DOI:
10.1039/C9MT00232D
(Paper)
Metallomics, 2020,
12, 104-113
Synthesis, characterization, and antitumor properties of Au(I)–thiourea complexes†
Received
15th September 2019
, Accepted 14th November 2019
First published on 15th November 2019
Abstract
The anticancer property of cisplatin has stimulated the development of metal complexes as antitumor agents. Among these complexes, metal thiourea complexes have attracted sufficient attention, and they possess the potential possibility to become new antitumor metallodrugs. Herein, four Au(I) complexes derived from N,N-disubstituted cyclic thiourea ligands were synthesized and characterized. The crystal structure analysis indicated that the complex Au(I)(3c)2OTf was a mononuclear crystal structure with Au(I) coordinated by two sulfur atoms. These Au(I) complexes exhibited excellent toxicities against several tumor cell lines, especially complex Au(I)(3c)2OTf (IC50 = 8.06 μM against HeLa). It was found that Au(I)(3c)2OTf triggered a burst of ROS, disrupted the mitochondrial membrane potential (MMP), subsequently released Cyt-c, and then triggered the activation of caspase 9, caspase 7 and caspase 3. Mechanism experiments manifested that Au(I)(3c)2OTf induced the down-regulation of Bcl-2 and up-regulation of Bax, which further indicated that Au(I)(3c)2OTf triggered mitochondria-mediated apoptosis. In addition, the ROS scavenger-NAC completely blocked the apoptosis and inhibited the reduction of MMP, showing that Au(I)(3c)2OTf induced a ROS-dependent apoptosis pathway. These results indicate that Au(I)(3c)2OTf is worthy of in-depth research as an antitumor agent and may throw light on a better understanding of the effect of thiourea derivatives on antitumor mechanisms.
Significance to metallomics
Au(I) complexes have wide applications in the treatment of tumors. In this work, we synthesized and explored the cytotoxicity of a series of Au(I) complexes (N,N′-disubstituted cyclic thiourea ligands): (1) a series of cyclic thiourea ligands and their Au(I) complexes were synthesized characterized. The X-ray crystallography data indicated that Au(I)(3c)2OTf was coordinated by two S atoms from two thiourea ligands, forming a two-coordinated complex. (2) These Au(I) complexes showed excellent toxicities, especially Au(I)(3c)2OTf. Mechanism research indicated that Au(I)(3c)2OTf induced a burst of ROS, collapse of the MMP and the release of Cyt-c. Moreover, the Au(I)(3c)2OTf activated caspase 3, 7 and 9, and triggered the disordered expression of Bcl-2 and Bax, confirming the synergistic mitochondria-mediated apoptosis. In addition, the ROS scavenger-NAC could completely reverse the apoptosis induced by Au(I)(3c)2OTf and inhibited the collapse of the MMP, showing a ROS-dependent apoptosis pathway. We are convinced that these above findings will contribute to the toxicity studies of metal complexes and attract wide interest.
|
Introduction
Cisplatin, a star molecule in chemotherapy drugs, has been extensively applied in cancer treatment.1–3 In spite of its widespread clinical use, the resistance of tumor cells to cisplatin usually results in chemotherapy failure.4–6 On the other side, the discovery of cisplatin toxicity mostly stimulated the development of other metal drugs for treating cancer.7–9 In the past few decades, there has been a great amount of interest in gold metal complexes as antitumor drugs in medicinal chemistry.10–14 The particular features of metal ions can be utilized in rational drug design, to increase selectivity, or sustain other action mechanisms to current drugs. Since Au(I) has a strong affinity for soft atoms such as S and Se, it has been reported that the target molecule of Au(I) complexes is thioredoxin reductase,15–18 which is a thiol- and selenol-containing protein closely related to the redox homeostasis inside cells. According to the literature reports, Au(I) complexes based on N-heterocyclic carbine19,20 and bidentate phosphine ligands have been shown to be selectively toxic to tumor cells rather than normal cells and displayed a mechanism of inhibiting the activity of thioredoxin reductase in mitochondria.
Thiourea derivatives are well documented in coordination chemistry, and can chelate with many metal ions, such as potentially compatible atoms (O, S) in the coordination reactions.21,22 For example, the benzoyl thioureas can chelate with transition metals,23,24 forming complexes with S, O coordination. Due to their various coordination modes and wide application, thiourea and its metal complexes have gained growing interest, such as in metal exaction,25 synthetic precursors for nanomaterials,26 growth regulators for plants and biological activities. Because of the S atom in thiourea, which possesses strong nucleophilicity, the thiourea ligands have strong affiliative ability with Au(I). The highlight of the studies is that thiourea metal complexes are more effective as antitumor agents. However, the relationship between the structure and biological activity and stability of thiourea metal complexes need further research, which will help to design more effective anti-tumor drugs. Che and co-workers reported “Gold(I) complex of N,N′-disubstituted cyclic thiourea with in vitro and in vivo anticancer properties – potent tight-binding inhibition of thioredoxin reductase”.18 They synthesized three coinage metal complexes (Au, Ag, Cu) of an N,N′-disubstituted cyclic thiourea ligand and studied the antitumor mechanism of the Au(I) complex. The IC50 value of their Au(I) complex was 14.6 μM in the HeLa cell line. The Au(I) complex was found to specifically inhibit the activity of thioredoxin reductase. As we all know, halogens, especially F and Cl, can be found everywhere in medicinal chemistry because of their special properties. They can be not only regarded as a Lewis base, but also hydrophobic moieties.27 Besides, compounds substituted with halogens can form “halogen bonding”.28 The diverse interaction possibilities between ligand and protein make halogens a useful tool during structure–activity relationship research. For example, 5-fluorouracil (5-FU), which is widely used for stomach, breast and cervical cancer, is one of the safest and most effective drugs in the health system. Therefore, we wondered if the biological properties of the Au(I) complex would become better if the substituent was changed to halogens. Here, we report the synthesis, characterization and antitumor properties of new N,N-disubstituted cyclic thiourea ligands and their Au(I) metal complexes.
Results and discussion
Synthesis
Intermediates 2a–2d were synthesized following Fu's method with high yields29 (Scheme 1). To obtain the compounds 3a–3d, the “thiocarbonyl diimidazole” method30 was applied due to its advantages of high yield and purity. The reaction of the thiourea ligands with AuOTf gave the corresponding Au(I) complexes, followed by transmetallation with [Au(THT)Cl], and then the expected Au(I) complexes were obtained with high purity.
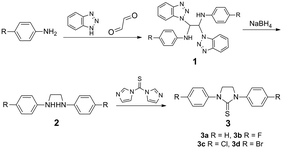 |
| Scheme 1 Synthetic procedure for the ligands. | |
Crystal structure
The crystal structure of the complex – Au(I)(3c)2OTf was determined by single-crystal X-ray diffraction. The crystallographic data and refinement details are listed in Table S1 (ESI†). The molecular structures of Au(I)(3c)2OTf are depicted in Fig. 1. As displayed in Fig. 1, the center Au(I) was coordinated by two S atoms from two thiourea ligands, forming a two-coordinated complex. The geometry of the Au atom was distorted linear, with the bond angle S(1)–Au(1)–S(1) of 175.03°. The bond angle of Au(1)–S(1) was 2.289 Å, which was similar to the other Au(I) complex. Selected bond distances and angles are displayed in Table S2 (ESI†).
