DOI:
10.1039/D0MO00015A
(Research Article)
Mol. Omics, 2020,
16, 327-338
Pan-transcriptomic analysis identified common differentially expressed genes of Acinetobacter baumannii in response to polymyxin treatments†
Received
26th January 2020
, Accepted 22nd May 2020
First published on 22nd May 2020
Abstract
Multidrug-resistant Acinetobacter baumannii is a top-priority Gram-negative pathogen and polymyxins are a last-line therapeutic option. Previous systems pharmacological studies examining polymyxin killing and resistance usually focused on individual strains, and the derived knowledge could be limited by strain-specific genomic context. In this study, we examined the gene expression of five A. baumannii strains (34654, 1207552, 1428368, 1457504 and ATCC 19606) to determine the common differentially expressed genes in response to polymyxin treatments. A pan-genome containing 6061 genes was identified for 89 A. baumannii genomes from RefSeq database which included the five strains examined in this study; 2822 of the 6061 genes constituted the core genome. After 2 mg L−1 or 0.75 × MIC polymyxin treatments for 15 min, 41 genes were commonly up-regulated, including those involved in membrane biogenesis and homeostasis, lipoprotein and phospholipid trafficking, efflux pump and poly-N-acetylglucosamine biosynthesis; six genes were commonly down-regulated, three of which were related to fatty acid biosynthesis. Additionally, comparison of the gene expression at 15 and 60 min in ATCC 19606 revealed that polymyxin treatment resulted in a rapid change in amino acid metabolism at 15 min and perturbations on envelope biogenesis at both time points. This is the first pan-transcriptomic study for polymyxin-treated A. baumannii and our results identified that the remodelled outer membrane, up-regulated efflux pumps and down-regulated fatty acid biosynthesis might be essential for early responses to polymyxins in A. baumannii. Our findings provide important mechanistic insights into bacterial responses to polymyxin killing and may facilitate the optimisation of polymyxin therapy against this problematic ‘superbug’.
Introduction
Over the past two decades, multidrug-resistant (MDR) Acinetobacter baumannii has become a serious threat to human health worldwide.1,2 In 2017, the World Health Organization prioritised A. baumannii as a ‘Critical’ pathogen that urgently requires novel antimicrobial therapies.3A. baumannii can rapidly acquire antibiotic resistance via either horizontal gene transfer or spontaneous mutations.4–6 Three major international clones I–III were identified in A. baumannii clinical isolates.7,8 Previous studies suggested that A. baumannii had an ‘open’ pan-genome and displayed a remarkable genomic plasticity, which potentially contributes to its ability of surviving adverse conditions, including antibiotic treatments.8
Polymyxins are a group of cationic lipopolypeptide antibiotics first discovered in the 1940s. They are increasingly used as a last-line defence against Gram-negative ‘superbugs’ over the last decade and only polymyxin B and colistin (i.e. polymyxin E) are available in the clinic.9–12 Polymyxin B and colistin differ only at position 6 (D-phenylalanine for polymyxin B, D-leucine for colistin) and have almost identical minimum inhibitory concentrations (MICs).13 The known antimicrobial mechanism of polymyxins involves the initial electrostatic and the subsequent hydrophobic interactions with lipid A of lipopolysaccharide (LPS) in the bacterial outer membrane, followed by cell envelope disorganisation and eventually cell death.13 Generation of hydroxyl radicals and inhibition of membrane-bound type II NADH–quinone oxidoreductase may also contribute to polymyxin killing.14,15 However, the detailed mechanism of polymyxin activity remains unclear. A. baumannii can develop resistance to polymyxins by modifying lipid A with positively charged phosphoethanolamine (pmrC or eptA) and D-galactosamine (naxD), or LPS loss,16–22 which reduces the overall negative charges at the cell surface and attenuates the initial electrostatic interactions with polymyxin molecules.
Transcriptomics was employed to investigate antibacterial killing and resistance in A. baumannii.17,23,24 Previous transcriptomic studies revealed significant changes in gene expression levels due to polymyxin treatments;17,23,24 however, these changes could be a result of strain-specific responses, and a holistic analysis at species level is thus much required. Here we reported the first pan-transcriptomic study of bacterial responses to polymyxins in A. baumannii and identified a set of commonly perturbed genes involved in cellular stress responses, lipid transport and metabolism and cell membrane biogenesis. These findings will facilitate future optimisation of polymyxin therapy against MDR A. baumannii.
Materials and methods
Data acquisition and pre-processing
RNA-Seq data were retrieved from Gene Expression Omnibus (GEO) database (Table 1)25 and the raw reads were downloaded from NCBI Sequence Read Archive (SRA) database.26 Draft genome assembly of each strain was obtained from NCBI Assembly database,27 and employed as a reference for read alignment using Subread.28 Read counts were summarised by featureCounts.29
Table 1 GEO RNA-Seq data of A. baumannii isolates treated with polymyxins
Accession |
Strain |
Treatment |
Replicate |
Ref. |
MIC was not clarified in the original datasets.
—: No reference was published for this dataset.
|
GSE56218 |
34654 |
Polymyxin B (0.75 × MICa)/control 15 min |
2 : 2 |
—b |
GSE56219 |
478810 |
Polymyxin B (0.75 × MICa)/control 15 min |
2 : 2 |
— |
GSE56220 |
983759 |
Polymyxin B (0.75 × MICa)/control 15 min |
2 : 2 |
— |
GSE56221 |
1000160 |
Polymyxin B (0.75 × MICa)/control 15 min |
2 : 2 |
— |
GSE56222 |
1207552 |
Polymyxin B (0.75 × MICa)/control 15 min |
2 : 2 |
— |
GSE56223 |
1428368 |
Polymyxin B (0.75 × MICa)/control 15 min |
2 : 2 |
— |
GSE56224 |
1457504 |
Polymyxin B (0.75 × MICa)/control 15 min |
2 : 2 |
— |
GSE56225 |
1564232 |
Polymyxin B (0.75 × MICa)/control 15 min |
2 : 2 |
— |
GSE56226 |
1592897 |
Polymyxin B (0.75 × MICa)/control 15 min |
2 : 2 |
— |
GSE62794 |
ATCC 19606 |
Colistin (0.2 mg L−1, 60 min)/colistin (2 mg L−1, 15 min)/colistin (2 mg L−1, 60 min)/control (15 min)/control (60 min) |
2 : 2 : 2 : 2 : 2 : 2 |
17
|
Strain typing, pan-genome analysis and phylogeny inference
The draft assemblies of the five strains (Table 1) were uploaded to PubMLST (https://pubmlst.org/bigsdb?db=pubmlst_abaumannii_isolates) to determine their sequence types.30,31 The core genome of the five selected strains and additional 84 strains with gapless genomes was identified by Roary, with amino acid sequence identity of ≥ 95% and carriage of ≥ 99% across A. baumannii genomes (Table 1 and Table S1, ESI†).32 A maximum-likelihood tree was constructed by RAxML33 using general time reversible (GTR) gamma model with 100 bootstrap replicates, and was then visualised by iTOL.34
Identification of differentially expressed genes (DEGs)
The edgeR package35 was used to identify DEGs, with Benjamini–Hochberg false discovery rate (FDR) adjusted P-value < 0.01 and fold change (FC) ≥ 2 (i.e. log2
FC ≤ −1 or ≥ 1).36 Heatmaps were generated by ComplexHeatmap package37 and Venn diagrams were created using VennDiagram package.38 All packages were installed and used in R 3.6.2.
