DOI:
10.1039/C9NA00682F
(Paper)
Nanoscale Adv., 2020,
2, 332-339
Effects of methylamine doping on the stability of triple cation (FA0.95−xMAxCs0.05)PbI3 single crystal perovskites†
Received
28th October 2019
, Accepted 13th November 2019
First published on 15th November 2019
Abstract
Despite being promising photovoltaic materials, widespread use of organic–inorganic halide perovskite materials is still hindered by their undesirable stability. To cope with this challenge, methylamine (MA) is doped into triple cation perovskite single crystals of (FA0.95−xMAxCs0.05)PbI3, and cesium-containing triple cation perovskite single crystals with five different MA molar ratios (x = 0, 0.05, 0.10, 0.15, and 0.20) are synthesized and characterized. Among them, (FA0.8MA0.15Cs0.05)PbI3 shows high stability against water-oxygen and light for 60 days, and the thermal decomposition temperature of (FA0.8MA0.15Cs0.05)PbI3 reaches as high as 305 °C. Besides, the carrier lifetime of (FA0.8MA0.15Cs0.05)PbI3 is up to 5.957 μs, which remains as 5.646 μs (95%) after 60 days of light illumination. This work studies the stability of perovskite single crystals based on (FA0.95−xMAxCs0.05)PbI3 compositions and provides a reference for the discovery of novel perovskite photovoltaic devices with high efficiency and long-term stability.
Introduction
Perovskite solar cells (PSCs) have received wide attention due to their easy preparation1–9 and ever-increasing photoelectric conversion efficiency (PCE).10–14 Currently, the certified highest PCE of PSCs has reached 25.2%.15 The high light absorption16 and tunable cation components17–19 of perovskites make them promising for application in PSCs. However, compared with commercial silicon-based solar cells (with more than 20 years of service life),20 the undesirable stability of PSCs still hinders their rapid development and widespread use. The performance of perovskite photovoltaic devices was threatened by light, heat and water-oxygen,21–25 and the main reason is the instability of the perovskite material itself. For example, MAPbI3 perovskite was reported to be sensitive to moisture26–28 and temperature.29–31 FAPbI3 (ref. 32 and 33) perovskite easily decomposes into yellow phase δ-FAPbI3 at room temperature, which leads to the loss of optical properties. Snaith34 reported that replacing some of the formamidinium (FA) in FAPbI3 with methylamine (MA), forming (FA/MA)PbI3 perovskite, can efficiently inhibit its phase separation and enhance the stability of the perovskite. Besides, doping an appropriate amount of cesium (Cs) ions can reduce trap state density and further improve the thermal stability of (FA/MA)PbI3 perovskite. The introduction of the Cs ion can inhibit the formation of yellow phase FAPbI3 due to its smaller radius. However, excessive doping of Cs will lead to the decrease of crystal size and induce phase decomposition forming CsPbI3.32,35–37 According to the literature reports, doping a trace amount of Cs ions would be optimal for (FA/MA)PbI3 perovskite.
In addition, most of the studies on PSCs are based on polycrystalline films with pores, grain boundaries and defects,38–42 resulting in severe hysteresis and environmental sensitivity,43 which are detrimental to electron transport in perovskites and long-term stability44 of PSCs. Nowadays, single crystal perovskites have drawn a lot of attention in the development of PSCs,45 and perovskites with low defect states and suitable optical band gaps benefit both the photovoltaic performance and long-term stability of PSCs. Compared with polycrystalline thin films, FAPbI3 single crystal perovskite has good thermal resistance,46 but the optical properties and carrier drift of the FAPbI3 single crystal material are seriously affected by its easy decomposition into yellow phase δ-FAPbI3.47 MAPbI3 single crystal perovskite is the most widely used because of its excellent photoelectric performance, and the formation of (FA/MA)PbI3 perovskite can effectively improve the structural stability.48 And after doping a small amount of Cs ion into the FAPbI3 single crystal perovskite, the density of defect states is reduced.49 Thus, doping a trace amount of Cs ions into (FA/MA)PbI3 single crystal perovskite would lead to better performance than the polycrystalline counterpart.
In this work, a series of mixed cation perovskites (FA0.95−xMAxCs0.05)PbI3 were prepared as single crystals, and a systematic study of their long-term stability, including water-oxygen, light and thermal stability was carried out, respectively. Related optimization effects were investigated and discussed in this paper. And the optimal cation doping ratio was determined based on the characterization of the properties of the materials. Cesium-containing triple cation perovskite single crystals with excellent stability and a long carrier lifetime were obtained, and they can be used as light absorption layers in high PCE and stable PSCs.
Experimental section
Materials
CH3NH3I (MAI, ≥ 99.5%), NH2CH
NH2I (FAI, ≥99.5%) and PbI2 (>99.99%) were purchased from Xi’an Polymer Light Technology Corp. CsI (99.999%) was purchased from Shanghai Mater Win New Materials Co., Ltd. The solvent of γ-butyrolactone (GBL, 99%) was purchased from Aladdin. All the materials were used as received.