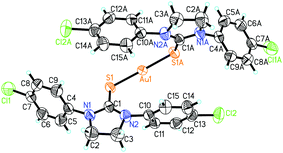 |
| Fig. 1 The ORTEP molecular structure of complex Au(I)(3c)2OTf shown as 50% thermal ellipsoid probability. There are two halves of complex Au(I)(3c)2OTf in the asymmetric unit and only one complete molecule is shown. Symmetry code: A = 1 − x, − y, z. | |
Cytotoxicity and cell cycle
The MTT assay was employed to measure the cell viability to the complexes. The IC50 values against the four cell lines (HeLa, MCF-7, SGC-7901, GES-1) were summarized as follows. As displayed in Table 1, all the Au(I) complexes showed apparent toxicities to the three cancer cell lines and lower toxicity to the normal GES-1 cell line, which exhibited good selectivity, especially complex Au(I)(3c)2OTf. It possessed an IC50 of 8.06 μM, which was lower than that in previous literature reported by Che and co-workers,18 displaying better toxicity. The result was not surprising, since the substitution of Cl could improve the biological activity. Complex Au(I)(3c)2OTf was significantly more toxic. So, Au(I)(3c)2OTf was selected for further mechanism experiments. Moreover, the IC50 values of the relevant thiourea ligands were over 100 μM, which are not given in the table, suggesting that the biological activities of the complexes were largely metal-mediated. The antitumor activities of these Au(I) complexes may be ascribed to their interactions with biological ligands such as DNA and proteins.
Table 1 IC50 values of Au(I) complexes against cancerous and non-tumor cells
IC50 (μM) |
HeLa |
MCF-7 |
SGC-7901 |
GES-1 |
Au(I)(3a)2OTf
|
17.01 ± 3.29 |
24.15 ± 1.77 |
24.23 ± 7.44 |
29.86 ± 5.92 |
Au(I)(3b)2OTf
|
19.27 ± 14.97 |
19.35 ± 11.67 |
18.52 ± 8.82 |
45.11 ± 33.63 |
Au(I)(3c)2OTf
|
8.06 ± 0.63 |
20.35 ± 10.39 |
16.43 ± 1.86 |
48.17 ± 14.13 |
Au(I)(3d)2OTf
|
17.75 ± 14.30 |
32.20 ± 31.11 |
24.71 ± 16.90 |
59.47 ± 20.12 |
There are many reasons for the inhibition of tumor cell proliferation by toxic complexes, such as cell cycle arrest, apoptosis, ROS regulation, DNA cleavage, or a combination of these factors. Therefore, we explored the features involved in these cases. Firstly, PI was applied to detect the effect of Au(I)(3c)2OTf on cell cycle distribution. As shown in Fig. S1 (ESI†), the cell cycle distribution remained stable when the changes in phase ratio remained under 5%, indicating that the toxicity aroused by Au(I)(3c)2OTf was probably not due to cell cycle arrest.
Apoptosis measurement
Next, we executed apoptosis studies to illuminate the effects of Au(I)(3c)2OTf on HeLa cells through Annexin-V/PI double staining and a TUNEL experiment. It was confirmed that Au(I)(3c)2OTf induced apoptosis through flow cytometry and confocal experiments. Apoptotic cells experience morphological transformation. The phosphatidylserine residues translocate from the membrane's interior to the exterior, a symbol of early apoptosis, and this can be tested by Annexin-V but not PI, as the cell membrane is integrated while the phosphatidylserine residues are exterior. Late apoptotic and necrotic cells are both Annexin-V-positive and PI-positive. As displayed in Fig. 2(a), when the HeLa cells were treated with 5 μM Au(I)(3c)2OTf, the proportion of apoptotic cells reached 40%. When the dose concentration increased to 10 μM, the number of apoptotic cells rose up to 70%, exhibiting a dose-dependent manner. The TUNEL experimental result is shown in Fig. 2(b). In the control groups, the cell nuclei displayed homogenous and round blue staining. In the cells treated with Au(I)(3c)2OTf, the nuclei became concentrated, smaller and showed green fluorescence, which indicated early apoptosis. However, these phenomena have not been detected in the untreated group. Therefore, we concluded that Au(I)(3c)2OTf could induce cell death through apoptosis.
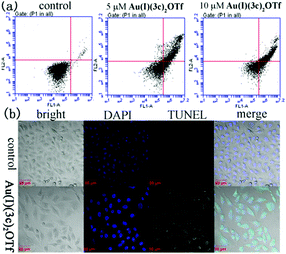 |
| Fig. 2 Induction of apoptosis of HeLa cells treated by Au(I)(3c)2OTf measured by PI and Annexin V-FITC staining (a) and a TUNEL experiment (b). | |
Disruption of mitochondrial membrane potential (MMP)
Au(I)(3c)2OTf triggered the collapse of the mitochondrial membrane potential (MMP). Another critical factor during apoptosis is the decline of the mitochondrial membrane potential (MMP) which leads to the release of Cyt-c (pro-apoptotic factors).31 MMP is a sensitive indicator for measuring mitochondrial function.32 It relates to the mitochondrial function and the activity of electron transport. The normal cells show good-defined mitochondrial membrane integrity, while apoptotic cells usually exhibit the collapse of the MMP.33,34 In order to determine whether Au(I)(3c)2OTf induced the depletion of MMP, Rh123 was employed to detect the MMP. Rh123 is a cationic, lipophilic dye that preferentially concentrates in mitochondria according to the highly negative MMP. When the MMP depolarizes, Rh123 would be free from the mitochondria, and the intracellular fluorescence intensity decreases. As shown in Fig. 3, the MMP of cells treated with 8 μM Au(I)(3c)2OTf significantly decreased by 25%. When the concentration increased to 16 μM, Au(I)(3c)2OTf nearly resulted in a 40% reduction of cellular MMP. These results indicated that Au(I)(3c)2OTf obviously disrupted the cellular MMP, probably triggered by mitochondria-mediated apoptosis.