Enrichment using clusters of orthologous groups (COGs)
COG classifications of each A. baumannii strain were predicted by eggNOG.39 Functional enrichment was conducted using a hypergeometric distribution model.40 The probability (P) of observing at least x genes in a COG category containing m genes by chance was determined by the cumulative hypergeometric distribution model below
where k represents the number of DEGs from n genes in a dataset.
Results
Data collection and strain verification
In total, there were 10 RNA-Seq datasets of A. baumannii strains treated with polymyxins for 15 min in GEO database (Table 1) thus far; GSE62794 was from our previous study17 and the other nine datasets were from PRJNA234525. In the present study, we focused on transcriptomic responses in polymyxin-susceptible wild-type strains. The strains in GSE31206 were polymyxin-resistant mutants18 and those in GSE10796419 and GSE10995141 were collected from patients who received colistin therapies and were polymyxin-resistant; hence, GSE31206, GSE107964 and GSE109951 were excluded in the present study. Our group previously reported another transcriptomics dataset for strain AB307-0294 using an in vitro pharmacokinetic/pharmacodynamic model;24 this dataset was not included here because dynamic polymyxin exposure was used and the results cannot be compared to those with static polymyxin treatments as described above. In dataset GSE62794, A. baumannii ATCC 19606 (colistin MIC = 1 mg L−1) was treated with colistin at 0.2 mg L−1 (0.2 × MIC) for 60 min, and 2 mg L−1 (2 × MIC) for 15 and 60 min. In the other nine datasets (Table 2), each isolate was treated with polymyxin B at 0.75 × MIC for 15 min. Among the nine isolates, only 4 (34654, 1207552, 1428368 and 1457504) were A. baumannii (Table 2) and belonged to Sequence Type 2 (ST2, international clone II). Strain ATCC 19606 was classified as ST52 but not assigned an international clone lineage.
Table 2 Species identification for 9 Acinetobacter strains by 16S rRNA BLAST and MLST
Accession |
Strain |
Verification (16S rRNA BLAST)a |
Verification (MLST) |
Used in this study |
BLASTn, identity ≥ 95%, coverage ≥ 95%, E-value ≤ 1 × 10−10.
|
GSE56218 |
34654 |
A. baumannii
|
A. baumannii
|
Yes |
GSE56219 |
478810 |
Acinetobacter sp. strain HS2YWS12 |
A. pittii
|
No |
GSE56220 |
983759 |
A. radioresistens strain a2 |
|
No |
GSE56221 |
1000160 |
A. baumannii
|
|
No |
GSE56222 |
1207552 |
A. baumannii
|
A. baumannii
|
Yes |
GSE56223 |
1428368 |
A. baumannii
|
A. baumannii
|
Yes |
GSE56224 |
1457504 |
A. baumannii
|
A. baumannii
|
Yes |
GSE56225 |
1564232 |
A. pittii
|
|
No |
GSE56226 |
1592897 |
A. nosocomialis
|
A. nosocomialis
|
No |
Phylogenetic analysis and core genome analysis
An A. baumannii pan-genome consisting of 6061 genes was identified in the selected five strains (Table 1) and the other 84 strains with gapless genomes. A core set of 1738 genes were identified and accounted for 42.6–50% of the 89 genomes. A maximum-likelihood phylogenetic tree was constructed based on the core-genome alignment (Fig. 1). Strains 34654 and 1457504 were closely related, ATCC 19606 and 1428368 were in a same clade, and 1207552 was distantly related to the other four strains. Overall, 2,822 genes were commonly present in all five strains (Fig. 2), accounting for 68.6–75.3% of each genome. These common genes were involved in a range of functions including amino acid transport and metabolism (221 genes), transcription (220 genes), inorganic ion transport and metabolism (183 genes), energy production (176 genes), translation, ribosome biogenesis (155 genes), cell envelope biogenesis (143 genes), and lipid transport and metabolism (136 genes) (Fig. 3). In addition, 3239 accessory genes (obtained by subtracting the core-genome from the pan-genome) were identified, including those related to replication, recombination and repair, cell envelope biogenesis, and transcription (Fig. S1, ESI†).
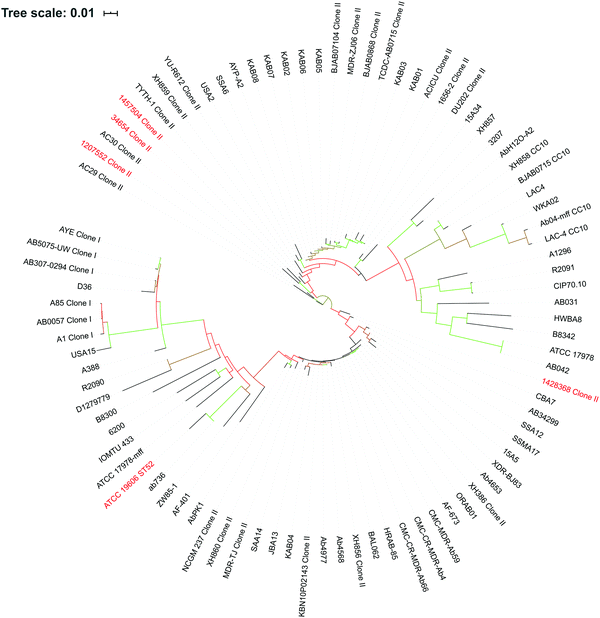 |
| Fig. 1 Phylogenetic tree of 89 A. baumannii strains. The five strains examined in this study are highlighted in red. International clones (ICs) are indicated following strain names. The bootstrap values are indicated by the colour of branches, which ranges from red (bootstrap = 10) to green (bootstrap = 100). | |
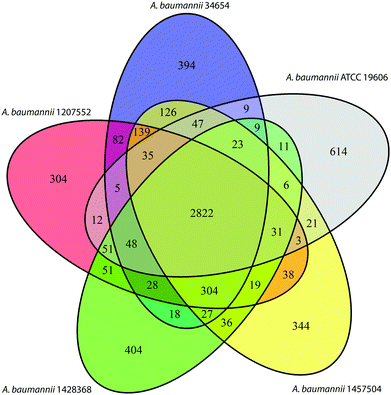 |
| Fig. 2 Numbers of common and unique genes in the five A. baumannii strains. | |
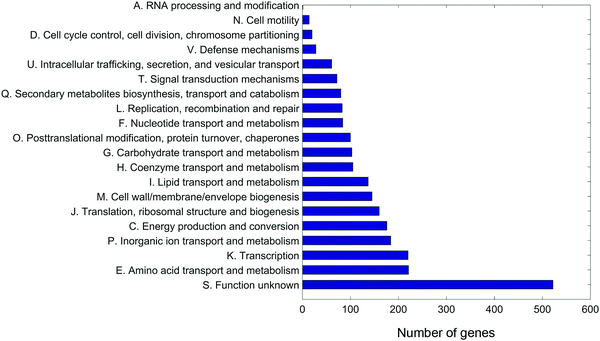 |
| Fig. 3 Functional classifications of the common genes in five A. baumannii strains. | |
Differentially expressed genes in response to 15 min polymyxin treatments
COG analysis on the expression changes of the 2822 common genes showed that several categories were significantly perturbed by polymyxin treatments (2 mg L−1 colistin or 0.75 × MIC polymyxin B) at 15 min, including lipid transport and metabolism (I), cell envelop biogenesis (M), cell motility (N), intracellular trafficking, secretion and vesicular transport (U), and defence mechanisms (V) (Fig. 4). In all five strains under polymyxin treatments, fatty acid biosynthesis genes (fabBDGIZ and accABCD) were significantly down-regulated; the genes involved in lipoprotein trafficking (lolABD), efflux pumps and poly-N-acetylglucosamine biosynthesis (pgaAC) were also remarkably up-regulated. Specifically, in strain 1457504 the expression levels of the genes involved in pili assembly and twitching motility were significantly decreased under polymyxin treatment.