Growth of perovskite single crystals
A series of (FA0.95−xMAxCs0.05)PbI3 single crystals were prepared using the inverse temperature crystallization (ITC) method. (FA0.95Cs0.05)PbI3 and (FA0.9MA0.05Cs0.05)PbI3 can be acquired from the 1.2 M clear GBL solution, and (FA0.85MA0.1Cs0.05)PbI3, (FA0.8MA0.15Cs0.05)PbI3 and (FA0.75MA0.2Cs0.05)PbI3 can be acquired from the 1.6 M clear GBL solution. Crystal samples were gradually heated on a thermal stirrer (IKA C-MAG HS 7) to find the complete dissolution temperature and the crystallization temperature, and during this process the solution will change from a clear yellow solution to a black suspension, as shown in ESI Video 1.† The growth temperature of each crystal composition can be precisely and efficiently determined by this method. And the perovskite samples were slowly heated from the complete melting temperature to the crystallization temperature with 1 °C/30 min. Finally, the perovskite samples were sintered at the crystallization temperature for 3 hours, and all the parameters are listed in Table 1.
Table 1 The growth parameters of perovskite single crystal systems
Samples |
Solution concentration (mol L−1) |
Temperature |
(FA0.95Cs0.05)PbI3 |
1.2 |
70–120 °C |
(FA0.9MA0.05Cs0.05)PbI3 |
1.2 |
50–100 °C |
(FA0.85MA0.1Cs0.05)PbI3 |
1.6 |
75–125 °C |
(FA0.8MA0.15Cs0.05)PbI3 |
1.6 |
60–110 °C |
(FA0.75MA0.2Cs0.05)PbI3 |
1.6 |
70–120 °C |
Perovskite single crystal alloy characterization
EDS mapping of the perovskite single crystal powders was performed using a field-emission scanning electron microscope (SEM) (Jeol JEM 6510LV). The X-ray diffraction (XRD) spectra were obtained using a Bruker-AXS D8 Advance X-ray diffractometer. Absorbance was tested using a UV-vis spectrophotometer (UV 3600, Shimadzu). Steady-state photoluminescence (PL) experiments were performed using an FLSP920 spectrometer (Edinburgh Instruments Ltd), and transient PL measurements were carried out using an FLS 980E fluorometer. The PL spectra were measured with a 485 nm laser as the excitation light source. Thermogravimetric Analysis (TGA) was carried out on a Mettler-TGA1 to characterize the thermal stability of the samples. And the long-term stability, water and oxygen stability, and light and thermal stability of the perovskite single crystal samples were evaluated, respectively. For the water and oxygen stability tests, the perovskite single crystal powders were put in a brown desiccator with a humidity of 20% and characterized intermittently for 60 days. For the light stability tests, the perovskite single crystal powders were stored in a self-made sealed box filled with argon, and kept in an ultraviolet aging box (Q-Lab, USA) for 60 days to conduct the “Day and Night” simulation test. After 12 hour illumination, the samples were kept in the dark for 12 hours, and the above-mentioned treatments were repeated. The device performances were measured under ambient conditions (15% < relative humidity (RH) < 60%) every time.
Results and discussion
Cs containing triple cation (FA0.95−xMAxCs0.05)PbI3 perovskite single crystals were synthesized as shown in Fig. 1. EDS mapping was carried out on the single crystal powders (Fig. 2), and the uniform distribution of the Cs element indicates the successful doping of the Cs element into the (FA0.8MA0.15Cs0.05)PbI3 perovskite. In order to analyze the crystal structure of the perovskites, the powder samples were subjected to XRD tests. As shown in Fig. 3a, diffraction peaks at 11.6° were detected from (FA0.95Cs0.05)PbI3, (FA0.9MA0.05Cs0.05)PbI3 and (FA0.85MA0.1Cs0.05)PbI3 samples, and can be assigned to the (010) plane of the yellow phase δ-FAPbI3. And the 11.6° peak disappeared when the MA doping ratio reached 0.15, which