is required to use the bulk PZT for acoustic mixing, which is limited by special structural design and instability.33–35,43 To enhance the mixing performance in SAW-induced acoustic mixing, only the shape and the configuration of the electrodes are modified.36,37,42 Thus, there is room for improving the performance of SAW-based microfluidic devices by modulating other design factors.
In this study, we report the development of a dome-shaped chamber-based SAW (DC-SAW) device for the first time to utilize SAW effectively by overcoming the previous limitations. The dome-shaped polydimethylsiloxane (PDMS) chamber can be simply fabricated using a single piece of adhesive tape and a drop of ultraviolet (UV)-curable material without soft lithography processes. A contact angle of the dome-shaped chamber was controlled as approximately 68° to maximize the effect of SAW transmitted at a Rayleigh angle of ∼22°. The proposed device is a dome-shaped enclosed system with two inlets and one outlet. This design does not require considering droplet instability, and it enables the utilization of SAW under continuous fluid-flow conditions. The capability of the proposed DC-SAW device was estimated by acoustic mixing of two different fluids, evaluating the effect of the flow rate and applied voltage on mixing performance.
Working principle
A schematic of the proposed DC-SAW device for acoustic mixing is depicted in Fig. 1. The device comprises a piezoelectric substrate (lithium niobate, LiNbO3, 128°, Y-cut, X-propagation, NEL Crystal Co., Fukushima) with patterned focused interdigitated transducers (F-IDT) and a dome-shaped PDMS chamber. The electrode patterned on the piezoelectric substrate is designed as F-IDTs to generate a focused SAW (FSAW) for using the highly concentrated acoustic force.44 The dome-shaped PDMS chamber has two inlets and one outlet. Fluids A and B are injected into two separate inlets via a syringe pump and flow to the FSAW working region. When a radio frequency (RF) signal is applied to the F-IDTs, FSAWs are generated and propagate across the piezoelectric substrate, as shown in Fig. 1(b).
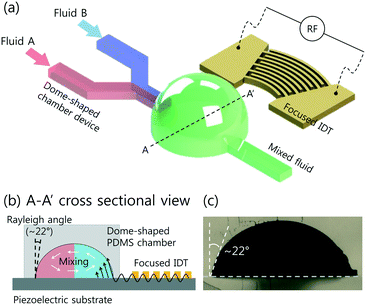 |
| Fig. 1 (a) Schematic of dome-shaped chamber for micromixing using surface acoustic wave. Fluids A and B were injected into the inlets. By applying an RF signal to focused interdigital transducers (IDT) (F-IDTs), the concentrated acoustic energy was generated, and two fluids were mixed in the dome-shaped chamber. (b) Cross sectional image of dome-shaped chamber on the line A–A′ depicted in (a). (c) Cross sectional image of fabricated dome-shaped chamber device for acoustic mixing. | |
A cross-sectional image of the dome-shaped chamber on a black dotted line, A–A′, in Fig. 1(a) is shown in Fig. 1(b). When the FSAWs contact a liquid interface in the dome-shaped chamber during propagation, the FSAW become leakage waves because of the high viscosity of the liquid compared with that of the substrate. The leakage of the FSAW radiation from the substrate is at a Rayleigh angle, which can be defined as:
where
cf and
cs indicate the acoustic wave velocities in the fluid and on the piezoelectric substrate, respectively. Between the lithium niobate wafer substrate and water, the refraction angle can be calculated at ∼22°,
45 as depicted in
Fig. 1(b). Therefore, to remove the SAW-less effective area near the wall
7 and to utilize the acoustic streaming effectively, the contact angle of the dome-shaped PDMS chamber is controlled to reach the desired Rayleigh angle. Therefore, acoustic streaming is induced, resulting in active fluid mixing in the dome-shaped chamber, as depicted by the white arrows in
Fig. 1(b).
Fig. 1(c) shows a microscopic image of the cross section of the fabricated dome-shaped PDMS chamber.