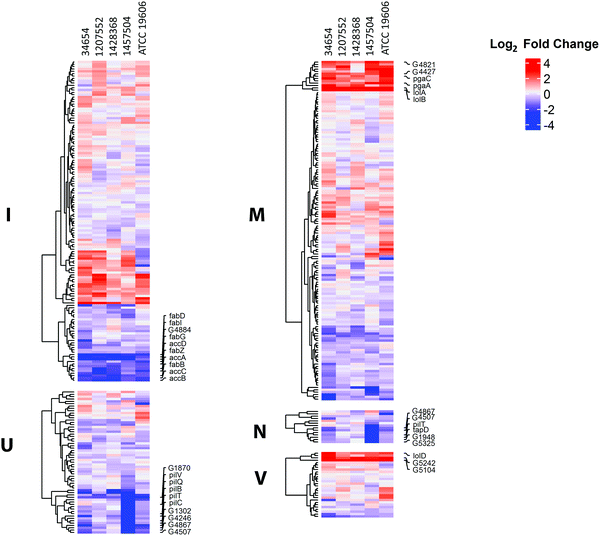 |
| Fig. 4 Gene expression changes of the common genes in five A. baumannii strains following polymyxin treatments for 15 min. I: Lipid transport and metabolism; M: Cell envelop biogenesis; N: Cell motility; U: Intracellular trafficking, secretion, and vesicular transport; V: Defence mechanisms. | |
Interestingly, only 47 common DEGs were identified across all five strains under 2 mg L−1 or 0.75 × MIC polymyxin treatments for 15 min, representing a relatively small core set of polymyxin regulome in A. baumannii. Among these genes, 41 were up-regulated and 6 were down-regulated (Fig. 5 and 6). The commonly up-regulated DEGs included the genes associated with cell envelope biogenesis, lipid transport and metabolism, and signal transduction. With a combination of less stringent thresholds (i.e. FDR < 0.05 and FC ≥ 1.5), 88 common DEGs were identified across the five strains; among them, 69 were commonly increased and 17 were commonly down-regulated. Compared to the 47 DEGs identified with stringent thresholds (i.e. FDR < 0.01 and FC ≥ 2), 41 DEGs were uniquely identified (Table S2, ESI†), which included the genes involved in efflux pumps (G2078 and arcD) and fatty acid biosynthesis (fabB).
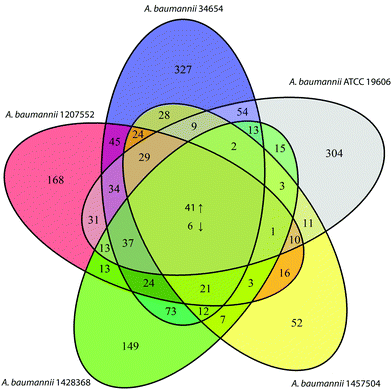 |
| Fig. 5 Numbers of differentially expressed genes commonly or uniquely identified in the five A. baumannii strains. | |
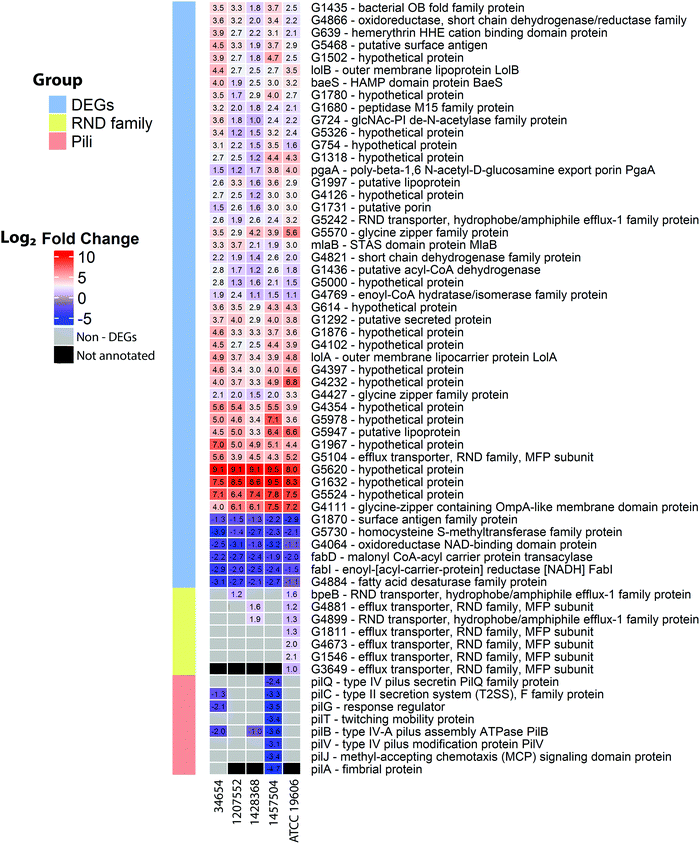 |
| Fig. 6 Heatmap of common DEGs across the five strains, as well as RND family genes and pili genes. Grey denotes non-DEGs and black denotes the genes not detected in a specific strain. Log2 fold changes are shown by the heatmap and labelled in each cell. | |
The bacterial outer membrane constitutes the frontline of defence against external adverse conditions, such as polymyxin treatments. Under polymyxin treatments, genes lolA and lolB in Lol lipoprotein trafficking system showed 10.4–30.6 and 5.6–20.8 fold higher expression in all five strains compared to untreated control, respectively; whereas lolD was up-regulated by 2.4–6.1 fold in all strains except strain 1457504 (Fig. 7). In addition, mlaB, a component of phospholipid retrograding system that maintains the outer membrane phospholipid asymmetry,42 was up-regulated by 3.9–12.9 fold following polymyxin treatments in all 5 strains (Fig. 7).