suggests that yellow phase δ-FAPbI3 formation can be efficiently inhibited with the addition of the MA cation (Fig. 3a). And the strong peaks at 14.1°, 28.3° and 31.8°, assigned to (110), (220) and (310) planes, proved the successful synthesis of cesium-containing triple cation perovskite single crystals. The magnified XRD pattern of the (110) plane is displayed in Fig. 3b. Obviously, all diffraction peaks demonstrate a shift to a high degree, and this is due to the smaller radius of the MA (2.17 Å) ions compared to the FA (2.53 Å) ions and the partial replacement of the FA ions with MA ions in the perovskite structure, causing lattice shrinking.50
 |
| Fig. 1 Photographs of the series of (FA0.95−xMAxCs0.05)PbI3 perovskite single crystals: (a) (FA0.95Cs0.05)PbI3, (b) (FA0.9MA0.05Cs0.05)PbI3, (c) (FA0.85MA0.1Cs0.05)PbI3, (d) (FA0.8MA0.15Cs0.05)PbI3 and (e) (FA0.75MA0.2Cs0.05)PbI3. | |
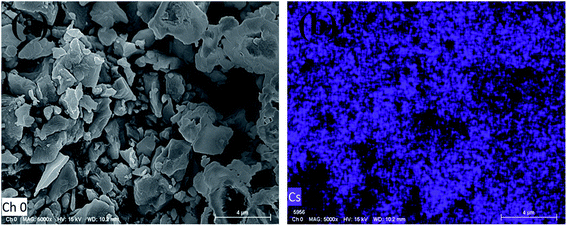 |
| Fig. 2 (a) FESEM image of perovskite single crystals of (FA0.8MA0.15Cs0.05)PbI3. (b) EDS mapping of the Cs element. | |
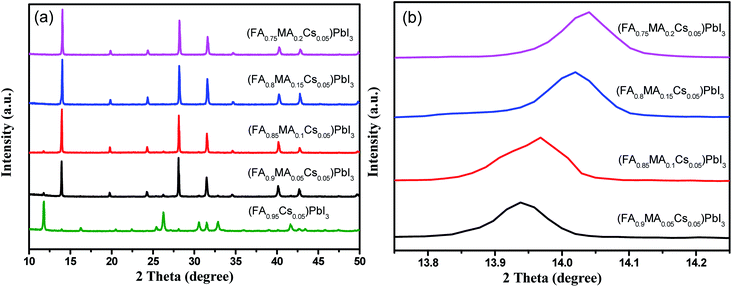 |
| Fig. 3 XRD patterns of (a) (FA0.95Cs0.05)PbI3, (FA0.9MA0.05Cs0.05)PbI3, (FA0.85MA0.1Cs0.05)PbI3, (FA0.8MA0.15Cs0.05)PbI3 and (FA0.75MA0.2Cs0.05)PbI3, and (b) magnified angles on the (110) plane. | |
Fig. 4 shows the UV-vis absorption spectra of the perovskite single crystals. A single crystal perovskite with high absorption at 830 nm is obtained. With MA ion doping, the absorption intensity of the perovskite single crystal is significantly increased, which could be the reason for the formation of a black α-phase perovskite (as shown in Fig. 3a) resulting in higher light absorption.51 According to the absorption spectra, the band gaps of the perovskite single crystals of (FA0.9MA0.05Cs0.05)PbI3, (FA0.85MA0.1Cs0.05)PbI3, (FA0.8MA0.15Cs0.05)PbI3 and (FA0.75MA0.2Cs0.05)PbI3 are estimated to be 1.42 eV, 1.44 eV, 1.45 eV and 1.455 eV respectively (Fig. 5a–d). With the increase of the molar ratio of MA cations, the band gaps of the perovskite single crystals are slightly widened, which can be attributed to the smaller radius of the MA cation compared to that of the FA cation.52 Doping a smaller quantity of MA into the perovskite crystal structure shrinks the crystal lattice, according to the Bragg equation, and the XRD peaks shift to the right, corresponding to Fig. 3b, and the bandgap is widened.53,54
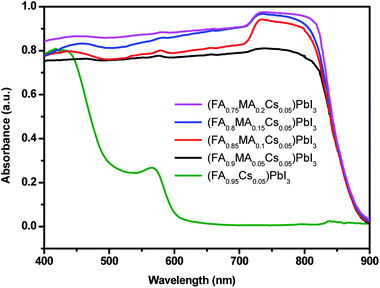 |
| Fig. 4 UV-vis absorption spectra of (FA0.95Cs0.05)PbI3, (FA0.9MA0.05Cs0.05)PbI3, (FA0.85MA0.1Cs0.05)PbI3, (FA0.8MA0.15Cs0.05)PbI3 and (FA0.75MA0.2Cs0.05)PbI3. | |
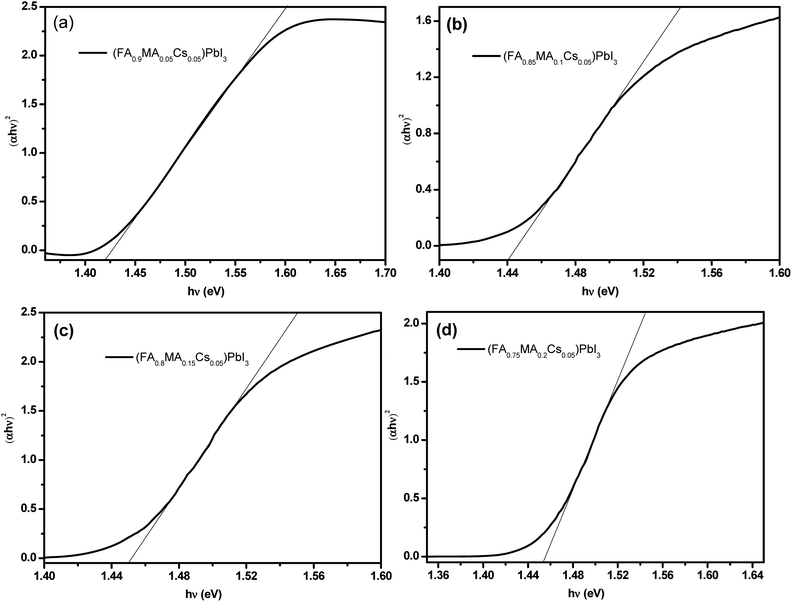 |
| Fig. 5 Tauc plots of the five compositions of (a) (FA0.9MA0.05Cs0.05)PbI3 (1.42 eV), (b) (FA0.85MA0.1Cs0.05)PbI3 (1.44 eV), (c) (FA0.8MA0.15Cs0.05)PbI3 (1.45 eV) and (d) (FA0.75MA0.2Cs0.05)PbI3 (1.455 eV). | |
Emission peaks of single crystals of (FA0.9MA0.05Cs0.05)PbI3, (FA0.85MA0.1Cs0.05)PbI3, (FA0.8MA0.15Cs0.05)PbI3 and (FA0.75MA0.2Cs0.05)PbI3 at 830 nm are presented in Fig. 6a. (FA0.8MA0.15Cs0.05)PbI3 with a 15% molar ratio of MA exhibits the highest PL intensity, indicating the least occurrence of nonradiative recombination.46 Moreover, transient PL measurements (Fig. 6b) are carried out to characterize the carrier lifetime of the perovskite crystals. All samples used for testing were fresh crystals. The average carrier lifetime of (FA0.8MA0.15Cs0.05)PbI3 is as long as 5.957 μs, which is the highest among the average carrier lifetimes of the other three perovskite single crystal samples (5.030 μs of (FA0.75MA0.2Cs0.05)PbI3, 4.120 μs of (FA0.85MA0.1Cs0.05)PbI3 and 3.637 μs of (FA0.9MA0.05Cs0.05)PbI3, and all parameters are summarized in Table 2).
