DOI:
10.1039/C9NA00766K
(Paper)
Nanoscale Adv., 2020,
2, 808-813
Charge transfer between lead halide perovskite nanocrystals and single-walled carbon nanotubes†
Received
7th December 2019
, Accepted 2nd January 2020
First published on 2nd January 2020
Abstract
A charge transfer study between lead halide-based perovskite nanocrystals and single-walled carbon nanotubes (PNC@CNT nanocomposite) was performed. Solution-processed MAPbX3 PNCs displayed very bright luminescence, but it quenched in the presence of CNTs. This was attributed to the electron transfer from PNCs to CNTs. The detailed changes in fluorescence lifetime were investigated through time-correlated single-photon counting (TCSPC), which suggested mixed static and dynamic quenching along with a decrease in the lifetime. Morphological changes were investigated via transmission electron microscopy (TEM) and attributed to the incorporation of PNCs on long CNTs. Also, the PNC@CNT nanocomposite was explored for photoinduced current response, which indicated an ∼3 fold increase in photoconductivity under light illumination (with a 1 mV bias). This electron transfer study between PNCs and CNTs contributes to the exploration of charge dynamics.
1. Introduction
Metal halide perovskites1 have emerged among the superior materials for optoelectronic devices2–4 including light-emitting diodes,4 solar cells,5,6 photodetectors, lasers, and in light harvesting.2,3,8–12 The efficacy of these applications relies on good absorption characteristics, easy charge carrier formation and transport in these materials.2,13–15 Besides, the low-cost solution synthesis of these perovskite nanocrystals (PNCs) results in intense fluorescence and nearly unity quantum yield.2,16–21 On the other hand, the strong confinement of carbon nanotubes (CNTs) to one dimension has also attracted the attention of the scientific community in the areas of charge transport, field emission, gas storage, photodetectors and other photovoltaic applications.22,23 Advancement in this research field has been probed through various photo-responsive CNT-based nanocomposites with organic fluorophores and inorganic moieties including conventional quantum dots (e.g., CdSe/ZnS).24–28 The mechanistic investigation of CNTs and quantum dots revealed that the excited states of fluorescent quantum dots donate either excited electrons or holes to CNTs, which leads to the quenching of the photoluminescence.25
PNCs have size-dependent properties7,17 because of the quantum confinement effect, whereas CNTs have high electron transfer properties because of their nanoscale size, large surface area and direct path for charge transport.29–31 The formation of nanocomposites by combining the properties of both materials will lead to new avenues of opportunities in the field of nanotechnology. The photo-excited electron can be transferred from electron donor PNCs to electron acceptor CNTs. Earlier investigations on electron migration through static fluorescence quenching have been performed by Nair et al. using FAPbBr3 and fullerene C60.32 Kuang and coworkers studied the improvements in the charge transfer and light-harvesting properties of mixed cesium lead halide PNCs and CNTs.33 Chen and colleagues recently reported graphene sandwich stable PNC light-emissive ultrasensitive and ultrafast broadband phototransistors.34
Herein, we report a detailed electron transfer study using single-walled CNTs and methylammonium lead halide PNCs. The preference of single-walled CNTs over C60 fullerene is due to their superior electron transfer properties. Also, we have investigated the steady-state photoluminescence and the corresponding time-resolved lifetime, through which we have found a mixture of static and dynamic quenching (Fig. 1). The methods of preparation of MAPbBr3, MAPbI3 and CNT solutions are detailed in the ESI.†