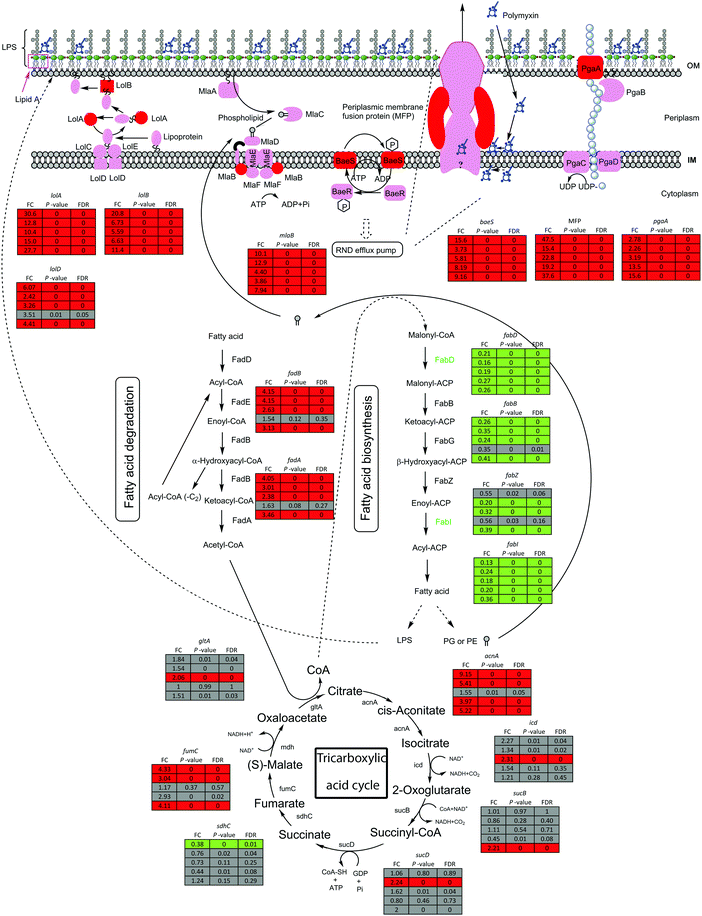 |
| Fig. 7 Polymyxin-induced transcriptomic perturbations in A. baumannii. Proteins in red are encoded by the genes that exhibited increased expression in response to polymyxin treatments in all five strains and proteins in green show decreased expression in the five strains. Fold-change values for A. baumannii 34654, 1207552, 1428368, 1457504 and ATCC 19606 are denoted next to the protein. Grey box indicates non-DEGs. OM, outer membrane; IM, inner membrane; PG, phosphatidylglycerols; PE, phosphatidylethanolamine. Solid arrows indicate the direct transformations or transportations, and dashed arrows denote the indirect transformations or transportations. | |
A two-component regulatory system responds to changes in the environment and consists of a sensor kinase and its cognate response regulator.43 In all strains, the sensor gene baeS of BaeSR two-component system was significantly up-regulated by 3.7–15.6 fold after 15 min polymyxin treatments (Fig. 7). The BaeSR system regulates the expression of RND efflux pumps,44 up-regulation of baeS could result in an enhanced expression of these genes. Indeed, the genes (G5104 and G5242, identified by pan-genome analysis, Table S3, ESI†) encoding RND transporters showed significantly increased expression across all five strains (Fig. 7).45–47 Notably, seven RND family genes (bpeB, G4881, G4899, G1811, G4673, G1546 and G3649, Table S3, ESI†) were up-regulated exclusively in ATCC 19606 (Fig. 6).
Fatty acids are essential components of bacterial membranes.48 Polymyxin treatments perturbed fatty acid biosynthesis in most strains. The expression of fabD (malonyl-CoA:ACP transacylase) and fabI (enoyl-ACP reductase) was slightly reduced following 15 min polymyxin treatments in all five strains. β-oxidation genes fadA and fadB were up-regulated by 2.4–4.1 and 2.6–4.2 fold, respectively, in four strains except 1457504. The accABCD operon encodes acetyl-CoA carboxylase (ACC) which commits the first step of fatty acid biosynthesis.49 With polymyxin treatments (2 mg L−1 colistin or 0.75 × MIC polymyxin B), accB and accC were down-regulated by 3.2–4.5 and 3.0–4.1 fold in four strains (except 1457504), respectively; accD was down-regulated in strains 1428368 and ATCC 19606 by 2.5 and 2.6 fold, respectively; accA was down-regulated in strains 1207552, 1428368 and ATCC 19606 by 3.3–4.3 fold.
Biofilm formation in A. baumannii is associated with exopolysaccharide production, of which poly-β-1,6-N-acetyl-D-glucosamine (PNAG) is crucial for biofilm formation.50 The expression of PNAG synthesis genes was increased after 15 min of polymyxin treatment in all five strains. In particular, pgaA (encoding the outer membrane porin) was up-regulated by 2.3–15.6 fold, while pgaB (encoding the poly-β-1,6-N-acetyl-D-glucosamine [PGA] N-deacetylase outer membrane export lipoprotein) was up-regulated only in 3 strains (34654, 1428368 and ATCC 19606). pgaC and pgaD encode the inner membrane proteins; pgaC was significantly increased by 2.2–10.6 fold in 4 strains except 1457504, and pgaD was increased by 3.0–9.4 fold in strains 1428368 and ATCC 19606. Pilus-mediated biofilm formation is regarded as an important virulence factor in A. baumannii.51 The csuA/BABCDE chaperone–usher pili assembly system is involved in the adherence of A. baumannii biofilm to abiotic surface.52 Gene csuC was significantly down-regulated only in strain ATCC 19606. A. baumannii twitching mobility depends on the repetitive extension and retraction of type IV pili.53 Notably, pilA exclusively exists in strains 34654 and 1457504, while pilBCFGJTQV are in all five strains. Interestingly, genes pilA and pilBCFGJTQV were significantly down-regulated only in A. baumannii 1457504 (Fig. 6). Together, the down-regulated gene expression above indicates an inhibition of pili biogenesis and bacterial mobility under polymyxin treatments.
The tricarboxylic acid (TCA) cycle generates redox equivalents.54 The expression level of the key genes (acnA, icd, sucB, sucD, fumC and gltA) was significantly increased in at least one strain, indicating an enhanced generation of redox equivalents via TCA cycle to cope with the potential oxidative damage induced by the polymyxin treatments (Fig. 7).
Differential gene expression caused by polymyxin treatment at 15 min versus 60 min
Bacteria may respond to polymyxin treatment in a different manner at 15 and 60 min. Indeed, following treatment with 2 mg L−1 colistin, the expression of 613 and 480 genes were significantly changed in strain ATCC 19606 at 15 and 60 min, respectively, and 350 genes were in common. COG enrichment analysis revealed that at 15 min DEGs were overrepresented in amino acid transport and metabolism, whereas at 60 min DEGs were overrepresented in cell envelope biogenesis.
Within the common DEGs, genes from the following categories were significantly over-represented at both time points: lipid transport and metabolism, cell envelope biogenesis, post-translational modification, protein turnover, chaperones and defence mechanisms (P < 0.01, cumulative hypergeometric distribution model). A consistent expression pattern of these genes was observed, highlighting their importance in response to polymyxin treatment in A. baumannii. Specifically, the expression of genes in cell envelope biogenesis (M) was changed (Tables S4–S6, ESI†) at both 15 and 60 min. The expression of glmU, the gene responsible for producing UDP-N-acetyl-α-D-glucosamine from D-glucosamine 1-phosphate, was significantly increased at 15 and 60 min; while gene glmS, encoding glucosamine-6-phosphate synthase that initiates the amino sugar synthesis,55 showed increased expression at 60 min only. The gene yaeT, which encodes a conserved integral membrane protein and is responsible for the assembly of integral β-barrel proteins,56,57 showed increased expression between 2.0- and 2.3-fold during the treatment at both 15 min to 60 min. Interestingly, at 60 min the gene yfiO, which encodes the only essential lipoprotein component of the YaeT complex in outer membrane,58 was up-regulated by 2.4 fold and might play an important role in stabilising the outer membrane.