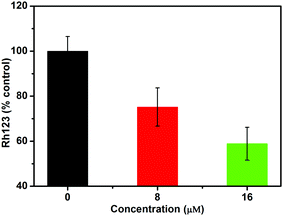 |
| Fig. 3 Effect of Au(I)(3c)2OTf on mitochondrial membrane potential analyzed by Rh123. | |
The release of Cyt-c from mitochondria
The experiments revealed that Au(I)(3c)2OTf induced the release of Cyt-c in a dose-dependent manner. Cyt-c is an electron transporter which resides in the inter-membrane space of the mitochondria.35–37 It serves as an electron carrier during the procedure of oxidative phosphorylation and generation of ATP. It is also a vital index during the intrinsic pathway of apoptosis and acts as a death messenger. So we measured the effect of Au(I)(3c)2OTf on the release of Cyt-c from the mitochondrial membrane. As displayed in Fig. 4, when the HeLa cells were treated with 4 μM Au(I)(3c)2OTf, the release of Cyt-c was 38 nmol g−1. When the dose concentration increased to 8 and 12 μM, the amount of Cyt-c was 43 and 64 nmol g−1, respectively, which was almost three times that of the control. The release of Cyt-c was probably a result of mitochondria-mediated apoptosis. Subsequently, the release of Cyt-c further activated caspase 9, which in sequence activated caspase 3, and finally resulted in cell death.38
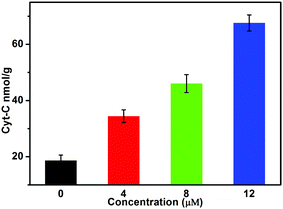 |
| Fig. 4 Measurement of Cyt-c in HeLa cells exposed to Au(I)(3c)2OTf of different concentrations. | |
Induction of mitochondria-mediated apoptosis
The Western blot tests indicated that Au(I)(3c)2OTf caused apoptosis through a mitochondria-mediated pathway. There are two widely acknowledged apoptotic pathways. One is the death receptor pathway, and the other is the mitochondrial pathway. The mitochondria-mediated pathway is involved in various mechanisms, such as MPTP opening, membrane injury or Bcl-2/Bax channel polarization. These parameters may collaboratively enhance the permeability of the mitochondrial inner membrane, which results in the collapse of the membrane potential, swelling and mitochondrial dysfunction. Since Au(I)(3c)2OTf reduced the MMP, we supposed that the mechanism of mitochondrial permeabilization was concerned with the mitochondrial-related proteins such as Bcl-2 and Bax. Bcl-2 family proteins are able to regulate the permeability of the outer mitochondrial membrane to control the mitochondrial pathway. The anti-apoptotic protein Bcl-2 locates in the outer nuclear membrane, endoplasmic reticulum and mitochondrial membrane. It can reduce chromatin condensation, cell shrinkage and DNA cleavage to inhibit cell apoptosis. Bax can be activated at the mitochondria in response to various stimuli or injuries.39 It can lead to the release of Cyt-c and induce the caspase-3 dependent and mitochondria-mediated apoptotic route. The ratio of Bcl-2/Bax has been shown to be a key factor for the apoptotic system. As shown in Fig. 5, the protein level of Bcl-2 was decreased and the expression of Bax was up-regulated in the Au(I)(3c)2OTf treated group compared with the control ones, which further proved the mitochondria-mediated apoptosis.
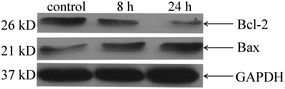 |
| Fig. 5 Western blot analysis of Bcl-2 and Bax in HeLa cells treated with Au(I)(3c)2OTf of different times (8 h, 24 h). | |
Caspase activities
The measurement of caspase activities implied that Au(I)(3c)2OTf triggered apoptosis via a caspase-dependent pathway. It is widely acknowledged that caspase family proteins play critical roles in the regulation of apoptosis, which is an evolutionarily conserved procedure with extensive links to various human diseases. In general, caspases are presented as an inactive state in the cells and become active when they encounter proteolytic enzymes. Caspase 3, 7, and 9 are among the caspase family proteins that play crucial parts in apoptosis. Caspase 9 is a vital member in the apoptosis pathway, which becomes active through the formation of the apoptosome after the release of Cyt-c from mitochondria. Activated caspase 9 which is an initiator during apoptosis furthermore activates caspase 3. Caspase 3 and caspase 7, which are executors in the mitochondrial apoptosis pathways can degrade different substrates such as regulatory and structural proteins. In this paper, Western blot analysis was applied to detect whether caspase 3, caspase 7 and caspase 9 were activated when the HeLa cells were treated with Au(I)(3c)2OTf. As shown in Fig. 6, the HeLa cells exposed to the complex Au(I)(3c)2OTf presented a noticeable rise in the activity of caspase 3 and caspase 7 as the expression of caspase 3 accompanied by caspase 7 decreased versus control, which indicated that caspase 3 and caspase 7 in HeLa cells were activated efficiently. Caspase 9 was also activated when the cells were treated with the complex Au(I)(3c)2OTf.
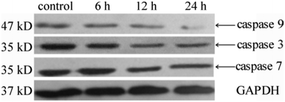 |
| Fig. 6 Expressions of caspase family proteins in HeLa cells after incubation with complex Au(I)(3c)2OTf for different times (6 h, 12 h, and 24 h). | |
Elevation of intracellular ROS generation
Au(I)(3c)2OTf also leads to the outbreak of intracellular ROS. To determine the effect of oxidative stress on Au(I)(3c)2OTf, the level of intracellular ROS generation was evaluated. It has been universally acknowledged that ROS play an essential role in apoptotic signaling pathways during chemotherapy and radiotherapy.40,41 Moderate ROS production promotes cell growth and proliferation. However, excessive generation of ROS could lead to metabolic disturbances, mitochondrial dysfunction or inflammatory responses,42 which is responsible for apoptosis. So we measured the intracellular ROS generation in HeLa cells by detecting the DCFH-DA fluorescence intensity. DCFH-DA is hydrolyzed by intracellular esterase to DCFH after entering into the cells. Then, DCFH is stuck in the cells. The intracellular ROS in cells could oxidize DCFH to a strongly fluorescent DCF. As a result, the fluorescence intensity is proportional to the amount of ROS in the cells. As shown in Fig. 7, in the cells treated with 8 μM Au(I)(3c)2OTf, the amount of intracellular ROS was 3.5 times that of the control. Moreover, when the dose concentration increased to 16 μM, the level of ROS generation was 4.9 fold that of the control, indicating that Au(I)(3c)2OTf triggered prodigious ROS generation in a dose-dependent manner and ROS may contribute a positive antitumor effect on apoptosis.
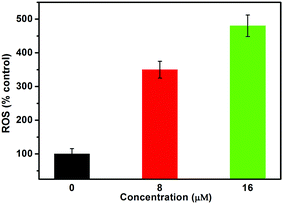 |
| Fig. 7 Analysis of ROS levels by flow cytometry after HeLa cells were treated with Au(I)(3c)2OTf at the indicated concentrations for 24 h. Quantification of the flow cytometric results with increased intracellular DCF oxidation compared to control cells. | |
Inhibition of intracellular ROS generation suppresses the MMP, and apoptosis
ROS elevation is probably the primary cause of apoptosis aroused by Au(I)(3c)2OTf. ROS have been shown to be closely related to the process of cell apoptosis. To further examine the importance of ROS on apoptosis, the ROS scavenger – N-acetyl-cysteine (NAC) was recommended. We detected the MMP, and the apoptotic proportion in the presence of NAC. As shown in Fig. 8(a), NAC completely blocked the generation of ROS induced by Au(I)(3c)2OTf. Next, we explored the influence of NAC on MMP. As presented in Fig. 8(b), in the Au(I)(3c)2OTf treated group, the MMP reduced obviously. However, when NAC was pre-cultivated, the decrement of MMP was potently impeded. Thus, NAC significantly inhibited the collapse of MMP. Fig. 8(c) showed that NAC significantly suppressed the apoptosis in Au(I)(3c)2OTf induced cell death. When the cells were incubated with Au(I)(3c)2OTf, the ratio of apoptotic cells was approximately 65%. But in the NAC co-incubated group, the number of apoptotic cells decreased significantly in contrast with the Au(I)(3c)2OTf treated cells. The protective effect of NAC was probably attributed to its antioxidant ability, which might be a result of its ability to clear free radicals.