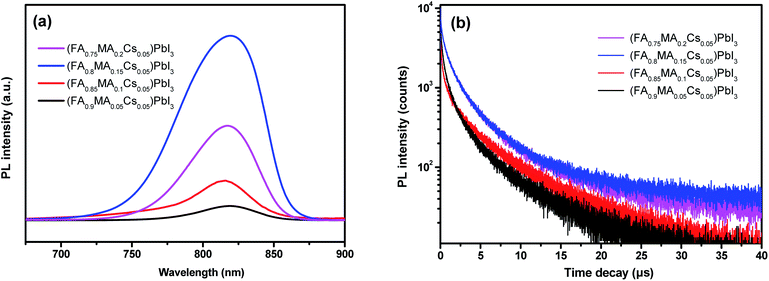 |
| Fig. 6 The perovskite single crystals of (FA0.9MA0.05Cs0.05)PbI3, (FA0.85MA0.1Cs0.05)PbI3, (FA0.8MA0.15Cs0.05)PbI3 and (FA0.75MA0.2Cs0.05)PbI3 with (a) steady-state PL spectra and (b) transient photoluminescence spectra. | |
Table 2 The parameters of the time-resolved PL spectra of the perovskite single crystals: (FA0.9MA0.05Cs0.05)PbI3, (FA0.85MA0.1Cs0.05)PbI3, (FA0.8MA0.15Cs0.05)PbI3 and (FA0.75MA0.2Cs0.05)PbI3
Samples |
τ
ave [μs] |
τ
1 [μs] |
τ
2 [μs] |
(FA0.9MA0.05Cs0.05)PbI3 |
3.637 |
5.336 |
1.259 |
(FA0.85MA0.1Cs0.05)PbI3 |
4.120 |
6.092 |
1.523 |
(FA0.8MA0.15Cs0.05)PbI3 |
5.957 |
8.234 |
1.808 |
(FA0.75MA0.2Cs0.05)PbI3 |
5.030 |
7.098 |
1.617 |
In order to evaluate the water-oxygen stability of the perovskite single crystals, we placed the perovskite powders in a brown dryer and stored them in a dark environment, exposed to water (20% humidity) and oxygen. It is found that the (FA0.9MA0.05Cs0.05)PbI3 sample gradually turns yellow, and (FA0.85MA0.1Cs0.05)PbI3, (FA0.8MA0.15Cs0.05)PbI3 and (FA0.75MA0.2Cs0.05)PbI3 samples still remain black. After 60 days of water-oxygen treatment, phase characterization was carried out on all samples by XRD tests (Fig. 7). The peaks at 11.6° suggest δ-FAPbI3 phase separation from (FA0.9MA0.05Cs0.05)PbI3 and (FA0.85MA0.1Cs0.05)PbI3 samples. By comparison, (FA0.8MA0.15Cs0.05)PbI3 and (FA0.75MA0.2Cs0.05)PbI3 samples still maintained their pure perovskite single crystal structure. With the incorporation of 15% and 20% MA (molar ratio), the long-term stability of the perovskite samples was efficiently enhanced. Doping a smaller quantity of MA into the perovskite crystal structure enables the promotion of the interaction between the cation and the inorganic octahedron, which reduces the defect state of perovskite crystals thus increasing the long-term water-oxygen stability of the crystals.20
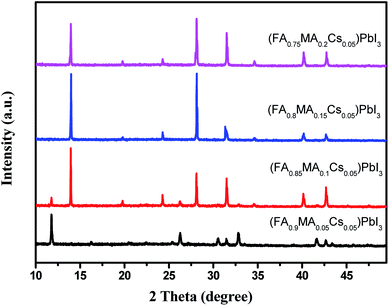 |
| Fig. 7 XRD patterns of (FA0.9MA0.05Cs0.05)PbI3, (FA0.85MA0.1Cs0.05)PbI3, (FA0.8MA0.15Cs0.05)PbI3 and (FA0.75MA0.2Cs0.05)PbI3 after placing them for 60 days in contact with water-oxygen. | |
To investigate the long-term light stability of the perovskite crystals, the samples were placed in a self-made sealed transparent box filled with argon gas and under simulated illumination of day and night. After 60 days of “Day and Night” simulation test, the XRD characterization (Fig. 8a) reveals that (FA0.9MA0.05Cs0.05)PbI3 and (FA0.85MA0.1Cs0.05)PbI3 samples undergo phase decomposition while (FA0.8MA0.15Cs0.05)PbI3 and (FA0.75MA0.2Cs0.05)PbI3 show excellent light stability. The transient PL spectra of the pre-treated and post-treated (FA0.8MA0.15Cs0.05)PbI3 perovskites are presented in Fig. 8b. After 60 days of light illumination, the post-treated (FA0.8MA0.15Cs0.05)PbI3 sample still maintains a long carrier lifetime of 5.646 μs, which is 95% of the carrier lifetime of the pre-treated (FA0.8MA0.15Cs0.05)PbI3 sample (5.957 μs). The above results show that the illumination causes only a small amount of defects in the (FA0.8MA0.15Cs0.05)PbI3 perovskite crystal without phase separation.