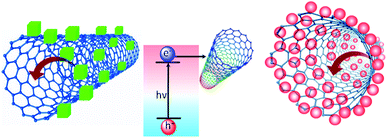 |
| Fig. 1 Schematic representation of electron transfer from MAPbX3 to a single-walled carbon nanotube. | |
The absorption spectra of blue luminescent MAPbBr3 and red luminescent MAPbI3 are shown in Fig. 2a, and images of MAPbI3 PNCs in daylight and UV light are presented in Fig. 2b and c. Images of green and blue luminescent MAPbBr3 PNCs in the presence of increasing concentrations of CNT are shown in Fig. S1.† XRD patterns of synthesized perovskite nanocrystals are shown in Fig. S2† for blue luminescent MAPbBr3 and red luminescent MAPbI3, confirming the presence of the cubic and tetragonal phases in MAPbBr3 and MAPbI3 PNC.17 Absorption peaks of MAPbBr3 solution were observed at 462 nm and at 563 and 604 nm for MAPbI3 in the presence of CNTs. MAPbX3 PNCs are highly fluorescent and their optical properties can be easily modified by size variation. The addition of CNTs to PNC solution leads to the adhering of quantum dots to the walls of CNTs because of van der Waals interactions. In our case, we sonicated the CNT solution for a longer time to separate the CNT bundles and increase the surface area of CNTs so that electron transfer would take place more efficiently. Pristine CNTs (not functionalized) were used, and the presence of oleic acid and oleylamine helped the adhesion of PNCs to the walls of the CNTs. To monitor the effect of solvent (i.e. CHCl3), we performed the emission studies in the presence of CHCl3 (without the addition of CNT) and with respect to time as shown in Fig. S3,† indicating the negligible effect of the solvent, which confirmed that the quenching is due to the adhesion of perovskite nanocrystals to CNTs.
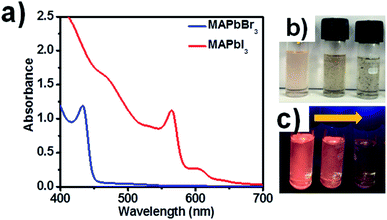 |
| Fig. 2 (a) Absorption spectra of MAPbBr3 and MAPbI3 PNCs in the presence of CNTs. (b) Daylight images of MAPbI3 solution with increasing concentration of CNTs (from left to right). (c) MAPbI3 solution with increasing concentration of CNTs (from left to right) under UV light. | |
As shown in Fig. 3a–c, there was almost 100% fluorescence quenching of blue, green and red luminescent PNCs with CNTs, which is clear evidence that the electron transfer takes place from the electron donor PNCs to the acceptor CNT bundles. The quenching experiment was performed with the sequential addition of CNT solution (0.01% w/v in chloroform) from 10 μL to 300 μL. We observed blue shifts in all PNC peaks as compared to previous reports17 due to the dilution of samples before quenching studies. In Fig. 3a–c, the emission peaks of the blue luminescent MAPbBr3, green luminescent MAPbBr3 and red luminescent MAPbI3 (without CNTs) were observed at 443 nm, 510 nm and 596 nm, respectively.
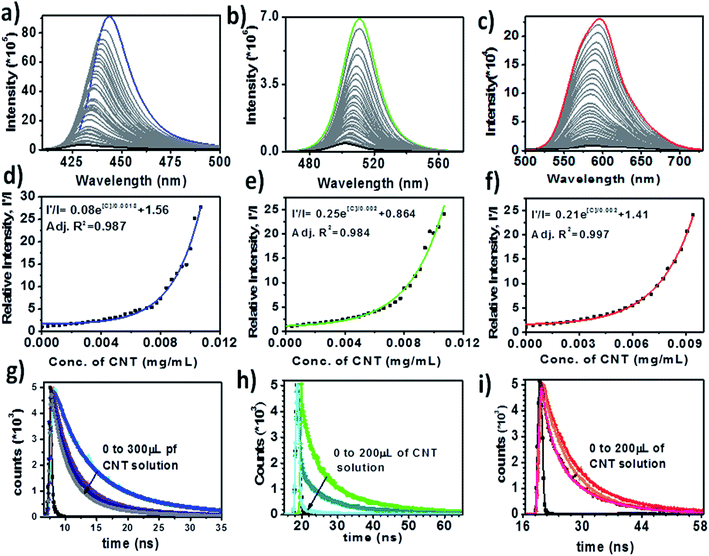 |
| Fig. 3 Photoluminescence (PL) quenching of (a) blue luminescent MAPbBr3, (b) green luminescent MAPbBr3, and (c) red luminescent MAPbI3 PNCs with CNTs. (d) Relative PL intensity changes with CNT concentration on the quenching of (d) blue, (e) green, and (f) red luminescent PNCs. TCSPC measurements of (g) blue, (h) green and (i) red luminescent PNCs on the addition of CNTs. The CNTs were added in the form of 0.01% w/v solution. | |
We observed almost 100% quenching with the addition of 300 μL of CNT solution for all three PNCs. Apart from the quenching of the emission peaks, we also observed blue shifts in the spectra (initially) on increasing the amount of CNT (predominantly for blue luminescent MAPbBr3 PNCs). The rate of PL quenching was nonlinear as shown in Fig. 3d–f. A possible reason for these PNC@CNT nanocomposites to give nonlinear Stern–Volmer plots is the presence of both static and dynamic quenching.35Fig. 3d–f shows curves along with exponential equations and rate constants for blue, green and red luminescent PNCs, respectively.