Experimental
Device design and fabrication
The fabrication process for the DC-SAW device for acoustic mixing using photocurable UV epoxy is depicted in Fig. 2. A single adhesive tape with thickness of ∼300 μm is used as the master mold, which is cut using a cutting plotter (CE6000-60, Graphtec). The patterned tape mold is placed in a Petri dish (Fig. 2(a)). To fabricate the dome-shaped chamber, a drop of UV-curable material (NOA61, Norland Optical Adhesive) is deposited on a 3 mm–diameter circular part of the tape mold. Then, the tape-NOA mold is cured under UV exposure (8 W, 365 nm; EA-180, Spectroline, Spectronics, New York) for 2 min for full cross-linking (Fig. 2(b)). A replica molding made of NOA shows good repeatability for mass production.46 The volume of the UV-curable material is determined to achieve a contact angle of 68° for the Rayleigh angle (∼22–23°) (see ESI,† Fig. S1). Based on experimental results, 5 μl of NOA61 was used to achieve the dome-shaped chamber with a diameter of 3 mm and a contact angle of ∼68°. A mixture of the PDMS base (Sylgard 184, Dow Corning, USA) and curing agent (10
:
1 ratio) was poured into the Petri dish (Fig. 2(c)). The mixture was degassed in a vacuum chamber and thermally cured for 90 min at 80 °C in an oven. The cured PDMS mold was peeled from the tape-NOA mold and cut.
 |
| Fig. 2 Fabrication process of the dome-shaped chamber using a UV curable material. (a) A single-adhesive tape-based master mold was placed in a Petri dish. (b) UV-curable material was deposited on the tape mold and cured by UV exposure for 2 min. (c) Uncured PMDS mixture was poured into the Petri dish and thermally cured for 90 min at 80 °C. (d) Fabricated dome-shaped PDMS chamber was irreversibly bonded on a piezoelectric substrate. | |
The F-IDTs have 80 pairs of fingers with a pitch of 25 μm at 10° concentric opening, which were patterned onto a piezoelectric substrate (lithium niobate, LiNbO3, 128°, Y-cut, X-propagation, NEL Crystal Co., Fukushima, Japan). The fabricated dome-shaped chamber was bonded on the F-IDT-patterned substrate using oxygen plasma at 250 W and 80 mTorr for 50 s (CUTE, Femto Science Co., Korea) (Fig. 2(d)).
Experimental procedure
The working frequency for the F-IDTs was set to 39.6 MHz, based on fw = c/λ, where c is the wave propagation speed of the substrate (∼3960 m s−1), and λ is the wavelength (100 μm). The F-IDTs were actuated by a signal generator (AFG3102C, Tektronix) with an amplifier (ZHL-1-2W, Mini-Circuits) and a direct-current power supply (IPS-18B10, VuPOWER).
Two different fluids were injected into two separate inlets using two plastic syringes and a syringe pump (KDS 210, KD Scientific, MA, USA) at flow rates ranging from 50 to 450 μl min−1 at intervals of 50 μl min−1. One fluid stream was deionized (DI) water, and the other was 300 nm fluorescent particles (R300, Thermo Fisher Scientific, CA) suspended in DI water. The mixing performance was observed using an inverted microscope (CKX-41, Olympus) and a fluorescent camera (CS230B, Olympus). During the acoustic mixing process, an air cooling system was used for temperature control of the DC-SAW device during the mixing process47 (see ESI,† Fig. S2).
Results and discussion
To visualize the mixing performance in the DC-SAW device, the DI water and fluorescent particle suspension were injected into two inlets at a fixed flow rate of 50 μl min−1 (Qtotal = 100 μl min−1). Fig. 3 shows the sequential images of mixing behavior in the dome-shaped chamber without and with an applied voltage of 20 Vpp (peak-to-peak voltage) (see ESI† Movie S1). Without input power applied (FSAW off), unmixed two fluids showed a clear interface of laminar flow, as shown in Fig. 3(a). As soon as the input voltage of 20 Vpp was applied to the F-IDTs, the flow of the fluorescent particle suspension showed a 3-dimensional (3D) swirling in the dome-shaped chamber at t = 0 and 30 ms, owing to the focused acoustic force depicted as black dotted arrow in Fig. 3(b) and (c). Finally, at t = 60 ms, the fluorescent streaming reached the opposite sidewall of the dome-shaped chamber, which can achieve the fully mixed condition.
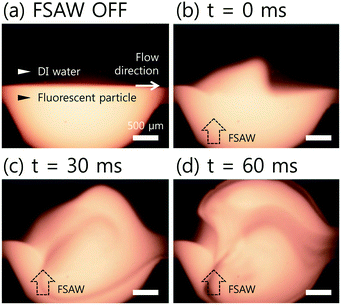 |
| Fig. 3 Time-lapsed images of FSAW-induced acoustic mixing in the dome-shaped PDMS chamber (a) without an applied voltage, and with the applied voltage of 20 Vpp at a fixed flow rate of 50 μl min−1 at (b) t = 0 ms, (c) t = 30 ms, and (d) t = 60 ms, respectively. Black dotted arrow shows FSAW generated from the F-IDTs. | |
For quantitative analysis of the mixing performance, the intensity variation over the pixels after the acoustic mixing was compared to that of the initial, unmixed state using the normalized grayscale values of the experimental images. A mixing index (M) is defined as follows.48
Here, N is the number of pixels over a region of interest (ROI), Ii is the grayscale intensity of the current frame,
is the intensity of the initial frame and Ii,
correspond to the average intensities of the current and initial frame.