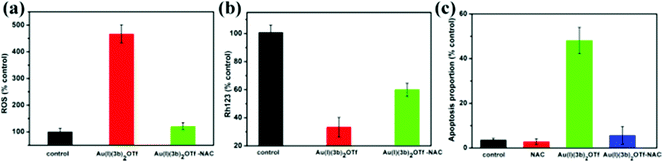 |
| Fig. 8 Effect of ROS on MMP and apoptosis. The HeLa cells were pretreated with NAC and then incubated with Au(I)(3c)2OTf for another 24 h. NAC completely blocked the intracellular ROS generation (a), NAC inhibited the collapse of MMP (b), and NAC restrained apoptosis (c). | |
Conclusion
In conclusion, this work illuminated the synthesis and antitumor properties of new Au(I) metal complexes. The results demonstrated that these Au(I) compounds obviously inhibited tumor cell growth, particularly Au(I)(3c)2OTf. Mechanism studies indicated that Au(I)(3c)2OTf triggered a burst of ROS, decreased the mitochondrial membrane potential, followed by the release of Cyt-c, and initiated a cascade of caspase 9 and caspase 7, which were cooperative effectors in apoptosis. The elevation of Bax and reduction of Bcl-2 declared that Au(I)(3c)2OTf caused a mitochondria-mediated apoptotic process. Moreover the ROS inhibitor-NAC restrained apoptosis and other apoptosis-related factors. The mechanism experiments also indicated that Au(I)(3c)2OTf induced apoptosis in a synergistic manner, which enhanced the antitumor efficacy involved in the mitochondrial signalling pathway. This work may open up deep insights for better understanding the antitumor mechanisms of metal complexes, and will attract more attention to the metal complexes as potential antitumor agents.
Experimental section
Materials and methods
All the reagents were purchased from commercial suppliers and used without further purification unless otherwise noted. The solvents were purified according to standard methods. The reactions and manipulations were performed in Schlenk flasks under a nitrogen atmosphere. 1H and 13C NMR spectra were recorded using a Mercury VX-300M spectrometer. Mass spectra (ESI-MS) were recorded using an LCQ Fleet Spectrometer. IR spectra of powder samples were recorded using a Nexus 670 Fourier spectrometer in the range of 4000–400 cm−1. Single crystal X-ray data were collected at room temperature using a Bruker Kappa APEX-Duo CCD diffractometer. [Au(THT)Cl] was prepared according to literature procedures with modification.43 Flow cytometric analysis was carried out using a BD Accuri C6. Confocal experiments were analyzed by using a Zeiss LSM 510 Laser. 2′,7′-Dichlorofluorescein diacetate (DCF-DA), Rhodamine 123 (Rh123), and N-acetylcysteine (NAC) were obtained from Sigma Aldrich. The bicinchoninic acid (BCA) kit and the antibodies for Bcl-2 and Bax were brought from Beyotime. 3-(4,5-Dimethylthiazol-2-yl)-2,5-diphenyl tetrazolium bromide (MTT) was purchased from Amresco. The antibodies of caspase family proteins were purchased from Cell Signal Technology. DMEM and other medium additives were purchased from Gibco. The GAPDH was brought from Proteintech. Milli-Q water was employed in all the experiments.
Synthesis and characterization
The synthetic routes of the ligands are shown in Scheme 1 by modifications of the literature procedures.30,44
Synthesis of 1a–1d.
Intermediates 1a–1d were obtained under the same conditions by a general synthetic procedure. To a solution of benzotriazole (2 equiv., 10 mmol) in EtOH (50 mL), aniline (2 equiv., 10 mmol) was added. The mixture was stirred for 10 min at 25 °C, and then a 40% aqueous solution of glyoxal (1 equiv., 5 mmol) was slowly added. After being stirred in EtOH overnight, a white solid was obtained through filtration and dried in vacuo.
1a: phenylamine (0.93 g, 10 mmol). Yield: (2.1 g, 94%).
1b: 4-fluoroaniline (1.11 g, 10 mmol) and employing n-propanol instead of EtOH. Yield: (2.3 g, 95%).
1c: 4-chloroaniline (1.27 g, 10 mmol), yield: (2.6 g, 94%).
1d: 4-bromoaniline (2.1 g, 10 mmol) and employing MeOH in place of EtOH. Yield: (3.2 g, 93%).
Synthesis of 2a–2d.
General procedure for the synthesis of 2a–2d.
To a suspension of 1 (1 equiv., 4 mmol) in dry THF (50 mL), sodium borohydride (3 equiv., 12 mmol) was added at 0 °C under nitrogen. The suspension was stirred at room temperature overnight to get a clear solution. The reaction was quenched by the addition of water and ethyl acetate and then the organic layer was washed with dilute sodium hydroxide solution and saturated brine, dried with Na2SO4 and concentrated under vacuum. The white solid was obtained through recrystallization from ethyl acetate.
2a (N,N'-Diphenylethane-1,2-diamine).
Yield: (0.68 g, 80%). 1H NMR (300 MHz, CDCl3): δ (ppm) 3.40 (s, 4H), 3.87 (s, 2H), 6.66 (d, J = 9 Hz, 4H), 6.74 (t, J = 9 Hz, 4H), 7.20 (t, J = 9 Hz, 2H); 13C NMR (75 MHz, CDCl3): δ (ppm) 43.52, 113.34, 118.13, 129.70, 148.36; IR (KBr, cm−1): 3417.72, 3050.21, 2945.47, 2921.87, 1600.13, 1514.78, 1496.69, 1458.14, 745.06, 693.17.
2b (N,N′-Bis(4-fluorophenyl)ethane-1,2-diamine).
Yield: (0.88 g, 82%). 1H NMR (300 MHz, CDCl3): δ (ppm) 3.35 (s, 4H), 3.76 (s, 2H), 6.61–6.57 (m, 4H), 6.91 (t, J = 9 Hz, 4H); 13C NMR (75 MHz, CDCl3): δ (ppm) 43.87, 113.85, 115.86, 144.27, 154.41, 157.53; IR (KBr, cm−1): 3406.94, 3062.04, 3039.39, 2892.32, 1614.10, 1500.12, 1478.99, 1400.60, 1310.33, 1206.19, 1128.17, 819.03, 771.42.
2c (N,N′-Bis(4-chlorophenyl)ethane-1,2-diamine).