The most efficient way to determine whether quenching is static, dynamic or both is through PL decay lifetime measurements. If the lifetime values remain unchanged in the presence of CNTs, it might be due to static quenching, as previously reported with FAPbBr3 and fullerene.28 However, in the case of MAPbX3 PNCs there was a decrease in the lifetime of PNCs with increasing the amount of CNTs, which was attributed to the presence of static and dynamic quenching as shown in Fig. 3g–i. The average lifetimes along with the details of parameters for blue, red and green luminescent PNCs in the presence of CNT solution are listed in Table 1. The average lifetime was calculated by the following formula:
τavg = (A1τ12 + A2τ22 + A3τ32)/(A1τ1 + A2τ2 + A3τ3) |
where
A1,
A2 and
A3 are weighing parameters,
τ1,
τ2 and
τ3 are the corresponding lifetimes and
τavg is the average lifetime.
Table 1 TCSPC details of PNC solutions in the presence of CNT
Sample |
CNT volume (μL) |
A
1
|
A
2
|
A
3
|
τ
1 (ns) |
τ
2 (ns) |
τ
3 (ns) |
τ
avg (ns) |
Blue MAPbBr3 |
0 |
32.24 |
53.35 |
14.40 |
4.23 |
10.53 |
60.08 |
37.40 |
100 |
48.31 |
41.89 |
9.80 |
2.82 |
8.14 |
53.82 |
31.40 |
200 |
34.87 |
9.84 |
55.29 |
8.93 |
55.41 |
2.88 |
32.92 |
300 |
37.35 |
9.44 |
53.22 |
7.69 |
46.58 |
2.45 |
26.88 |
Green MAPbBr3 |
0 |
41.95 |
27.51 |
30.54 |
9.05 |
0.12 |
32.63 |
26 |
100 |
3.76 |
3.96 |
92.27 |
6.12 |
24.96 |
0.044 |
20 |
200 |
9.04 |
12.93 |
78.03 |
2.75 |
17.81 |
0.25 |
15.19 |
Red MAPbI3 |
0 |
37.60 |
54.26 |
8.14 |
5.63 |
12.03 |
52.30 |
24.26 |
100 |
47.62 |
47.56 |
4.86 |
5.41 |
10.26 |
49.35 |
18.51 |
200 |
22.10 |
67.51 |
10.39 |
2.56 |
9.03 |
31.68 |
16 |
The X-ray diffraction (XRD) pattern of MAPbBr3@CNT is provided in Fig. S4,† showing the presence of perovskite peaks. TEM images of blue, green and red luminescent MAPbX3 PNCs are given in Fig. S5.† From Fig. S5,† the tunable morphology is directly evidenced by the TEM images, where the morphology changes from quantum dots to nanoplates to quantum dots by shifting from blue-green-red luminescent MAPbX3 PNCs, which leads to adhesion to CNTs as shown in Fig. 4. These interactions are due to the electron migration or transfer from electron donor halide perovskites to electron acceptor CNTs. TEM images of PNC@CNT nanocomposites provide direct evidence of PNCs adhering to CNTs as shown in Fig. 4. The typical Fourier transform-infrared spectroscopy (FT-IR) vibration modes are presented in Fig. S6.† XPS analysis of green luminescent MAPbBr3 in the presence of CNT confirms the presence of the elemental composition of PNC with the main peaks of Pb 4f, C 1s, N 1s and Br 3d in narrow scans as shown in Fig. S7.†
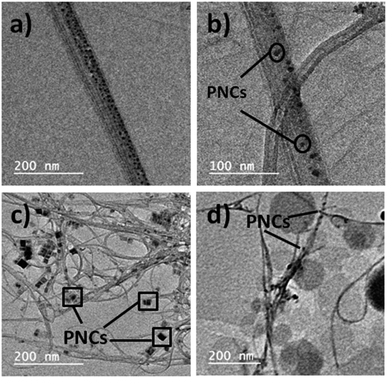 |