Two fully mixed fluids from a vortex mixer (Vortex Genie 2, Scientific Industries Inc., NY, USA) were injected into the DC-SAW device, and the mixing index was calculated by observing the ROI located at 1 mm downstream from the dome-shaped chamber indicated by red dotted line in the inset image in Fig. 4(a) to set the reference value. Additionally, two unmixed fluids with the clear interface of laminar flow was examined. The mixing index, depending on the experimental conditions, such as the applied voltage and flow rate, was then normalized based on the reference value. Therefore, the mixing indices of 0 and 1 indicate the initial, unmixed state and the fully mixed state, respectively.
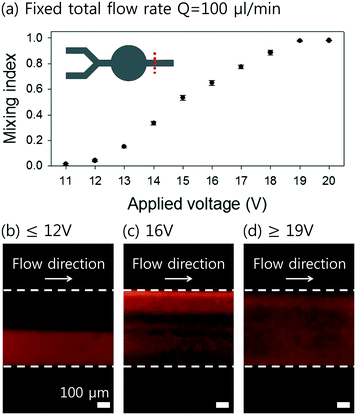 |
| Fig. 4 (a) Effect of the applied voltage on the mixing index for a fixed total flow rate of 100 μl min−1. Red dotted line shows the region of interest (ROI) at 1 mm downstream from the dome-shaped chamber for evaluation of the mixing index. The standard deviation depicts the measured values from five different experiments (n = 5). The fluorescent images at ROI with an applied voltage of (b) 12 Vpp, (c) 16 Vpp, and (d) 19 Vpp, respectively. | |
To evaluate the mixing performance of the DC-SAW device, the effect of the applied voltage was investigated. Fig. 4(a) shows the mixing indices at various applied voltages ranging from 11 to 20 Vpp at an interval of 1 Vpp at the fixed flow rate of 50 μl min−1 (Qtotal = 100 μl min−1). The total power consumption in this voltage ranged from 110 mW for 11 Vpp to 363 mW for 20 Vpp. For the unmixed state at the applied voltage lower than 12 Vpp (V ≤ 12 Vpp), the mixing index was approximately 0 (Fig. 4(b)). As the applied peak-to-peak voltage increased, the mixing index increased, because the acoustic streaming velocity is proportional to the applied input power (vf ∝ P ∝ Vp–p,2 where vf, P, and Vp–p are the acoustic streaming velocity, the RF input power, and the applied peak-to-peak voltage, respectively).37,49 With the applied voltage of 16 Vpp, fluorescent gradient was formed, as shown in Fig. 4(c), of which the mixing index was approximately 0.65. Finally, two fluids were fully mixed in the dome-shaped chamber at 19 Vpp, as shown in Fig. 4(d).
To determine the effect of the flow rate on the mixing performance, the flow rate of each fluid stream was adjusted from 50 to 2500 μl min−1 at an interval of 50 μl min−1. Fig. 5(a) shows the mixing indices at different flow rates having a fixed applied voltage of 20 Vpp. At the flow rates lower than 150 μl min−1 for each flow (Qtotal = 300 μl min−1), successful mixing of two fluids was achieved, and the mixing indices was higher than 0.9 (Fig. 5(b)). As the flow rate becomes higher, the exposure time to the FSAW working region becomes shorter, so that the mixing performance is expected to be degraded. As the flow rate increased to Qtotal = 400 μl min−1, the mixing index decreased to approximately 0.75 (Fig. 5(c)). With the further increase of the total flow rate higher than 500 μl min−1, the mixing index became much lower as 0.58 (Fig. 5(d)).