Yield: (0.97 g, 81%). 1H NMR (300 MHz, CDCl3): δ (ppm) 3.36 (s, 4H), 3.86 (s, 2H), 6.57 (d, J = 9 Hz, 4H), 7.14 (d, J = 9 Hz, 4H); 13C NMR (75 MHz, CDCl3): δ (ppm) 41.45, 112.34, 120.57, 127.41, 144.76; IR (KBr, cm−1): 3412.66, 3022.10, 2901.73, 2850.37, 1601.01, 1540.22, 1480.17, 1402.35, 1384.41, 1321.64, 1178.38, 1120.82, 1083.61, 812.87.
2d (N,N′-bis(4-bromophenyl)ethane-1,2-diamine).
Yield: (1.36 g, 85%). 1H NMR (300 MHz, CDCl3): δ (ppm) 3.36 (s, 4H), 3.87 (s, 2H), 6.53 (d, J = 9 Hz, 4H), 7.28 (d, J = 9 Hz, 4H); 13C NMR (75 MHz, CDCl3): δ (ppm) 42.61, 108.88, 114.19, 131.58, 146.59;IR (KBr, cm−1): 3408.34, 3061.08, 3016.61, 2895.06, 2849.76, 1633.53, 1600.01, 1523.30, 1479.49, 1399.18, 1384.50, 1324.26, 1294.24, 1243.53, 1178.81, 1121.56, 1068.50, 809.28, 506.17.
Synthesis of 3a–3d
Ligands 3a–3d were prepared by a general procedure.
To a solution of compound 2 (1 equiv., 3 mmol) in dry THF was added 1,1′-thiocarbonyl diimidazole (1.1 equiv., 3.3 mmol). The solution was refluxed for 10 h and monitored by TLC. After the addition of water (10 mL) and dilute HCl (2 M), the solution was extracted with ethyl acetate (3 × 20 mL). The organic layer was combined, dried and concentrated under vacuum. The pure cyclic thiourea compounds were obtained through recrystallization from ethanol.
3a (1,3-Diphenyl-2-imidazolidinethione).
Yield: (0.61 g, 80%). 1H NMR (300 MHz, CDCl3): δ (ppm) 4.17 (s, 4 H), 7.29 (d, J = 9 Hz, 4 H), 7.44 (t, J = 9 Hz, 4 H), 7.57 (d, J = 6 Hz, 2 H); 13C NMR (75 MHz, CDCl3): δ (ppm) 49.28, 125.36, 126.55, 128.79, 140.65, 181.00; IR (KBr, cm−1): 3424.37, 3050.05, 2968.19, 2893.79, 1637.18, 1597.11, 1497.27, 1464.96, 1421.47, 1396.22, 1350.01, 1296.43, 1270.67, 759.84, 690.53, 563.39.
3b (1,3-Bis(4-fluorophenyl)imidazolidine-2-thione).
Yield: (0.75 g, 85%). 1H NMR (300 MHz, CDCl3): δ (ppm) 4.14 (s, 4H), 7.12 (t, J = 9 Hz, 4H), 7.48–7.53 (m, 4H); 13C NMR (75 MHz, CDCl3): δ (ppm) 49.50, 115.80, 127.42, 136.57, 159.25, 162.52, 181.74; IR (KBr, cm−1): 3428.31, 3081.25, 2968.49, 2917.35, 2849.72, 1604.05, 1508.74, 1467.36, 1430.80, 1394.43, 1316.62, 1287.34, 1272.61, 1235.94, 830.47, 550.36.
3c (1,3-Bis(4-chlorophenyl)imidazolidine-2-thione).
Yield: (0.77 g, 81%). 1H NMR (300 MHz, CDCl3): δ (ppm) 4.15 (s, 4H), 7.40 (d, J = 9 Hz, 4H), 7.52 (d, J = 9 Hz, 4H); 13C NMR (75 MHz, CDCl3): δ (ppm) 49.02, 126.50, 128.98, 132.06, 138.96, 180.91; IR (KBr, cm−1): 3438.42, 3093.21, 2954.84, 2885.14, 1635.50, 1592.12, 1491.50, 1470.97, 1419.10, 1401.62, 1339.54, 1290.11, 1265.86, 1091.13, 1010.42, 823.10.
3d (1,3-Bis(4-bromophenyl)imidazolidine-2-thione).
Yield: (0.74 g, 63%). 1H NMR (300 MHz, CDCl3): δ (ppm) 4.15 (s, 4H), 7.46 (d, J = 6 Hz, 4H), 7.55 (d, J = 6 Hz, 4H); 13C NMR (75 MHz, CDCl3): δ (ppm) 48.92, 119.97, 126.75, 131.91, 139.50, 180.74; IR (KBr, cm−1): 3428.90, 2925.96, 1633.86, 1488.06, 1470.73, 1417.07, 1385.69, 1336.56, 1289.19, 1190.86, 833.55, 819.02.
Synthesis of Au(I) complexes of thiourea ligands
General procedure for the synthesis of Au(I) complexes45.
To a solution of ligand 3 (2 equiv., 1 mmol) in CH2Cl2 (10 mL), AgOTf (0.5 mmol) was added under a nitrogen atmosphere. The mixture was stirred at room temperature overnight (protected from light). Then the solution was filtered, and excess Et2O (30 mL) was added to the filtrate to get a white precipitate. The precipitate was dissolved in CH2Cl2 (20 mL) and a solution of [Au(THT)Cl] (0.25 mmol) in CH2Cl2 (5 mL) was added. The reaction solution was stirred overnight and then filtered. Finally, a large amount of Et2O (50 mL) was added, and the product was precipitated as a white solid.
Au(I)(3a)2OTf
.
Yield: (0.19 g, 85%). 1H NMR (300 MHz, CDCl3): δ (ppm) 4.36 (s, 8H), 7.34–7.44 (m, 20H); ESI-MS for C30H28AuN4S2 found: 705.32 [M]+, calcd: 705.67. HRMS found: 706.0212 [M + H]+ calcd: 705.1367.
Au(I)(3b)2OTf
.
Yield: (0.2 g, 83%). 1H NMR (300 MHz, CDCl3): δ (ppm) 4.33 (s, 8H), 7.10–7.16 (m, 8H), 7.43–7.45 (m, 8H); ESI-MS for C30H24AuF4N4S2 found: 777.28 [M]+, calcd: 777.63, HRMS found: 776.7860, [M]+ calcd: 776.9925.
Au(I)(3c)2OTf
.
Yield: (0.23 g, 88%). 1H NMR (300 MHz, CDCl3): δ (ppm) 4.35 (s, 8H), 7.40 (m, 16H); ESI-MS for C30H24AuCl4N4S2 found: 843.12 [M]+, calcd: 843.45, HRMS found: 842.9692 [M]+, calcd: 843.0836.
[Au(I)(3d)2]OTf
.
Yield: (0.18 g, 75%). 1H NMR (300 MHz, CDCl3): δ (ppm) 4.35 (s, 8H), 7.32 (d, J = 9 Hz, 8H), 7.52 (d, J = 9 Hz, 8H); ESI-MS for C30H24AuBr4N4S2 found: 1020.97 [M]+, calcd: 1021.25 HRMS found: 1020.6602 [M]+, calcd: 1020.7975.