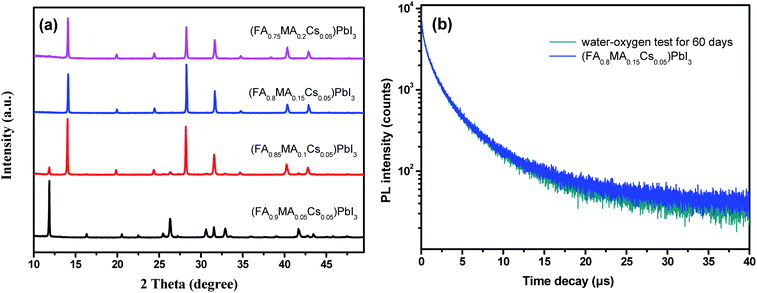 |
| Fig. 8 (a) XRD patterns of (FA0.9MA0.05Cs0.05)PbI3, (FA0.85MA0.1Cs0.05)PbI3, (FA0.8MA0.15Cs0.05)PbI3 and (FA0.75MA0.2Cs0.05)PbI3 after illumination for 60 days. (b) Transient photoluminescence spectra of (FA0.8MA0.15Cs0.05)PbI3 and the crystal under illumination for 60 days. | |
The thermal stability of the perovskite crystals was investigated by TGA measurements. After the TGA test on (FA0.8MA0.15Cs0.05)PbI3 (fresh crystal), two compositions of perovskite single crystals, namely (MA0.95Cs0.05)PbI3 and (FA0.8MA0.2)PbI3, were synthesized to investigate the effect of FA and Cs cation doping on the thermal stability of the perovskite. The TGA spectrum of (FA0.8MA0.15Cs0.05)PbI3 is shown in Fig. 9a; the thermal decomposition temperature is 305 °C, which is close to that of pure FAPbI3, and the weight loss of 20% between 305 and 360 °C can be attributed to the removal of organic components.46,55,56 Lead halide is evaporated above 470 °C. By comparison, the removal of the organic component from MAPbI3 occurs at 150–200 °C.57–59 And the initial decomposition temperatures (Fig. 9b and c) of (MA0.95Cs0.05)PbI3 and (FA0.8MA0.2)PbI3 are 290 °C and 300 °C, respectively. Compared to the perovskite crystals of (MA0.95Cs0.05)PbI3 and (FA0.8MA0.2)PbI3, the better thermal stability of (FA0.8MA0.15Cs0.05)PbI3 can be explained by the strong interaction between the cation and the inorganic octahedron. The initial decomposition temperature of the crystal without the FA cation decreased by 15 °C and the initial decomposition temperature without the Cs cation decreased by only 5 °C, which indicate that the FA cation has a stronger influence on the thermal stability of the crystal than the Cs cation, and this provides a reference for the synthesis of novel perovskite composition with excellent thermal stability.