Discussion
A comprehensive understanding of the mechanisms of antibiotic action and resistance is crucial for optimisation of antimicrobial chemotherapy. Transcriptomic studies were conducted to identify the gene expression changes in response to polymyxin treatments.59 However, the transcriptomic responses to polymyxin killing could vary substantially in A. baumannii due to significant genetic divergence between strains and different treatments (e.g. concentration and time). Therefore, we conducted the first pan-transcriptomic study in A. baumannii to identify common DEGs in five strains in response to polymyxin treatments; these DEGs are involved in dramatic cell membrane re-modelling, perturbed fatty acid metabolism and increased expression of RND efflux pumps (Fig. 6 and 7). Three major international clones were reported in A. baumannii and were commonly associated with multidrug resistance and nosocomial outbreaks globally.7,60,61 Significant differences in transcriptomic responses to polymyxin treatments were identified between lineages (e.g. down-regulated expression of RND transporter genes in ST52 strain ATCC 19606 but not in international clone II strains) and within the lineage (e.g. down-regulated expression of type IV pili genes in strain 1457504 but not in other international clone II strains). pilA determines different twitching motility and biofilm formation in A. baumannii strains ACICU, BIDMC57 and AB507562 and displays remarkable sequence divergence among A. baumannii strains.63 The pilA gene was found only in strains 34654 and 1457504, and its expression following polymyxin treatments decreased only in strain 1457504, possibly due to the polymyxin concentration examined. mrkD is part of the chaperone–usher system which assembles type 3 fimbrial adhesin for adherence to collagen-coated surfaces.64 In the five strains in this study, mrkD was present only in strain 1457504. The genome divergence above implies the potential discrepancies in the colonisation and motility among A. baumannii strains.
Overall, 2822 genes were identified in five A. baumannii strains. Among them, the expression of fabBDGIZ, accABCD, lolAB and pgaAC was commonly changed following polymyxin treatment (Fig. 4). Discrepancies in transcriptomic responses across the five strains could be a result of different experimental designs. For example, colistin was used against ATCC 19606 and polymyxin B was applied to the other four strains, and their concentrations were also different (Table 1). Therefore, the fold change values for the same genes from different studies cannot be directly compared; however, the similar trends in the gene expression profiles provide valuable mechanistic information on A. baumannii responses to polymyxins.
Polymyxins may exert time-dependent effects in altering gene expression. Time-course comparison of the responses of ATCC 19606 to polymyxin treatment for 15 min and 60 min was previously reported,17 and the gene ontology (GO) terms enriched from common DEG lists were presented for both time points, such as protein transportation, membrane assembly and fatty acid β-oxidation pathway. In order to provide a systematic overview of the functional perturbations over 60 min, in the present study we examined the time effect of polymyxin treatment on transcriptomic changes in A. baumannii using COG. The COG enrichment showed that amino acid transport and metabolism genes were rapidly perturbed at 15 min, while the expression of cell envelope biosynthesis genes was perturbed continuously up to 60 min. Our time-dependent transcriptomic studies in Klebsiella pneumoniae65 and A. baumannii24 indicated that 15 min treatment mainly resulted in differential expression of genes associated with bacterial stress responses to polymyxin treatment, including two-component regulatory system BaeSR. At both 15 and 60 min following colistin treatment, 350 genes were differentially expressed possibly as a response to the outer membrane damage caused by polymyxins. These DEGs included those from lipid transport and metabolism, cell envelope biogenesis, post-translational modification, protein turnover, chaperones and defence mechanism.
We discovered that several key genes in the outer membrane biogenesis were commonly up-regulated in all five strains, including mlaB, lolABD, baeSR, pgaABCD and TCA genes. The Mla system is responsible for phospholipid trafficking and plays a key role in maintaining outer membrane asymmetry by transporting phospholipids to the inner membrane.66 The increased expression of mlaB indicates that the polymyxin treatments might induce dramatic perturbation in the outer membrane (Fig. 7) and bacterial cells tried to repair the damaged outer membrane. In Gram-negative bacteria, Lol system contributes to lipoproteins transfer across the inner membrane to the outer membrane.67 The increased expression of Lol genes following polymyxin treatment suggests an increased demand of lipoproteins to stabilise the outer membrane. In the BaeSR system, BaeS senses the signal of cell envelope stresses and activates BaeR which in turn up-regulates the expression of RND efflux pump genes.45,68 Indeed, across all five strains, significant up-regulation of BaeSR-regulating RND family genes was observed after 15 min exposure to polymyxins, indicating an important role of the BaeSR system in the rapid response to polymyxin treatments. The pgaABCD operon is responsible for synthesis and transport of the biofilm-associated exopolysaccharide PNAG,50,69 and the increased expression of pga operon genes also suggests that the biofilm formation in A. baumannii was triggered to help bacteria survive polymyxin treatments. The TCA cycle is a major source of NADH and starts with acetyl-CoA, the building-block metabolites for lipid biosynthesis70 (Fig. 7). Interestingly, six genes encoding key enzymes in the TCA cycle were significantly up-regulated by polymyxin treatments (2 mg L−1 or 0.75 × MIC polymyxin treatment for 15 min) in at least one strain. The commonly down-regulated DEGs include those for fatty acid biosynthesis (Fig. 6 and 7). It is very likely that in A. baumannii polymyxin treatments resulted in cell membrane damage and various degree of LPS loss in the outer membrane, leading to increased synthesis of LPS and inhibition of fatty acid synthesis.
Notably, 18 of the common 47 DEGs at 15 min encoded hypothetical proteins. Pairwise BLASTp search showed that three up-regulated DEGs (G1632, G1967 and G5620) were close to rcnB in E. coli (E value ≤ 0.2 × 10−5–0.7 × 10−3, identity = 31–38%, coverage = 35–51%). RcnB is a periplasmic modulator of Ni2+ and Co2+ efflux,71 and is essential for intracellular Ni2+ and Co2+ homeostasis in E. coli.71 Previously, we showed that rcnB was up-regulated in A. baumannii AB5075 when treated with 0.5 mg L−1 polymyxin B for 24 h. The rcnB inactivation mutant (rcnB::Tn) showed a slightly higher proportion of colistin-resistant subpopulations, compared to the wild-type strain.72 Taken together, these findings suggested that rcnB may play an important role in A. baumannii in response to polymyxin treatments.
Conclusions
Collectively, A. baumannii commonly up-regulated genes in lipid transport and fatty acid metabolism, and down-regulated genes in fatty acid biosynthesis as a rapid response to polymyxin treatments. A. baumannii could also change the gene expression of RND transporters and pili assembly in response to polymyxins in a strain-specific manner. To the best of our knowledge, this is the first pan-transcriptomics study in A. baumannii to determine common and unique gene expression patterns in responses to polymyxins. These findings provide important mechanistic insights into the mechanisms of polymyxin antibacterial activity and resistance in A. baumannii.
Authors’ contributions
M. L., Y. Z. and J. L. conceived the study. M. L. collected and analysed the data. S. A., M. U. A., M. H. and M. A. K. A. contributed to the pathway analysis. M. L. wrote the manuscript. C. L., J. S., J. B., D. P., T. V., Y. Z. and J. L. revised the manuscript. All authors read and approved the final manuscript.
Conflicts of interest
The authors declared no competing interest.