| Fig. 4 TEM images of (a), (b) blue, (c) green and (d) red luminescent PNCs in the presence of CNTs. | |
2. Photoresponse study
A prototype device was fabricated to investigate the conductive properties of the PNC@CNT nanocomposite. A schematic illustration is provided in Fig. 5 for measuring the photoconductivity. The device was made by two silver electrodes separated by 0.5 mm on a silica substrate and drop-casting the concentrated MAPbI3@CNT nanocomposite sample to connect the two electrodes. The photoinduced current was measured on a Keithley 2612B source meter while irradiating the sample with a daylight lamp having a brightness of 1200 lumens. Fig. 5a shows the current–voltage curve of the nanocomposite in the dark and in the presence of light, displaying excellent ohmic responses. The resistance in the presence of light was 14.2 Ω, whereas in the dark it was 15.37 Ω, which confirms that irradiation in light leads to an increase in current (Fig. 5a). Photoinduced charge was generated in this device system upon irradiation with light.21 It was found that the photoconductivity increased ∼3 fold under light illumination at 1 mV voltage (Fig. 5b) and the photocurrent was basically constant over time as shown in Fig. 5b, indicating the good stability of the nanocomposite over a number of cycles. It has been confirmed that the presence of CNT alone does not lead to the change in current under light irradiation.36,37 As such, the increase in the current (Fig. 5a and b) was due to the presence of PNCs and that led to the charge transfer between CNTs and PNCs and completed the circuit. For multiple current cycle investigations, the light was turned on and off with a 15 s interval.
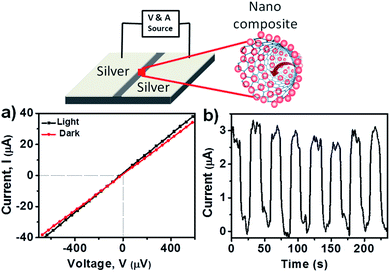 |
| Fig. 5 Schematic illustration of the fabricated device (top); (a) the I–V curve for MAPbI3@CNT in the presence and absence of light and (b) the corresponding I–t curve for MAPbI3@CNT. | |
Photocurrent (Iph) was evaluated by Iph = |Il − Id| where Id is the current in the dark and Il is the current under illumination. As shown in Fig. 5b, the PNC@CNT nanocomposite exhibited a photocurrent which led to a difference in current under light irradiation. The device exhibited a photocurrent of 2.92 μA because of the adhesion of PNCs on CNTs through van der Waals interactions. Applied bias led to the generation of charge carriers that migrated from the electron donor PNCs to electron acceptor CNTs, leading to the enhancement of the current. These results indicate that charge transfer between PNCs and CNTs produced a noticeable photocurrent, which paves the way towards new optoelectronic and nanosensor-based applications.