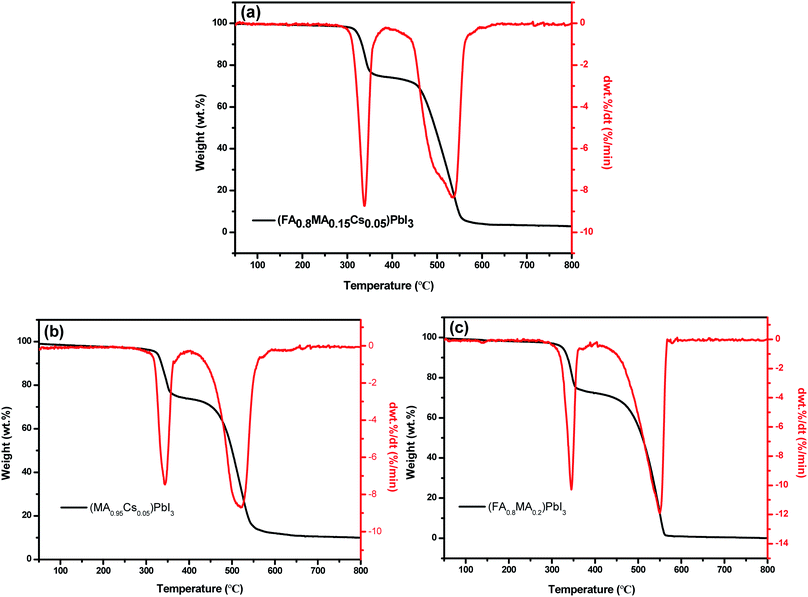 |
| Fig. 9 TGA and DTA curves of perovskite single crystals: (a) (FA0.8MA0.15Cs0.05)PbI3, (b) (MA0.95Cs0.05)PbI3 and (c) (FA0.8MA0.2)PbI3. | |
Conclusions
In summary, we prepared a series of compositions of perovskite single crystals. Among them, (FA0.8MA0.15Cs0.05)PbI3 perovskite maintains long-term stability after 60 days of water-oxygen and light treatment. The MA ion doping enabled a strong interaction between the cation and the inorganic octahedron, which efficiently improved the structural stability of the perovskite samples. The band gap of (FA0.8MA0.15Cs0.05)PbI3 is 1.45 eV, which is very close to the optimal optical absorption band gap (1.1–1.4 eV); the carrier lifetime of (FA0.8MA0.15Cs0.05)PbI3 perovskite single crystals reaches 5.957 μs, and the lifetime is still 5.646 μs after 60 days of light illumination. The thermal decomposition temperature of (FA0.8MA0.15Cs0.05)PbI3 is as high as 305 °C. This study has provided a reference for the synthesis of perovskite single crystal materials with improved long-term stability and carrier lifetime, which are promising to be applied in high-efficiency and stable perovskite solar cells.
Conflicts of interest
There are no conflicts to declare.
Acknowledgements
This research was financially supported by the National Natural Science Foundation of China (51572072 and 5170021087) and the China Postdoctoral Science Foundation (2017M622384).
References
- M. M. Lee, T. JoëL, M. Tsutomu, T. N. Murakami and H. J. Snaith, Science, 2012, 338, 643–647 CrossRef CAS.
- J. Burschka, N. Pellet, S. J. Moon, R. Humphry-Baker, P. Gao, M. K. Nazeeruddin and M. Grätzel, Nature, 2013, 499, 316 CrossRef CAS.
- L. Mingzhen, M. B. Johnston and H. Snaith, Nature, 2013, 501, 395 CrossRef.
- J. Nam Joong, N. Jun Hong, K. Young Chan, Y. Woon Seok, R. Seungchan and S. Sang, Nat. Mater., 2014, 13, 897–903 CrossRef.
- N. J. Jeon, J. H. Noh, W. S. Yang, Y. C. Kim, S. Ryu, J. Seo and S. Seok, Nature, 2015, 517, 476 CrossRef CAS.
- Y. Woon Seok, N. Jun Hong, J. Nam Joong, K. Young Chan, R. Seungchan, S. Jangwon and S. Seok, Science, 2015, 348, 1234 CrossRef.
- D. Bi, C. Yi, J. Luo, J. D. Décoppet, Z. Fei, S. M. Zakeeruddin, L. Xiong, A. Hagfeldt and M. Grätzel, Nat. Energy, 2016, 1, 16142 CrossRef CAS.
- W. S. Yang, B.-W. Park, E. H. Jung, N. J. Jeon, Y. C. Kim, U. L. Dong, S. S. Shin, J. Seo, E. K. Kim and J. H. Noh, Science, 2017, 356, 1376–1379 CrossRef CAS PubMed.
- D. Luo, W. Yang, Z. Wang, A. Sadhanala, Q. Hu, R. Su, R. Shivanna, G. F. Trindade, J. F. Watts and Z. Xu, Science, 2018, 360, 1442–1446 CrossRef CAS.
- A. Mei, X. Li, L. Liu, Z. Ku, T. Liu, Y. Rong, M. Xu, M. Hu, J. Chen and Y. Yang, Science, 2014, 345, 295–298 CrossRef CAS.
- H. Chen, F. Ye, W. Tang, J. He, M. Yin, Y. Wang, F. Xie, E. Bi, X. Yang and M. Grätzel, Nature, 2017, 550, 92 CrossRef CAS.
- H. Tan, A. Jain, O. Voznyy, X. Lan, G. D. A. Fp, J. Z. Fan, R. Quintero-Bermudez, M. Yuan, B. Zhang and Y. Zhao, Science, 2017, 355, 722–726 CrossRef CAS.
- Y. Rong, Y. Hu, A. Mei, H. Tan, M. I. Saidaminov, S. I. Seok, M. D. McGehee, E. H. Sargent and H. Han, Science, 2018, 361, 8235 CrossRef PubMed.
- S. Lin, H. Wang, X. Zhang, D. Wang, D. Zu, J. Song, Z. Liu, Y. Huang, K. Huang and N. Tao, Nano Energy, 2019, 62, 111–116 CrossRef CAS.
-
https://www.nrel.gov/pv/device-performance.html
.