X-ray crystallography studies
The crystallographic data was obtained using a Bruker SMART APEX CCD diffractometer with graphite monochromated Mo–K radiation (λ = 0.7103 Å) at room temperature. The collected frames were processed with the SMART and SAINT programs.46 The structure was solved by direct methods (SHELXL-2014/7) and refined against F2 by full-matrix least-squares analysis. Anisotropic thermal parameters were refined for non-hydrogen atoms. The hydrogen atoms were placed in the ideal positions and refined as riding atoms. Full crystal data collections are given in the ESI as CIF files.† CCDC reference numbers: (Au(I)(3c)2OTf) 1856988.†
Cellular experiments
Cell lines and cell culture conditions.
The cells were routinely incubated in DMEM supplemented with 100 units penicillin/streptomycin and 10% FBS. All the cells were cultured at 37 °C under 5% CO2 in a humidified atmosphere and harvested with trypsin every two or three days. During the experiments, the Au(I) complexes were dissolved in DMSO at an initial concentration of 10 mM and then diluted into the cell culture medium, where the final amount of DMSO did not exceed 1%.
Cell viability assay
The cells were seeded in 96-well flat microplates and incubated for 24 h. After attachment, Au(I) complexes of different concentrations were added and cultivated for 72 h. Then 10 μL of 5 mg mL−1 MTT solution was added to each well and incubated for another 4 h. Finally, the medium was removed and 150 μL DMSO was added to each well to dissolve the formazan complexes.47 The color intensity of the formazan solution, which reflected the cell viability, was measured at 490 nm using a microplate reader. The IC50 value was calculated as the concentration reducing the proliferation of the cells by 50%.
Stability analysis
The stability of the Au(I) complexes was measured from the UV-vis spectra in PBS and DMSO. The Au(I) complexes were dissolved in PBS and DMSO with the same concentration and then the absorbance was detected.
Cell cycle analysis
HeLa cells were incubated in 6-well plates at a density of 4 × 104 cells per well for 24 h. Then the medium was discarded and fresh medium containing Au(I)(3c)2OTf at different concentrations was added. After incubation for 24 h, the cells were harvested, washed with cold PBS and fixed with 70% ethanol overnight at −20 °C. After these treatments, the cells were re-suspended in 0.5 mL PBS including RNase A (1 mg mL−1) and incubated at 37 °C for 35 min and then stained with PI (propidium iodide, 50 μg mL−1) at 4 °C for 15 min. The cell cycle phase distribution was analyzed by using a flow cytometer and Modfit software.
TUNEL experiment
PC3 cells were seeded in a microplate at 2 × 104 cells per well. After incubation for 24 h, the cells were treated with complex Au(I)(3c)2OTf (2.5 μM) overnight. The following operation was according to the manufacturers’ instructions. At the end of incubation, the medium was removed and the cells were washed with PBS three times, and fixed with 4% paraformaldehyde for 30 min. The cells were permeabilized with 0.2% Triton X-100 for 5 min, and washed with PBS twice. Then, the equilibration buffer (100 μL) was added to keep them balanced for 10 min. The cells were covered with 50 μL TdT enzyme in a humidified chamber for 1 h at 37 °C. The nuclei were stained with DAPI, and an enclosed agent was added. Finally, the cells were examined under a confocal microscope.
Annexin V/PI assay
The assay was carried out by following the standard protocol. HeLa cells were cultured in 6-well plates at a density of 1 × 105 cells per well. After attachment, the cells were exposed to fresh media including Au(I)(3c)2OTf of different concentrations for 24 h. Then the cells were collected and re-suspended in 500 μL Annexin-binding buffer containing 5 μL Annexin V and 10 μL PI and stained for 5 min in the absence of light and detected immediately by flow cytometry.
ROS measurement
The generation of ROS in HeLa cells by Au(I)(3c)2OTf was measured by DCFH-DA.40 The HeLa cells were cultured in a 6-well plate at a density of 1 × 105 cells per well. Then, the cells were pre-treated with or without NAC (2 mM) for 30 min, and exposed to Au(I)(3c)2OTf of different concentrations for 24 h. After that, the cells were incubated with 500 nM DCFH-DA in serum-free medium for 30 min. Then the cells were collected, centrifuged and washed with PBS three times. Lastly, the cells were suspended in 1 mL PBS and measured by using a BD C6 flow cytometer.
Mitochondrial membrane potential determination
Rhodamine 123 was utilized to measure the mitochondrial membrane potential.48 The HeLa cells (1 × 105 cells per well) were seeded on a six-well plate. After 24 h, the cells preincubated with or without NAC (2 mM) were treated with different concentrations of Au(I)(3c)2OTf and incubated for another 24 h. Then, the cells were exposed to Rh123 (200 nM) for 30 min in the dark. Finally, the cells were harvested, centrifuged and washed with cold PBS. The fluorescence intensity of the cells was collected in an FL-1 channel using a BD C6 flow cytometer.
Western blot
50 × 104 HeLa cells were seeded to a 100 mm dish. After attachment, the cells were incubated with Au(I)(3c)2OTf in fresh media for different lengths of time (6 h, 12 h, 24 h). The cells were scraped, centrifuged, and washed with cold PBS three times. The cells were lysed by RIPA buffer in the presence of protease inhibitors on ice for 15 min, and centrifuged at 12
000g at 4 °C for 30 min. The supernatants were gathered as total cell extracts. The concentrations of proteins were quantified by using the BCA protein assay reagent kit. 30–50 μg proteins and pre-stained protein markers were separated on 10% sodium dodecyl sulfate–polyacrylamide gel followed by transferring onto activated PVDF membranes. The membranes were blocked with 5% non-fat milk in TBST. After that, the membranes were incubated with the diluted primary antibodies at 4 °C overnight. The blots were further incubated with the secondary antibodies conjugated with horseradish peroxidase at room temperature for 1 h. The immune reactive bands were visualized by the enhanced chemiluminescence. GAPDH was used as the endogenous control. Finally, the protein band results were recorded by an X-ray film.
Abbreviations
ROS | Reactive oxygen species |
MMP | Mitochondrial membrane potential |
DCFH-DA | 2′,7′-Dichlorodihydrofluorescein diacetate |
THT | Tetrahydrothiophene |
NAC |
N-Acetyl cysteine |
MTT | (3-(4,5-Dimethyl-2-thiazolyl)-2,5-diphenyl-2-H-tetrazolium bromide) |
Rh123 | (3,6-Diamino-9-[2-(methoxycarbonyl)-phenyl]xanthylium chloride) |
Conflicts of interest
There are no conflicts to declare.
Acknowledgements
We gratefully acknowledge the financial support of the National Natural Science Foundation of China (No. 21401144, 21673166), Nature Science Foundation of Hubei Province (No. 2013CFB236), the Independent Research Discipline Cross-funding of Wuhan University (2042017kf0215) and Bagui Scholar Program of Guangxi Province (2016).
References
- A. R. De Biasi, J. Villena-Vargas and P. S. Adusumilli, Cisplatin-induced antitumor immunomodulation: A review of preclinical and clinical evidence, Clin. Cancer Res., 2014, 20, 5384–5391 CrossRef CAS.