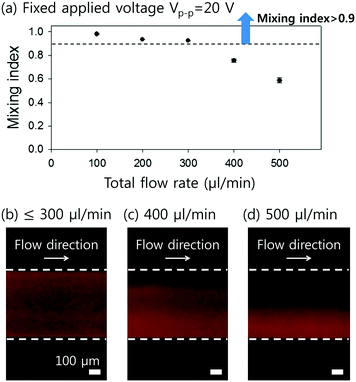 |
| Fig. 5 (a) Effect of the flow rate on the mixing index at a fixed applied voltage of 20 Vpp. The standard deviation depicts the measured values from five different experiments (n = 5). The fluorescent images at ROI at total flow rates of (b) 300 μl min−1, (c) 400 μl min−1, and (d) 500 μl min−1, respectively. | |
To compare the mixing performance with the previous acoustic mixing devices, the mixing index was used depending on the applied voltage or the device throughput, due to the differences in IDT configurations and SAW actuation conditions. With the similar applied voltages to our optimal condition (20 Vpp), the previous acoustic mixing devices showed the mixing indices of 0.95 at Qtotal = 100 μl min−1 and 21 Vpp (ref. 50) and 0.95 at Qtotal = 120 μl min−1 and 18 Vpp.51 On the other hand, at the similar throughput to our experimental condition (100 μl min−1), the previous works showed lower mixing indices with much higher applied voltages: 0.9 at Qtotal = 166 μl min−1 and 80 Vpp (ref. 37) and 0.15 at Qtotal = 100 μl min−1 and 85 Vpp.36 The DC-SAW device could achieve an improved mixing performance with a mixing index of 0.98 (98% of mixing efficiency) at a higher throughput (Qtotal = 100 μl min−1) and a lower applied voltage of 20 Vpp owing to maximized use of the SAW transmitted at the Rayleigh angle.
The current results show the capability of the DC-SAW device for acoustic mixing as a proof-of-concept. The mixing index can be further enhanced by optimizing the dimension and the volume of the dome-shaped chamber, instead of 5 μl of UV-curable material to fabricate the 3 mm–diameter dome-shaped chamber. Meanwhile, as a future work, fabrication of master molds for dome-shaped chamber with varying dimensions is in progress using 3D-printing techniques, which has recently drawn much attention because of its advantages of rapid, low-cost fabrication of complex and arbitrary geometries.52–54 In addition, as a newly developed device, the DC-SAW device requires additional parametric studies to characterize and optimize the device on the contact angle of the chamber (see ESI,† Fig. S3), the configurations of IDTs (interdigitated transducers), the location of FSAW focal point, and so on.
Conclusions
In summary, we demonstrated the first use of a DC-SAW device considering a SAW transmission at the refractive angle ‘Rayleigh angle’ at which the acoustic wave propagates from the substrate to the liquid. Using a low-cost fabrication process of a single adhesive tape and a UV-curable material without soft lithography, the DC-SAW device was simply fabricated. For validation of the DC-SAW device, acoustic mixing was conducted using flow streams of DI water and a fluorescent particle suspension. As the flow rate decreased and the applied voltage increased, the mixing performance was enhanced, resulting in a higher mixing index. With an applied voltage of 20 V, the mixing index was greater than 0.9 at a total flow rate of Q ≤ 300 μl min−1, which showed improved mixing performance compared to previous acoustic-based mixing devices.
Author contributions
Conceptualization, J. N.; methodology, J. N.; validation, J. N., H. L., and S. M. B.; formal analysis, J. N. and H. L.; investigation, H. L., and S. M. B.; data curation, J. N. and H. L.; writing—original draft preparation, J. N. and H. L.; writing—review and editing, J. N.; visualization, J. N. and H. L.; supervision, J. N. and H. C.; project administration, J. N. and H. C.; funding acquisition, J. N. and H. C.
Conflicts of interest
There are no conflicts to declare.
Acknowledgements
This research was supported by the Basic Science Research Program through the National Research Foundation of Korea (NRF) funded by the Ministry of Education (2017R1D1A1A09000962) and by a grant of the Establish R&D Platform Project through the Korea University Medical Center and Korea University Guro Hospital, funded by the Korea University Guro Hospital (O1903851).
References
- L. Y. Yeo and J. R. Friend, Annu. Rev. Fluid Mech., 2014, 46, 379–406 CrossRef.
- T. Dung Luong and N. Trung Nguyen, Micro Nanosyst., 2010, 2, 217–225 CrossRef.
- M. Alvarez, J. R. Friend and L. Y. Yeo, Langmuir, 2008, 24, 10629–10632 CrossRef CAS.
- T. Frommelt, M. Kostur, M. Wenzel-Schäfer, P. Talkner, P. Hänggi and A. Wixforth, Phys. Rev. Lett., 2008, 100, 034502 CrossRef.