3. Conclusions
In summary, we studied the charge transfer behaviour between lead halide based perovskites and carbon nanotubes. Fluorescence quenching and lifetime studies revealed the presence of static as well as dynamic quenching. TEM morphological studies and PL quenching also indicated significant interaction enhancement between PNCs and CNTs. Photoconductive perovskite nanocomposites have opened new avenues for solar cells, sensor-based nanodevices and light-driven optoelectronics.
Conflicts of interest
There are no conflicts of interest to declare.
Acknowledgements
This work was supported by Indian Institute of Technology Roorkee and Louisiana State University in Shreveport, LA, USA. P. K. acknowledges the Council of Scientific & Industrial Research (CSIR (01(2990)/19/EMR-II)), New Delhi, India. Authors acknowledge Institute Instrumentation Centre (IIC), IIT Roorkee for instrument facility. P. B. acknowledges J. William Fulbright Foreign Scholarships Board (FFSB), United States-India Educational Foundation (USIEF) and Institute of International Education (IIE) for Fulbright-Nehru Doctoral Program.
References
- L. C. Schmidt, A. Pertegás, S. González-Carrero, O. Malinkiewicz, S. Agouram, G. Mínguez Espallargas, H. J. Bolink, R. E. Galian and J. Pérez-Prieto, Non Template Synthesis of MAPbBr3 Perovskite Nanoparticles, J. Am. Chem. Soc., 2014, 136, 850–853 CrossRef CAS PubMed.
- H. Huang, L. Polavarapu, J. A. Sichert, A. S. Susha, A. S. Urban and A. L. Rogach, Colloidal Lead Halide Perovskite Nanocrystals: Synthesis, Optical Properties and Applications, NPG Asia Mater., 2016, 8, e328 CrossRef CAS.
- Y. Zhao and K. Zhu, Organic and Inorganic Hybrid Lead Halide Perovskites for Optoelectronic and Electronic Applications, Chem. Soc. Rev., 2016, 45, 655 RSC.
- M. Lu, J. Guo, P. Lu, L. Zhang, Y. Zhang, Q. Dai, Y. Hu, V. L. Colvin and W. W. Yu, Ammonium-Thiocyanate-Passivated CsPbI3 Perovskite Nanocrystals for Efficient Red Light-Emitting Diodes, J. Phys. Chem. C, 2019, 123, 22787–22792 CrossRef CAS.
- A. Kojima, K. Teshima, Y. Shirai and T. Miyasaka, Organometal Halide Perovskites as Visible-Light Sensitizers for Photovoltaic Cells, J. Am. Chem. Soc., 2009, 131, 6050–6051 CrossRef CAS PubMed.
-
(a) M. M. Lee, J. Teuscher, T. Miyasaka, T. N. Murakami and H. J. Snaith, Efficient Hybrid Solar Cells Based on Meso-superstructured Organometal Halide Perovskites, Science, 2012, 338, 643–647 CrossRef CAS PubMed;
(b) M. F. Aygüler, M. D. Weber, B. M. D. Puscher, D. D. Medina, P. Docampo and R. D. Costa, Light Emitting Electrochemical Cells Based on Hybrid Lead Halide Perovskite Nanoparticles, J. Phys. Chem. C, 2015, 119, 12047–12054 CrossRef.
- L. Protesescu, S. Yakunin, M. I. Bodnarchuk, F. Krieg, R. Caputo, C. H. Hendon, R. X. Yang, A. Walsh and M. V. Kovalenko, Nanocrystals of Cesium Lead Halide Perovskites (CsPbX3, X = Cl, Br and I): Novel Optoelectronic Materials Showing Bright Emission with Wide Color Gamut, Nano Lett., 2015, 15, 3692–3696 CrossRef CAS PubMed.