- M. A. Green, A. Ho-Baillie and H. J. Snaith, Nat. Photonics, 2014, 8, 506–514 CrossRef CAS.
- F. Hao, C. C. Stoumpos, D. H. Cao, R. P. H. Chang and M. G. Kanatzidis, Nat. Photonics, 2017, 8, 489–494 CrossRef.
- J. Kim, H. P. Kim, M. A. M. Teridi, A. R. B. M. Yusoff and J. Jin, Sci. Rep., 2016, 6, 37378 CrossRef CAS.
- X. Wang, Y. Cui, T. Li, M. Lei, J. Li and Z. Wei, Adv. Opt. Mater., 2019, 7, 1801274 CrossRef.
- L. Chen, Y. Y. Tan, Z. X. Chen, T. Wang, S. Hu, Z. A. Nan, L.-Q. Xie, Y. Hui, J.-X. Huang and C. Zhan, J. Am. Chem. Soc., 2019, 141, 1665–1671 CrossRef CAS PubMed.
- Z. Gan, F. Zheng, W. Mao, C. Zhou, W. Chen, U. Bach, P. Tapping, T. W. Kee, J. A. Davis and B. Jia, Nanoscale, 2019, 11, 14676–14683 RSC.
- Q. Guo, F. Yuan, B. Zhang, S. Zhou, J. Zhang, Y. Bai, L. Fan, T. Hayat, A. Alsaedi and Z. a. Tan, Nanoscale, 2019, 11, 115–124 RSC.
- Y. Li, L. Zhao, M. Xiao, Y. Huang, B. Dong, Z. Xu, L. Wan, W. Li and S. Wang, Nanoscale, 2018, 10, 22003–22011 RSC.
- E. S. Parrott, J. B. Patel, A.-A. Haghighirad, H. J. Snaith, M. B. Johnston and L. M. Herz, Nanoscale, 2019, 11, 14276–14284 RSC.
- M. Xiao, L. Zhao, M. Geng, Y. Li, B. Dong, Z. Xu, L. Wan, W. Li and S. Wang, Nanoscale, 2018, 10, 12141–12148 RSC.
- J. Yang, B. D. Siempelkamp, D. Liu and T. L. Kelly, ACS Nano, 2015, 9, 1955–1963 CrossRef CAS.
- Y. Rong, L. Liu, A. Mei, X. Li and H. Han, Adv. Energy Mater., 2015, 5, 1501066 CrossRef.
- Y. Liu, S. Akin, L. Pan, R. Uchida, N. Arora, J. V. Milić, A. Hinderhofer, F. Schreiber, A. R. Uhl and S. M. Zakeeruddin, Sci. Adv., 2019, 5, 2543 CrossRef.
- B. Conings, J. Drijkoningen, N. Gauquelin, A. Babayigit, J. D'Haen, L. D'Olieslaeger, A. Ethirajan, J. Verbeeck, J. Manca and E. Mosconi, Adv. Energy Mater., 2015, 5, 1500477 CrossRef.
- G. Divitini, S. Cacovich, F. Matteocci, L. Cinà, A. D. Carlo and C. Ducati, Nat. Energy, 2016, 15012 CrossRef CAS.
- H. Wang, R. Liu, Y. Li, X. Lü, Q. Wang, S. Zhao, K. Yuan, Z. Cui, X. Li and S. Xin, Joule, 2018, 2, 337–348 CrossRef CAS.
- Y. Yu, C. Wang, C. R. Grice, N. Shrestha, J. Chen, D. Zhao, W. Liao, A. J. Cimaroli, P. J. Roland and R. J. Ellingson, Chemsuschem, 2016, 9, 3288 CrossRef CAS.
- P. Gratia, G. Grancini, J.-N. Audinot, X. Jeanbourquin, E. Mosconi, I. Zimmermann, D. Dowsett, Y. Lee, M. Grätzel and F. De Angelis, J. Am. Chem. Soc., 2016, 138, 15821–15824 CrossRef CAS.
- D. P. Mcmeekin, G. Sadoughi, W. Rehman, G. E. Eperon, M. Saliba, M. T. Hörantner, A. Haghighirad, N. Sakai, L. Korte, B. Rech, M. B. Johnston, L. M. Herz and H. J. Snaith, Science, 2016, 351, 151 CrossRef CAS.
- M. Saliba, T. Matsui, J. Y. Seo, K. Domanski, J. P. Correa-Baena, M. K. Nazeeruddin, S. M. Zakeeruddin, W. Tress, A. Abate and A. Hagfeldt, Energy Environ. Sci., 2016, 9, 1989–1997 RSC.
- L. T. Schelhas, Z. Li, J. A. Christians, A. Goyal and J. J. Berry, Energy Environ. Sci., 2019, 12, 1341–1348 RSC.
- D. Kubicki, D. Prochowicz, A. Hofstetter, S. M. Zakeeruddin, M. Grätzel and L. Emsley, J. Am. Chem. Soc., 2017, 139, 7b07223 Search PubMed.
- Y. Huang, L. Zhao, Y. Li, W. Li and S. Wang, Appl. Surf. Sci., 2019, 493, 975–981 CrossRef CAS.