- H. Li, J. R. Friend and L. Y. Yeo, Biomed. Microdevices, 2007, 9, 647–656 CrossRef.
- M. K. Tan, J. R. Friend and L. Y. Yeo, Phys. Rev. Lett., 2009, 103, 024501 CrossRef.
- J. Nam, Y. Lee and S. Shin, Microfluid. Nanofluid., 2011, 11, 317–326 CrossRef CAS.
- J. Nam, H. Lim, D. Kim and S. Shin, Lab Chip, 2011, 11, 3361–3364 RSC.
- J. Shi, H. Huang, Z. Stratton, Y. Huang and T. J. Huang, Lab Chip, 2009, 9, 3354–3359 RSC.
- J. Shi, X. Mao, D. Ahmed, A. Colletti and T. J. Huang, Lab Chip, 2008, 8, 221–223 RSC.
- W.-K. Tseng, J.-L. Lin, W.-C. Sung, S.-H. Chen and G.-B. Lee, J. Micromech. Microeng., 2006, 16, 539 CrossRef CAS.
- R. Shilton, M. K. Tan, L. Y. Yeo and J. R. Friend, J. Appl. Phys., 2008, 104, 014910 CrossRef.
- G. Destgeer, H. Cho, B. H. Ha, J. H. Jung, J. Park and H. J. Sung, Lab Chip, 2016, 16, 660–667 RSC.
- G. Destgeer, J. H. Jung, J. Park, H. Ahmed and H. J. Sung, Anal. Chem., 2016, 89, 736–744 CrossRef.
- J. O. Castro, S. Ramesan, A. R. Rezk and L. Y. Yeo, Soft Matter, 2018, 14, 5721–5727 RSC.
- D. Ashtiani, H. Venugopal, M. Belousoff, B. Spicer, J. Mak, A. Neild and A. de Marco, J. Struct. Biol., 2018, 203, 94–101 CrossRef CAS.
- J. Reboud, R. Wilson, Y. Zhang, M. H. Ismail, Y. Bourquin and J. M. Cooper, Lab Chip, 2012, 12, 1268–1273 RSC.
- G. Xu, R. N. Gunson, J. M. Cooper and J. Reboud, Chem. Commun., 2015, 51, 2589–2592 RSC.
- Z. Guttenberg, H. Müller, H. Habermüller, A. Geisbauer, J. Pipper, J. Felbel, M. Kielpinski, J. Scriba and A. Wixforth, Lab Chip, 2005, 5, 308–317 RSC.
- L. Meng, F. Cai, Q. Jin, L. Niu, C. Jiang, Z. Wang, J. Wu and H. Zheng, Sens. Actuators, B, 2011, 160, 1599–1605 CrossRef CAS.
- V. Hessel, H. Löwe and F. Schönfeld, Chem. Eng. Sci., 2005, 60, 2479–2501 CrossRef CAS.
- J. W. Hong, V. Studer, G. Hang, W. F. Anderson and S. R. Quake, Nat. Biotechnol., 2004, 22, 435 CrossRef CAS.
- G. Schabas, H. Yusuf, M. G. Moffitt and D. Sinton, Langmuir, 2008, 24, 637–643 CrossRef CAS.
- S. Yang, F. Guo, B. Kiraly, X. Mao, M. Lu, K. W. Leong and T. J. Huang, Lab Chip, 2012, 12, 2097–2102 RSC.
- S. Hardt, H. Pennemann and F. Schönfeld, Microfluid. Nanofluid., 2006, 2, 237–248 CrossRef CAS.
- Z. Chen, M. Bown, B. O'Sullivan, J. MacInnes, R. Allen, M. Mulder, M. Blom and R. van't Oever, Microfluid. Nanofluid., 2009, 6, 763–774 CrossRef CAS.
- W. Buchegger, C. Wagner, B. Lendl, M. Kraft and M. J. Vellekoop, Microfluid. Nanofluid., 2011, 10, 889–897 CrossRef CAS.
- C.-C. Cho, Microfluid. Nanofluid., 2008, 5, 785–793 CrossRef.
- E. Choi, K. Kwon, S. J. Lee, D. Kim and J. Park, Lab Chip, 2015, 15, 1794–1798 RSC.
- Y. Wang, J. Zhe, B. T. Chung and P. Dutta, Microfluid. Nanofluid., 2008, 4, 375–389 CrossRef.
- B. Xu, T. N. Wong, N.-T. Nguyen, Z. Che and J. C. K. Chai, Biomicrofluidics, 2010, 4, 044102 CrossRef.