-
(a) C. Muthu and V. C. Nair, CH3NH3PbBr3 Perovskite Nanocrystals as Efficient Light-Harvesting Antenna for Fluorescence Resonance Energy Transfer, Chem. - Asian J., 2017, 12, 988–995 CrossRef CAS;
(b) P. Bansal and P. Kar, Probing the Energy Transfer Process by Controlling the Morphology of CH3NH3PbBr3 Nanocrystals with Rhodamine B Dye, J. Lumin., 2019, 215, 116609 CrossRef CAS.
- J. Zhang, C. Wang, X. Shen, M. Lu, J. Guo, X. Bai, Y. Zhang and W. W. Yu, Perovskite Light Emitting Diodes for Uniform Eight-Segment Displays, Appl. Phys. Lett., 2019, 115, 193104 CrossRef.
- P. Lu, M. Lu, H. Wang, N. Sui, Z. Shi, W. W. Yu and Y. Zhang, Metal Halide Perovskite Nanocrystals and Their Applications on Optoelectronic Devices, InfoMat, 2019, 1, 1–29 CrossRef.
- M. Lu, H. Wu, X. Zhang, H. Wang, Y. Hu, V. L. Colvin, Y. Zhang and W. W. Yu, Highly Flexible CsPbI3 Perovskite Nanocrystal Light-Emitting Diodes, ChemNanoMat, 2019, 5, 313–317 CrossRef CAS.
- H. Wu, Y. Zhang, M. Lu, X. Zhang, C. Sun, T. Zhang, V. L. Colvin and W. W. Yu, Surface Ligand Modification of Cesium Lead Bromide Nanocrystals for Improved Light-Emitting Performance, Nanoscale, 2018, 10, 4173–4178 RSC.
- G. Xing, N. Mathews, S. Sun, S. S. Lim, Y. M. Lam, M. Grätzel, S. Mhaisalkar and T. C. Sum, Long-Range
Balanced Electron and Hole-transport Lengths in Organic-Inorganic CH3NH3PbI3, Science, 2013, 342, 344–347 CrossRef CAS PubMed.
- A. M. Ontoria, I. Zimmermann, I. Garcia-Benito, P. Gratia, C. Roldän-Carmona, S. Aghazada, M. Grätzel, M. K. Nazeerudin and N. Martin, Benzotrithiophene-Based Hole-Transporting Materials for 18.2% Perovskite Solar Cells, Angew. Chem., Int. Ed., 2016, 55, 6270–6274 CrossRef PubMed.
-
(a) S. D. Stranks, G. E. Eperon, G. Grancini, C. Menelaou, M. J. P. Alcocer, T. Leijtens, L. M. Herz, A. Petrozza and H. J. Snaith, Electron-Hole Diffusion Lengths Exceeding 1 Micrometer in an Organometal Trihalide Perovskite Absorber, Science, 2013, 342, 341–343 CrossRef CAS PubMed;
(b) S. D. Stranks and H. J. Snaith, Metal Halide Perovskites for Photovoltaic and Light-emitting Devices, Nat. Nanotechnol., 2015, 10, 391–402 CrossRef CAS.
- H. C. Wang, S. Y. Lin, A. C. Tang, B. P. Singh, H. C. Tong, C. Y. Chen, Y. C. Lee, T. L. Tsai and R. S. Liu, Mesoporous Silica Particles Integrated with All-Inorganic CsPbBr3 Perovskite Quantum-Dot Nanocomposites (MP-PQDs) with High Stability and Wide Color Gamut used for Backlight Display, Angew. Chem., Int. Ed., 2016, 55, 7924–7929 CrossRef CAS PubMed.
- P. Bansal, Y. Khan and P. Kar, High Luminescence Color Gradient by Physical Mixing of two Perovskite Nanocrystals, New J. Chem., 2019, 43, 4116–4122 RSC.
- P. Bansal, Y. Khan, G. K. Nim and P. Kar, Surface Modulation of Solution Processed Organolead Halide Perovskite Quantum dots to Large Nanocrystals Integrated with Silica Gel G, Chem. Commun., 2018, 54, 3508–3511 RSC.