Acknowledgements
The authors thank Professor David A. Rasko at the University of Maryland for sharing transcriptomic data via GEO database. J. L. and T. V. were supported by National Institute of Allergy and Infectious Diseases of the National Institutes of Health (R01 AI132154). J. L. is an Australian National Health and Medical Research Council (NHMRC) Principal Research Fellow and T. V. is an NHMRC Career Development Level 2 Fellow. C. L. is an NHMRC CJ Martin Early Career Research Fellow. The content of this study is solely the responsibility of the authors and does not necessarily represent the official views of the National Institute of Allergy and Infectious Diseases or the National Institutes of Health.
References
- N. M. Clark, G. G. Zhanel and J. P. Lynch, 3rd, Emergence of antimicrobial resistance among Acinetobacter species: a global threat, Curr. Opin. Crit. Care, 2016, 22(5), 491–499 CrossRef PubMed.
- C. M. Harding, S. W. Hennon and M. F. Feldman, Uncovering the mechanisms of Acinetobacter baumannii virulence, Nat. Rev. Microbiol., 2018, 16(2), 91–102 CrossRef CAS PubMed.
-
World Health Organization, Global priority list of antibiotic-resistant bacteria to guide research, discovery, and development of new antibiotics, World Health Organization, Geneva, 2017 Search PubMed.
- A. Y. Peleg, H. Seifert and D. L. Paterson,
Acinetobacter baumannii: emergence of a successful pathogen, Clin. Microbiol. Rev., 2008, 21(3), 538–582 CrossRef CAS PubMed.
- L. Dijkshoorn, A. Nemec and H. Seifert, An increasing threat in hospitals: multidrug-resistant Acinetobacter baumannii, Nat. Rev. Microbiol., 2007, 5(12), 939–951 CrossRef CAS PubMed.
- F. Perez, A. M. Hujer, K. M. Hujer, B. K. Decker, P. N. Rather and R. A. Bonomo, Global challenge of multidrug-resistant Acinetobacter baumannii, Antimicrob. Agents Chemother., 2007, 51(10), 3471–3484 CrossRef CAS PubMed.
- L. Diancourt, V. Passet, A. Nemec, L. Dijkshoorn and S. Brisse, The population structure of Acinetobacter baumannii: expanding multiresistant clones from an ancestral susceptible genetic pool, PLoS One, 2010, 5(4), e10034 CrossRef PubMed.
- F. Imperi, L. C. Antunes, J. Blom, L. Villa, M. Iacono and P. Visca,
et al., The genomics of Acinetobacter baumannii: insights into genome plasticity, antimicrobial resistance and pathogenicity, IUBMB Life, 2011, 63(12), 1068–1074 CrossRef CAS PubMed.
- R. G. Benedict and A. F. Langlykke, Antibiotic activity of Bacillus polymyxa, J. Bacteriol., 1947, 54(1), 24 CAS.
- T. Velkov, K. D. Roberts, P. E. Thompson and J. Li, Polymyxins: a new hope in combating Gram-negative superbugs, Future Med. Chem., 2016, 8(10), 1017–1025 CrossRef CAS PubMed.
- J. Li, R. L. Nation, J. D. Turnidge, R. W. Milne, K. Coulthard and C. R. Rayner,
et al., Colistin: the re-emerging antibiotic for multidrug-resistant Gram-negative bacterial infections, Lancet Infect. Dis., 2006, 6(9), 589–601 CrossRef CAS.
- A. P. Zavascki, L. Z. Goldani, J. Li and R. L. Nation, Polymyxin B for the treatment of multidrug-resistant pathogens: a critical review, J. Antimicrob. Chemother., 2007, 60(6), 1206–1215 CrossRef CAS PubMed.
- T. Velkov, K. D. Roberts, R. L. Nation, P. E. Thompson and J. Li, Pharmacology of polymyxins: new insights into an ‘old’ lass of antibiotics, Future Microbiol., 2013, 8(6), 711–724 CrossRef CAS PubMed.
- T. Mogi, Y. Murase, M. Mori, K. Shiomi, S. Omura and M. P. Paranagama,
et al., Polymyxin B identified as an inhibitor of alternative NADH dehydrogenase and malate: quinone oxidoreductase from the Gram-positive bacterium Mycobacterium smegmatis, J. Biochem., 2009, 146(4), 491–499 CrossRef CAS PubMed.
- Z. Z. Deris, J. Akter, S. Sivanesan, K. D. Roberts, P. E. Thompson and R. L. Nation,
et al., A secondary mode of action of polymyxins against Gram-negative bacteria involves the inhibition of NADH–quinone oxidoreductase activity, J. Antibiot., 2014, 67(2), 147–151 CrossRef CAS PubMed.
- S. Baron, L. Hadjadj, J. M. Rolain and A. O. Olaitan, Molecular mechanisms of polymyxin resistance: knowns and unknowns, Int. J. Antimicrob. Agents, 2016, 48(6), 583–591 CrossRef CAS PubMed.
- R. Henry, B. Crane, D. Powell, D. Deveson Lucas, Z. Li and J. Aranda,
et al., The transcriptomic response of Acinetobacter baumannii to colistin and doripenem alone and in combination in an in vitro pharmacokinetics/pharmacodynamics model, J. Antimicrob. Chemother., 2015, 70(5), 1303–1313 CrossRef CAS PubMed.
- R. Henry, N. Vithanage, P. Harrison, T. Seemann, S. Coutts and J. H. Moffatt,
et al., Colistin-resistant, lipopolysaccharide-deficient Acinetobacter baumannii responds to lipopolysaccharide loss through increased expression of genes involved in the synthesis and transport of lipoproteins, phospholipids, and poly-beta-1,6-N-acetylglucosamine, Antimicrob. Agents Chemother., 2012, 56(1), 59–69 CrossRef CAS PubMed.
- D. Deveson Lucas, B. Crane, A. Wright, M. L. Han, J. Moffatt and D. Bulach,
et al., Emergence of high-level colistin resistance in an Acinetobacter baumannii clinical isolate mediated by inactivation of the global regulator H-NS, Antimicrob. Agents Chemother., 2018, 62(7), e02442 CrossRef PubMed.
- J. H. Moffatt, M. Harper, P. Harrison, J. D. Hale, E. Vinogradov and T. Seemann,
et al., Colistin resistance in Acinetobacter baumannii is mediated by complete loss of lipopolysaccharide production, Antimicrob. Agents Chemother., 2010, 54(12), 4971–4977 CrossRef CAS PubMed.
- M. D. Adams, G. C. Nickel, S. Bajaksouzian, H. Lavender, A. R. Murthy and M. R. Jacobs,
et al., Resistance to colistin in Acinetobacter baumannii associated with mutations in the PmrAB two-component system, Antimicrob. Agents Chemother., 2009, 53(9), 3628–3634 CrossRef CAS PubMed.
- J. H. Moffatt, M. Harper, B. Adler, R. L. Nation, J. Li and J. D. Boyce, Insertion sequence ISAba11 is involved in colistin resistance and loss of lipopolysaccharide in Acinetobacter baumannii, Antimicrob. Agents Chemother., 2011, 55(6), 3022–3024 CrossRef CAS PubMed.
- Y. K. Park, J. Y. Lee and K. S. Ko, Transcriptomic analysis of colistin-susceptible and colistin-resistant isolates identifies genes associated with colistin resistance in Acinetobacter baumannii, Clin. Microbiol. Infect., 2015, 21(8), 765 e1–765 e7 CrossRef PubMed.
- S. E. Cheah, M. D. Johnson, Y. Zhu, B. T. Tsuji, A. Forrest and J. B. Bulitta,
et al., Polymyxin resistance in Acinetobacter baumannii: genetic mutations and transcriptomic changes in response to clinically relevant dosage regimens, Sci. Rep., 2016, 6, 1–11 CrossRef PubMed.