- Y. Q. Chen and G. Chen, Combined therapeutic effect and molecular mechanisms of metformin and cisplatin in human lung cancer xenografts in nude mice, J. Cancer Res. Ther., 2015, 11, 324–330 CrossRef CAS PubMed.
- N. P. Farrell, Multi-Platinum Anti-cancer Agents. Substitution-inert compounds for tumor selectivity and new targets, Chem. Soc. Rev., 2015, 44, 8773–8785 RSC.
- G. Duan, M. Shi, L. Xie, M. Xu, Y. Wang, H. Yan, Y. Zhuge and X. Zou, Increased glutamine consumption in cisplatin-resistant cells has a negative impact on cell growth, Sci. Rep., 2018, 8, 1–11 CrossRef CAS PubMed.
- H. C. Chuang, M. H. Chou, C. Y. Chien, J. H. Chuang and Y. L. Liu, Triggering TLR3 pathway promotes tumor growth and cisplatin resistance in head and neck cancer cells, Oral Oncol., 2018, 86, 141–149 CrossRef CAS.
- J. F. Lin, Y. C. Lin, T. F. Tsai, H. E. Chen, K. Y. Chou and T. I. S. Hwang, Cisplatin induces protective autophagy through activation of BECN1 in human bladder cancer cells, Drug Des., Dev. Ther., 2017, 11, 1517–1533 CrossRef CAS.
- A. Kosiha, C. Parthiban and K. P. Elango, Metal(II) complexes of bioactive aminonaphthoquinone-based ligand: synthesis, characterization and BSA binding, DNA binding/cleavage, and cytotoxicity studies, J. Coord. Chem., 2018, 71, 1560–1574 CrossRef CAS.
- D. A. Megger, K. Rosowski, C. Radunsky, J. Koesters, B. Sitek and J. Mueller, Structurally related hydrazone-based metal complexes with different antitumor activities variably induce apoptotic cell death, Dalton Trans., 2017, 46, 4759–4767 RSC.
- K. D. Mjos and C. Orvig, Metallodrugs in medicinal inorganic chemistry, Chem. Rev., 2014, 114, 4540–4563 CrossRef CAS.
- P. Zhang and P. J. Sadler, Redox-active metal complexes for anticancer therapy, Eur. J. Inorg. Chem., 2017, 1541–1548 CrossRef CAS.
- A. S. El-Tabl, M. M. Abd El-Wahed, S. E. Abd-El Razek, S. M. El-Gamasy and O. E. A. El-Wahab, Novel metal complexes containing multidentate http://MEP_T_Chk3 base: synthesis, spectroscopic investigation and antitumor activity, J. Chem., Biol. Phys. Sci., 2016, 6, 944–972 CAS.
- M. Pellei, V. Gandin, M. Marinelli, A. Orsetti, F. Del Bello, C. Santini and C. Marzano, Novel triazolium based 11th group NHCs: synthesis, characterization and cellular response mechanisms, Dalton Trans., 2015, 44, 21041–21052 RSC.
- S. Tian, F. M. Siu, S. C. F. Kui, C. N. Lok and C. M. Che, Anticancer gold(I)–phosphine complexes as potent autophagy-inducing agents, Chem. Commun., 2011, 47, 9318–9320 RSC.
- C. T. Lum, R. W. Y. Sun, T. T. Zou and C. M. Che, Gold(III) complexes inhibit growth of cisplatin-resistant ovarian cancer in association with upregulation of proapoptotic PMS2 gene, Chem. Sci., 2014, 5, 1579–1584 RSC.
- C. Zhang, C. Hemmert, H. Gornitzka, O. Cuvillier, M. Zhang and R. W. Y. Sun, Cationic and neutral N-heterocyclic carbene gold(I) complexes: cytotoxicity, NCI-60 screening, cellular uptake, inhibition of mammalian thioredoxin reductase, and reactive oxygen species frmation, ChemMedChem, 2018, 13, 1218–1229 CrossRef CAS.
- O. Dada, G. Sanchez-Sanz, M. Tacke and X. Zhu, Synthesis and aanticancer activity of novel NHC-gold(I)–sugar complexes, Tetrahedron Lett., 2018, 59, 2904–2908 CrossRef CAS.
- Ö. Karaca, V. Scalcon, S. M. Meier-Menches, R. Bonsignore, J. M. J. L. Brouwer, F. Tonolo, A. Folda, M. P. Rigobello, F. E. Kühn and A. Casini, Characterization of hydrophilic
gold(I) N-heterocyclic carbene (NHC) complexes as potent TrxR inhibitors using biochemical and mass spectrometric approaches, Inorg. Chem., 2017, 56, 14237–14250 CrossRef.
- K. Yan, C. N. Lok., K. Bierla and C. M. Che, Gold(I) Complex of N,N′-disubstituted cyclic thiourea with in vitro and in vivo anticancer properties – potent tight-binding inhibition of thioredoxin reductase, Chem. Commun., 2010, 46, 7691–7693 RSC.
- J. F. Arambula, R. McCall, K. J. Sidoran, D. Magda, N. A. Mitchell, C. W. Bielawski, V. M. Lynch, J. L. Sessler and K. Arumugam, Targeting antioxidant pathways with ferrocenylated -heterocyclic carbene supported gold(I) complexes in A549 lung cancer cells, Chem. Sci., 2016, 7, 1245–1256 RSC.
- C. Schmidt, B. Karge, R. Misgeld, A. Prokop, R. Franke, M. Broenstrup and I. Ott, Gold(I) NHC complexes: antiproliferative activity, cellular uptake, inhibition of mammalian and bacterial thioredoxin reductases, and Gram-positive directed antibacterial effects, Chem. – Eur. J., 2017, 23, 1869–1880 CrossRef CAS.
- S. Adhikari, O. Hussain, R. M. Phillips, W. Kaminsky and M. R. Kollipara, Neutral and cationic half-sandwich arene d6 metal complexes containing pyridyl and pyrimidyl thiourea ligands with interesting bonding modes: synthesis, structural and anti-cancer studies, Appl. Organomet. Chem., 2018, 32, 4476 CrossRef.
- U. Solmaz, I. Gumus, G. Binzet, O. Celik, G. K. Balci, A. Dogen and H. Arslan, Synthesis, characterization, crystal structure, and antimicrobial studies of novel thiourea derivative ligands and their platinum complexes, J. Coord. Chem., 2018, 71, 200–218 CrossRef CAS.
- R. Vivas-Reyes, E. Espinosa-Fuentes, J. Forigua, A. Arias, R. Gaitan and E. Arguello, Theoretical study of a series of N-(n-propyl)-N'-(para-R-benzoyl)thioureas with trans[Pt(py)2Cl2] through chemistry reactivity descriptors based on density functional Theory, THEOCHEM, 2008, 862, 92–97 CrossRef CAS.