- A. N. Hellman, K. R. Rau, H. H. Yoon, S. Bae, J. F. Palmer, K. S. Phillips, N. L. Allbritton and V. Venugopalan, Anal. Chem., 2007, 79, 4484–4492 CrossRef CAS.
- D. Ahmed, X. Mao, B. K. Juluri and T. J. Huang, Microfluid. Nanofluid., 2009, 7, 727 CrossRef CAS.
- D. Ahmed, X. Mao, J. Shi, B. K. Juluri and T. J. Huang, Lab Chip, 2009, 9, 2738–2741 RSC.
- P.-H. Huang, Y. Xie, D. Ahmed, J. Rufo, N. Nama, Y. Chen, C. Y. Chan and T. J. Huang, Lab Chip, 2013, 13, 3847–3852 RSC.
- M. C. Jo and R. Guldiken, Sens. Actuators, A, 2013, 196, 1–7 CrossRef CAS.
- T.-D. Luong, V.-N. Phan and N.-T. Nguyen, Microfluid. Nanofluid., 2011, 10, 619–625 CrossRef CAS.
- X. Mao, B. K. Juluri, M. I. Lapsley, Z. S. Stratton and T. J. Huang, Microfluid. Nanofluid., 2010, 8, 139 CrossRef CAS.
- A. Ozcelik, D. Ahmed, Y. Xie, N. Nama, Z. Qu, A. A. Nawaz and T. J. Huang, Anal. Chem., 2014, 86, 5083–5088 CrossRef CAS.
- Z. Yang, S. Matsumoto, H. Goto, M. Matsumoto and R. Maeda, Sens. Actuators, A, 2001, 93, 266–272 CrossRef CAS.
- K. Yasuda, Sens. Actuators, B, 2000, 64, 128–135 CrossRef CAS.
- Q. Zeng, F. Guo, L. Yao, H. Zhu, L. Zheng, Z. Guo, W. Liu, Y. Chen, S. Guo and X. Zhao, Sens. Actuators, B, 2011, 160, 1552–1556 CrossRef CAS.
- P.-H. Huang, M. Ian Lapsley, D. Ahmed, Y. Chen, L. Wang and T. Jun Huang, Appl. Phys. Lett., 2012, 101, 141101 CrossRef PubMed.
- T.-T. Wu, H.-T. Tang, Y.-Y. Chen and P.-L. Liu, IEEE Trans. Ultrason. Ferroelectr. Freq. Control, 2005, 52, 1384–1392 Search PubMed.
- X. Ding, P. Li, S.-C. S. Lin, Z. S. Stratton, N. Nama, F. Guo, D. Slotcavage, X. Mao, J. Shi and F. Costanzo, Lab Chip, 2013, 13, 3626–3649 RSC.
- J. H. Sim, H. J. Moon, Y. H. Roh, H. W. Jung and K. W. Bong, Korean J. Chem. Eng., 2017, 34, 1495–1499 CrossRef CAS.
- A. Urbansky, F. Olm, S. Scheding, T. Laurell and A. Lenshof, Lab Chip, 2019, 19, 1406 RSC.
- Y. Zhang, C. Devendran, C. Lupton, A. De Marco and A. Neild, Lab Chip, 2019, 19, 262–271 RSC.
- N.-T. Nguyen and R. M. White, IEEE Trans. Ultrason. Ferroelectr. Freq. Control, 2000, 47, 1463–1471 CAS.
- J. Nam, W. S. Jang and C. S. Lim, Sens. Actuators, B, 2018, 258, 991–997 CrossRef CAS.
- J. Nam and C. S. Lim, Sens. Actuators, B, 2018, 255, 3434–3440 CrossRef CAS.
- C. Chen, B. T. Mehl, A. S. Munshi, A. D. Townsend, D. M. Spence and R. S. Martin, Anal. Methods, 2016, 8, 6005–6012 RSC.
- Y. Hwang, O. H. Paydar and R. N. Candler, Sens. Actuators, A, 2015, 226, 137–142 CrossRef CAS.
- S. Waheed, J. M. Cabot, N. P. Macdonald, T. Lewis, R. M. Guijt, B. Paull and M. C. Breadmore, Lab Chip, 2016, 16, 1993–2013 RSC.
Footnote |
† Electronic supplementary information (ESI) available. See DOI: 10.1039/c9lc00820a |
|
This journal is © The Royal Society of Chemistry 2020 |
Click here to see how this site uses Cookies. View our privacy policy here.