- T. Barrett, S. E. Wilhite, P. Ledoux, C. Evangelista, I. F. Kim and M. Tomashevsky,
et al., NCBI GEO: archive for functional genomics data sets – update, Nucleic Acids Res., 2013, 41, D991–D995 CrossRef CAS PubMed.
- Y. Kodama, M. Shumway and R. Leinonen, International Nucleotide Sequence Database C. The Sequence Read Archive: explosive growth of sequencing data, Nucleic Acids Res., 2012, 40, D54–D56 CrossRef CAS PubMed.
- P. A. Kitts, D. M. Church, F. Thibaud-Nissen, J. Choi, V. Hem and V. Sapojnikov,
et al., Assembly: a resource for assembled genomes at NCBI, Nucleic Acids Res., 2016, 44(D1), D73–D80 CrossRef CAS PubMed.
- Y. Liao, G. K. Smyth and W. Shi, The Subread aligner: fast, accurate and scalable read mapping by seed-and-vote, Nucleic Acids Res., 2013, 41(10), e108 CrossRef PubMed.
- Y. Liao, G. K. Smyth and W. Shi, featureCounts: an efficient general purpose program for assigning sequence reads to genomic features, Bioinformatics, 2014, 30(7), 923–930 CrossRef CAS PubMed.
- D. M. Aanensen and B. G. Spratt, The multilocus sequence typing network: mlst.net, Nucleic Acids Res., 2005, 33, W728–W733 CrossRef CAS PubMed.
- M. C. Maiden, M. J. Jansen van Rensburg, J. E. Bray, S. G. Earle, S. A. Ford and K. A. Jolley,
et al., MLST revisited: the gene-by-gene approach to bacterial genomics, Nat. Rev. Microbiol., 2013, 11(10), 728–736 CrossRef CAS PubMed.
- A. J. Page, C. A. Cummins, M. Hunt, V. K. Wong, S. Reuter and M. T. Holden,
et al., Roary: rapid large-scale prokaryote pan genome analysis, Bioinformatics, 2015, 31(22), 3691–3693 CrossRef CAS PubMed.
- A. Stamatakis, RAxML version 8: a tool for phylogenetic analysis and
post-analysis of large phylogenies, Bioinformatics, 2014, 30(9), 1312–1313 CrossRef CAS PubMed.
- I. Letunic and P. Bork, Interactive tree of life (iTOL) v3: an online tool for the display and annotation of phylogenetic and other trees, Nucleic Acids Res., 2016, 44(W1), W242–W245 CrossRef CAS PubMed.
- M. D. Robinson, D. J. McCarthy and G. K. Smyth, edgeR: a bioconductor package for differential expression analysis of digital gene expression data, Bioinformatics, 2010, 26(1), 139–140 CrossRef CAS PubMed.
- Y. Benjamini and H. Yosef, Controlling the false discovery rate: a practical and powerful approach to multiple testing, J. R. Stat. Soc. Series B Stat. Methodol., 1995, 57, 289–300 Search PubMed.
- Z. Gu, R. Eils and M. Schlesner, Complex heatmaps reveal patterns and correlations in multidimensional genomic data, Bioinformatics, 2016, 32(18), 2847–2849 CrossRef CAS PubMed.
- H. Chen and P. C. Boutros, VennDiagram: a package for the generation of highly-customizable Venn and Euler diagrams in R, BMC Bioinf., 2011, 12, 35 CrossRef PubMed.
- J. Huerta-Cepas, D. Szklarczyk, K. Forslund, H. Cook, D. Heller and M. C. Walter,
et al., eggNOG 4.5: a hierarchical orthology framework with improved functional annotations for eukaryotic, prokaryotic and viral sequences, Nucleic Acids Res., 2016, 44(D1), D286–D293 CrossRef CAS PubMed.
- M. Li, G. Hong, J. Cheng, J. Li, H. Cai and X. Li,
et al., Identifying reproducible molecular biomarkers for gastric cancer metastasis with the aid of recurrence information, Sci. Rep., 2016, 6, 24869 CrossRef CAS PubMed.
- V. Cafiso, S. Stracquadanio, F. Lo Verde, G. Gabriele, M. L. Mezzatesta and C. Caio,
et al., Colistin Resistant A. baumannii: Genomic and Transcriptomic Traits Acquired Under Colistin Therapy, Front. Microbiol., 2018, 9, 3195 CrossRef PubMed.
- S. Thong, B. Ercan, F. Torta, Z. Y. Fong, H. Y. Wong and M. R. Wenk,
et al., Defining key roles for auxiliary proteins in an ABC transporter that maintains bacterial outer membrane lipid asymmetry, eLife, 2016, 5, e19042 CrossRef PubMed.
- A. Y. Mitrophanov and E. A. Groisman, Signal integration in bacterial two-component regulatory systems, Genes Dev., 2008, 22(19), 2601–2611 CrossRef CAS PubMed.
- A. Y. Bhagirath, Y. Li, R. Patidar, K. Yerex, X. Ma and A. Kumar,
et al., Two component regulatory systems and antibiotic resistance in Gram-negative pathogens, Int. J. Mol. Sci., 2019, 20(7), 1781 CrossRef CAS PubMed.
- C. Kroger, S. C. Kary, K. Schauer and A. D. Cameron, Genetic regulation of virulence and antibiotic resistance in Acinetobacter baumannii, Genes, 2016, 8(1), 12 CrossRef PubMed.
- M. F. Lin, Y. Y. Lin, H. W. Yeh and C. Y. Lan, Role of the BaeSR two-component system in the regulation of Acinetobacter baumannii adeAB genes and its correlation with tigecycline susceptibility, BMC Microbiol., 2014, 14, 119 CrossRef PubMed.
- M. F. Lin, Y. Y. Lin and C. Y. Lan, The role of the two-component system BaeSR in disposing chemicals through regulating transporter systems in Acinetobacter baumannii, PLoS One, 2015, 10(7), e0132843 CrossRef PubMed.
- Y. Fujita, H. Matsuoka and K. Hirooka, Regulation of fatty acid metabolism in bacteria, Mol. Microbiol., 2007, 66(4), 829–839 CrossRef CAS PubMed.
- M. S. Davis, J. Solbiati and J. E. Cronan, Overproduction of acetyl-CoA carboxylase activity increases the rate of fatty acid biosynthesis in Escherichia coli, J. Biol. Chem., 2000, 275(37), 28593–28598 CrossRef CAS PubMed.
- A. H. Choi, L. Slamti, F. Y. Avci, G. B. Pier and T. Maira-Litran, The pgaABCD locus of Acinetobacter baumannii encodes the production of poly-beta-1-6-N-acetylglucosamine, which is critical for biofilm formation, J. Bacteriol., 2009, 191(19), 5953–5963 CrossRef CAS PubMed.
- U. J. Kim, H. K. Kim, J. H. An, S. K. Cho, K. H. Park and H. C. Jang, Update on the epidemiology, treatment, and outcomes of carbapenem-resistant Acinetobacter infections, Chonnam Med. J., 2014, 50(2), 37–44 CrossRef CAS PubMed.