- D. Yang, Z. Yang, W. Qin, Y. Zhang, S. F. Liu and C. Li, J. Mater. Chem. A, 2015, 3, 9401–9405 RSC.
- E. L. Unger, E. T. Hoke, C. D. Bailie, W. H. Nguyen, A. R. Bowring, T. Heumüller, M. G. Christoforo and M. D. McGehee, Energy Environ. Sci., 2014, 7, 3690–3698 RSC.
- F. Zheng, X. Hai, Y. Wang, Z. Zhao, Z. Lin, H. C. Cheng, S. J. Lee, G. Wang, Z. Feng and W. A. G. Iii, Joule, 2017, 117300302 Search PubMed.
- J. You, M. Lei, T. B. Song, T. F. Guo, Y. Yang, W. H. Chang, Z. Hong, H. Chen, H. Zhou and C. Qi, Nat. Nanotechnol., 2015, 11, 75–81 CrossRef PubMed.
- Y. Zhang, Z. Zhou, F. Ji, Z. Li, G. Cui, P. Gao, E. Oveisi, M. K. Nazeeruddin and S. Pang, Adv. Mater., 2018, 30, 1707143 CrossRef.
- M. I. Saidaminov, A. L. Abdelhady, B. Murali, E. Alarousu, V. M. Burlakov, W. Peng, I. Dursun, L. Wang, Y. He and G. Maculan, Nat. Commun., 2015, 6, 7586 CrossRef PubMed.
- C. Wu, H. Li, Y. Yan, C. Bo, P. Jian, L. Jian, M. Sanghadasa and S. Priya, Nano Energy, 2017, 36, 295–302 CrossRef CAS.
- Q. Han, S. H. Bae, P. Sun, Y. T. Hsieh, Y. Yang, Y. S. Rim, H. Zhao, Q. Chen, W. Shi and G. Li, Adv. Mater., 2016, 28, 2253–2258 CrossRef CAS.
- Z. Li, M. Yang, J.-S. Park, S.-H. Wei, J. J. Berry and K. Zhu, Chem. Mater., 2015, 28, 284–292 CrossRef.
- C. Liu, K. Wang, C. Yi, X. Shi, A. W. Smith, X. Gong and A. J. Heeger, Adv. Funct. Mater., 2016, 26, 101–110 CrossRef CAS.
- H. Wang, H. Wu, Y. Xian, G. Niu, W. Yuan, H. Li, H. Yin, P. Liu, Y. Long and W. Li, ACS Appl. Mater. Interfaces, 2019, 11, 13812–13821 CrossRef CAS PubMed.
- M. Saliba, T. Matsui, K. Domanski, J. Y. Seo, A. Ummadisingu, S. M. Zakeeruddin, J. P. Correa-Baena, W. R. Tress, A. Abate and A. Hagfeldt, Science, 2016, 354, 206 CrossRef CAS PubMed.
- D. Prochowicz, R. Runjhun, M. M. Tavakoli, P. Yadav, M. Saski, A. Q. Alanazi, D. J. Kubicki, Z. Kaszkur, S. M. Zakeeruddin and J. Lewiński, Chem. Mater., 2019, 31, 1620–1627 CrossRef CAS.
- K. Wang, W. S. Subhani, Y. Wang, X. Zuo, H. Wang, L. Duan and S. Liu, Adv. Mater., 2019, 1902037 CrossRef PubMed.
- J. P. Correa-Baena, Y. Luo, T. M. Brenner, J. Snaider, S. Sun, X. Li, M. A. Jensen, L. Nienhaus, S. Wieghold and J. R. Poindexter, Science, 2019, 363, 627–631 CrossRef CAS PubMed.
- M. S. D. Holanda, R. Szostak, P. E. Marchezi, L. G. T. A. Duarte and A. F. Nogueira, Sol. RRL, 2019, 1900199 CrossRef.
- Y. Liu, Z. Yang, D. Cui, X. Ren, J. Sun, X. Liu, J. Zhang, Q. Wei, H. Fan and F. Yu, Adv. Mater., 2015, 27, 5176–5183 CrossRef CAS PubMed.
- O. Nazarenko, S. Yakunin, V. Morad, I. Cherniukh and M. V. Kovalenko, NPG Asia Mater., 2017, 9, 373 CrossRef.
- E. J. Juarez-Perez, Z. Hawash, S. R. Raga, L. K. Ono and Y. Qi, Energy Environ. Sci., 2016, 9, 1039 RSC.
- I. Deretzis, A. Alberti, G. Pellegrino, E. Smecca, F. Giannazzo, N. Sakai, T. Miyasaka and A. La Magna, Appl. Phys. Lett., 2015, 106, 131904 CrossRef.
- B. Conings, J. Drijkoningen, N. Gauquelin, A. Babayigit, J. D'Haen, L. D'Olieslaeger, A. Ethirajan, J. Verbeeck, J. Manca and E. Mosconi, Adv. Energy Mater., 2015, 5, 1500477 CrossRef.
Footnote |
† Electronic supplementary information (ESI) available. See DOI: 10.1039/c9na00682f |
|
This journal is © The Royal Society of Chemistry 2020 |
Click here to see how this site uses Cookies. View our privacy policy here.