- R. Reshmy, K. K. Thomas and A. Sulekha,
N-Benzoyl-N′N′-disubstituted thioureas-a new binary accelerator system and its effect of nucleophilicity in sulfur vulcanization of natural rubber, J. Appl. Polym. Sci., 2012, 124, 978–984 CrossRef CAS.
- M. M. Habtu, S. A. Bourne, K. R. Koch and R. C. Luckay, Competitive bulk liquid membrane transport and solvent extraction of some transition and post-transition metal ions using acylthiourea ligands as ionophores, New J. Chem., 2006, 30, 1155–1162 RSC.
- R. K. Sharma, Y. N. Chouryal, S. Nigam, J. Saravanakumar, S. Barik and P. Ghosh, Tuning the crystal phase and morphology of the photoluminescent indium sulphide nanocrystals and their adsorption-based catalytic and photocatalytic applications, ChemistrySelect, 2018, 3, 8171–8182 CrossRef CAS.
- M. C. Ford and P. S. Ho, Computational tools to model halogen bonds in medicinal chemistry, J. Med. Chem., 2016, 59, 1655–1670 CrossRef CAS.
- R. Wilcken, M. O. Zimmermann, A. Lange, A. C. Joerger and F. M. Boeckler, Principles and applications of halogen bonding in medicinal chemistry and chemical biology, J. Med. Chem., 2013, 56, 1363–1388 CrossRef CAS.
- A. R. Katritzky, W. Q. Fan and C. Fu, Novel method for the synthesis of symmetrical vicinal tertiary and secondary diamines, J. Org. Chem., 1990, 55, 3209–3213 CrossRef CAS.
- D. Yang, Y. C. Chen and N. Y. Zhu, Sterically bulky thioureas as air and moisture stable ligands for Pd-catalyzed http://MEP_T_Chk5 reactions of aryl halides, Org. Lett., 2004, 6, 1577–1580 CrossRef CAS.
- M. Premanathan, K. Karthikeyan, K. Jeyasubramanian and G. Manivannan, Selective toxicity of ZnO nanoparticles toward Gram-positive bacteria and cancer cells by apoptosis through lipid peroxidation, Nanomedicine, 2011, 7, 184–192 CrossRef CAS.
- S. F. Mujtaba, A. Dwivedi, N. Yadav, R. Ch, H. N. Kushwaha, M. K. R. Mudiam, G. Singh and R. S. Ray, Superoxide mediated photomodification
and DNA damage induced apoptosis by benz(a)anthracene via mitochondrial mediated pathway, J. Photochem. Photobiol., B, 2015, 142, 92–102 CrossRef CAS.
- X. M. Hu, T. Hirano and K. Oka, Arsenic trioxide induces apoptosis in Cells of MOLT-4 and its daunorubicin-resistant cell line via depletion of intracellular glutathione, disruption of mitochondrial membrane potential and activation of caspase-3, Cancer Chemother. Pharmacol., 2003, 52, 47–58 CrossRef CAS.
- C. M. Palmeira, A. J. M. Moreno, V. M. C. Madeira and K. B. Wallace, Continuous monitoring of mitochondrial membrane potential in hepatocyte cell suspensions, J. Pharmacol. Toxicol., 1996, 35, 35–43 CrossRef CAS.
- P. Ludovico, F. Rodrigues, A. Almeida, T. Silva, A. Barrientos and M. Côrte-Real, Cytochrome C release and mitochondria involvement in programmed cell death induced by acetic acid in saccharomyces cerevisiae, Mol. Biol. Cell, 2002, 13, 2598–2606 CrossRef CAS.
- S. Emami, H. Ghourchian, A. Fau-Divsalar and A. Divsalar, Release of Cyt C from the model membrane due to conformational change induced by anticancer palladium complex, Int. J. Biol. Macromol., 2011, 48, 243–248 CrossRef CAS.
- R. Kumar, J. Han, H. J. Lim, W. X. Ren, J. Y. Lim, J. H. Kim and J. S. Kim, Mitochondrial induced and self-monitored intrinsic apoptosis by antitumor theranostic prodrug: in vivo imaging and precise cancer treatment, J. Am. Chem. Soc., 2014, 136, 17836 CrossRef CAS.
- J. C. Martinou, S. Desagher and B. Antonsson, Cytochrome C release from mitochondria: all or nothing, Nat. Cell Biol., 2000, 2, 41–43 CrossRef.
- S. Karmakar, N. L. Banik and S. K. Ray, Curcumin suppressed anti-apoptotic signals and activated cysteine proteases for apoptosis in human malignant glioblastoma U87MG cells, Neurochem. Res., 2007, 32, 2103–2113 CrossRef CAS.
- X. Y. Fan, X. Y. Chen, Y. J. Liu, H. M. Zhong, F. L. Jiang and Y. Liu, Oxidative stress-mediated intrinsic apoptosis in human promyelocytic leukemia HL-60 cells induced by organic arsenicals, Sci. Rep., 2016, 6, 29865 CrossRef CAS.
- Y. You and W. Nam, Designing photoluminescent molecular probes for singlet oxygen, hydroxyl radical, and iron-oxygen species, Chem. Sci., 2014, 5, 4123–4135 RSC.
- G. Y. Liou and P. Storz, Reactive oxygen species in cancer, Free Radical Res., 2010, 44, 479–496 CrossRef CAS.
- R. Uson, A. Laguna and M. Laguna, (Tetrahydrothiophene) gold(I) or gold(III) complexes, Inorg. Synth., 1989, 26, 85–91 CAS.
- A. J. Arduengo, R. Krafczyk and R. Schmutzler, Imidazolylidenes, imidazolinylidenes and imidazolidines, Tetrahedron, 1999, 55, 14523–14534 CrossRef CAS.
- C. Tubaro, M. Baron, M. Costante, M. Basato, A. Biffis, A. Gennaro, A. A. Isse, C. Graiff and G. Accorsi, Dinuclear gold(I) complexes with propylene bridged N-heterocyclic dicarbene ligands: synthesis, structures, and trends in reactivities and properties, Dalton Trans., 2013, 42, 10952–10963 RSC.
-
SMART and SAINT, Area detector software package and SAX area detector integration program, Bruker analytical X-ray, Madison, WI, USA, 1997 Search PubMed.
- K. Mitra, A. Shettar, P. Kondaiah and A. R. Chakravarty, Biotinylated platinum(II) ferrocenylterpyridine complexes for targeted photoinduced cytotoxicity, Inorg. Chem., 2016, 55, 5612–5622 CrossRef CAS.
- Y. Li, G. F. Liu, C. P. Tan, L. N. Ji and Z. W. Mao, Antitumor properties and mechanisms of mitochondria-targeted Ag(I) and Au(I) complexes containing N-heterocyclic carbenes derived from cyclophanes, Metallomics, 2014, 6, 1460–1468 RSC.
Footnote |
† Electronic supplementary information (ESI) available. CCDC 1856988 (Au(I)(3c)2OTf). For ESI and crystallographic data in CIF or other electronic format see DOI: 10.1039/c9mt00232d |
|
This journal is © The Royal Society of Chemistry 2020 |
Click here to see how this site uses Cookies. View our privacy policy here.