- A. P. Tomaras, C. W. Dorsey, R. E. Edelmann and L. A. Actis, Attachment to and biofilm formation on abiotic surfaces by Acinetobacter baumannii: involvement of a novel chaperone-usher pili assembly system, Microbiology, 2003, 149(Pt 12), 3473–3484 CrossRef CAS PubMed.
- K. M. Clemmer, R. A. Bonomo and P. N. Rather, Genetic analysis of surface motility in Acinetobacter baumannii, Microbiology, 2011, 157(Pt 9), 2534–2544 CrossRef CAS PubMed.
- D. B. Martin and P. R. Vagelos, The mechanism of tricarboxylic acid cycle regulation of fatty acid synthesis, J. Biol. Chem., 1962, 237, 1787–1792 CAS.
- F. Kalamorz, B. Reichenbach, W. Marz, B. Rak and B. Gorke, Feedback control of glucosamine-6-phosphate synthase GlmS expression depends on the small RNA GlmZ and involves the novel protein YhbJ in Escherichia coli, Mol. Microbiol., 2007, 65(6), 1518–1533 CrossRef CAS PubMed.
- T. E. Wilson, M. L. Day, T. Pexton, K. A. Padgett, M. Johnston and J. Milbrandt,
In vivo mutational analysis of the NGFI-A zinc fingers, J. Biol. Chem., 1992, 267(6), 3718–3724 CAS.
- S. Kim, J. C. Malinverni, P. Sliz, T. J. Silhavy, S. C. Harrison and D. Kahne, Structure and function of an essential component of the outer membrane protein assembly machine, Science, 2007, 317(5840), 961–964 CrossRef CAS PubMed.
- J. C. Malinverni, J. Werner, S. Kim, J. G. Sklar, D. Kahne and R. Misra,
et al., YfiO stabilizes the YaeT complex and is essential for outer membrane protein assembly in Escherichia coli, Mol. Microbiol., 2006, 61(1), 151–164 CrossRef CAS PubMed.
- Z. Wang, M. Gerstein and M. Snyder, RNA-Seq: a revolutionary tool for transcriptomics, Nat. Rev. Genet., 2009, 10(1), 57–63 CrossRef CAS PubMed.
- N. Karah, A. Sundsfjord, K. Towner and O. Samuelsen, Insights into the global molecular epidemiology of carbapenem non-susceptible clones of Acinetobacter baumannii, Drug Resist. Updates, 2012, 15(4), 237–247 CrossRef CAS PubMed.
- M. Matsui, M. Suzuki, M. Suzuki, J. Yatsuyanagi, M. Watahiki and Y. Hiraki,
et al., Distribution and molecular characterization of Acinetobacter baumannii international clone II lineage in Japan, Antimicrob. Agents Chemother., 2018, 62(2), e02190 Search PubMed.
- L. A. Ronish, E. Lillehoj, J. K. Fields, E. J. Sundberg and K. H. Piepenbrink, The structure of PilA from Acinetobacter baumannii AB5075 suggests a mechanism for functional specialization in Acinetobacter type IV pili, J. Biol. Chem., 2019, 294(1), 218–230 CrossRef CAS PubMed.
- K. H. Piepenbrink, E. Lillehoj, C. M. Harding, J. W. Labonte, X. Zuo and C. A. Rapp,
et al., Structural diversity in the Type IV pili of multidrug-resistant Acinetobacter, J. Biol. Chem., 2016, 291(44), 22924–22935 CrossRef CAS PubMed.
- J. Jagnow and S. Clegg,
Klebsiella pneumoniae MrkD-mediated biofilm formation on extracellular matrix- and collagen-coated surfaces, Microbiology, 2003, 149(Pt 9), 2397–2405 CrossRef CAS PubMed.
-
A. R. Nusaibah, S.-E. Cheah, Z. Yan, D. J. Matthew, B. John and H. Y. Heidi, et al., Integrative multi-omics network analysis of the synergistic killing of polymyxin B and chloramphenicol in combination against an NDM-producing Klebsiella pneumoniae isolate, Abstracts of the 26th European Congress of Clinical Microbiology and Infectious Diseases, Amsterdam, The Netherlands, 2016, Poster 4070, ESCMID, Basel, Switzerland.
- J. C. Malinverni and T. J. Silhavy, An ABC transport system that maintains lipid asymmetry in the Gram-negative outer membrane, Proc. Natl. Acad. Sci. U. S. A., 2009, 106(19), 8009–8014 CrossRef CAS PubMed.
- S. M. McLeod, P. R. Fleming, K. MacCormack, R. E. McLaughlin, J. D. Whiteaker and S. Narita,
et al., Small-molecule inhibitors of Gram-negative lipoprotein trafficking discovered by phenotypic screening, J. Bacteriol., 2015, 197(6), 1075–1082 CrossRef CAS PubMed.
- R. G. Raffa and T. L. Raivio, A third envelope stress signal transduction pathway in Escherichia coli, Mol. Microbiol., 2002, 45(6), 1599–1611 CrossRef CAS PubMed.
- X. Wang, J. F. Preston, 3rd and T. Romeo, The pgaABCD locus of Escherichia coli promotes the synthesis of a polysaccharide adhesin required for biofilm formation, J. Bacteriol., 2004, 186(9), 2724–2734 CrossRef CAS PubMed.
- M. Akram, Citric acid cycle and role of its intermediates in metabolism, Cell Biochem. Biophys., 2014, 68(3), 475–478 CrossRef CAS PubMed.
- C. Bleriot, G. Effantin, F. Lagarde, M. A. Mandrand-Berthelot and A. Rodrigue, RcnB is a periplasmic protein essential for maintaining intracellular Ni and Co concentrations in Escherichia coli, J. Bacteriol., 2011, 193(15), 3785–3793 CrossRef CAS PubMed.
-
T. B. Tran, Treatment of multidrug-resistant Acinetobacter baumannii with novel combinations of polymyxin B and other non-antibiotic drugs, Doctoral thesis, Monash University, Melbourne, Australia, 2017.
Footnote |
† Electronic supplementary information (ESI) available: Supplementary Material 1. Supplementary Fig. S1. Gene expression of the core genome in the rest of COG categories of five A. baumannii strains. log2 fold changes are shown in heatmaps. A: RNA processing and modification; C: energy production and conversion; D: cell cycle control, cell division, chromosome partitioning; E: amino acid transport and metabolism; F: nucleotide transport and metabolism; G: carbohydrate metabolism and transport; H: coenzyme transport and metabolism; J: translation, ribosomal structure and biogenesis; K: transcription; L: replication, recombination and repair; O: posttranslational modification, protein turnover, chaperones; P: inorganic ion transport and metabolism; Q: secondary metabolites biosynthesis, transport and catabolism; S: function unknown; and T: signal transduction mechanisms. Supplementary material 2. Supplementary Tables S1–S6. Table S1. 84 complete genomes from RefSeq; Table S3. 41 DEGs uniquely identified by FDR < 0.05 and FC ≥ 1.5; Table S3. 2822 core genes and fold changes in their expression after polymyxin treatments; Table S4. Common DEGs between samples treated with 2 mg L−1 colistin at 15 and 60 min; Table S5. Unique DEGs from samples treated with 2 mg L−1 colistin at 15 min; Table S6. Unique DEGs from samples treated with 2 mg L−1 colistin at 60 min. See DOI: 10.1039/d0mo00015a |
|
This journal is © The Royal Society of Chemistry 2020 |
Click here to see how this site uses Cookies. View our privacy policy here.