- P. Bansal and P. Kar, Ultralong Micro-Belts of Luminescent Lead Halide based Perovskites, Chem. Commun., 2019, 55, 6543–6546 RSC.
- D. Zhang, S. W. Eaton, Y. Yu, L. Dou and P. Yang, Solution-Phase Synthesis of Cesium Lead Halide Perovskite Nanowires, J. Am. Chem. Soc., 2015, 137, 9230–9233 CrossRef CAS PubMed.
- H. Lin, C. Zhou, M. Chaaban, L.-J. Xu, Y. Zhou, J. Neu, M. Worku, E. Berkwits, Q. He, S. Lee, X. Lin, T. Siegrist, M.-H. Du and B. Ma, Bulk Assembly of Zero-Dimensional Organic Lead Bromide Hybrid with Efficient Blue Emission, ACS Mater. Lett., 2019, 1, 594–598 CrossRef CAS.
- A. Javey, J. Guo, Q. Wang, M. Lundstorm and H. J. Dai, Ballistic Carbon Nanotube Field-Effect Transistors, Nature, 2003, 424, 654–657 CrossRef CAS PubMed.
- D. Shahrjerdi, A. D. Franklin, S. Oida, J. A. Ott, G. S. Tulevski and W. Haensch, High Performance Air-Stable n-Type Carbon Nanotube Transistors with Erbium Contacts, ACS Nano, 2013, 7, 8303–8308 CrossRef CAS PubMed.
- L. Wang, J. Han, Y. Zhu, R. Zhou, C. Jaye, H. Liu, Z.-Q. Li, G. T. Taylor, D. A. Fischer, J. Appenzeller and S. S. Wong, Probing the Dependence of Electron Transfer on Size and Coverage in Carbon Nanotube-Quantum Dot Heterostructures, J. Phys. Chem. C, 2015, 119, 26327–26338 CrossRef CAS.
- J. E. Weaver, M. R. Dasari, A. Datar, S. Talapatra and P. Kohli, Investigating Photoinduced Charge Transfer in Carbon Nanotube-Perylene-Quantum Dot Hybrid Nanocomposites, ACS Nano, 2010, 4, 6883–6893 CrossRef CAS PubMed.
- W. Feng, C. Qin, Y. Shen, Y. Li, W. Luo, A. Haoran and Y. Feng, A Layer-Nanostructured Assembly of PbS Quantum Dot/Multiwalled Carbon Nanotube for a High Performance Photoswitch, Sci. Rep., 2013, 4, 3777 CrossRef PubMed.
- M. Olek, T. Büsgen, M. Hilgendorff and M. Giersig, Quantum Dot Modified Multiwall Carbon Nanotubes, J. Phys. Chem. B, 2006, 110, 12901–12904 CrossRef CAS PubMed.
- A. D. Franklin and Z. Chen, Length Scaling of Carbon Nanotube Transistors, Nat. Nanotechnol., 2010, 5, 858–862 CrossRef CAS.
- G. Yu, J. C. Hummelen, F. Wudl and A. J. Heeger, Polymer Photovoltaic Cells-Enhanced Efficiencies via a Network of Internal Donor-Acceptor Heterojunctions, Science, 1995, 270, 1789–1791 CrossRef CAS.
- S. Iijima, Helical Microtubules of Graphitic Carbon, Nature, 1991, 354, 56–58 CrossRef CAS.
- J. A. Misewich, R. Martel, P. Avouris, J. C. Tsang, S. Heinz and J. Tersoff, Electrically Induced Optical Emission from a Carbon Nanotube FET, Science, 2003, 300, 783 CrossRef CAS PubMed.
- V. C. Nair, C. Muthu, A. L. Rogach, R. Kohara and V. Biju, Channeling Exciton Migration into Electron Transfer in Formamidinium Lead Bromide Perovskite Nanocrystal/Fullerene Composites, Angew. Chem., Int. Ed., 2017, 56, 1214–1218 CrossRef CAS.
- M.-Z. Yang, Y.-F. Xu, J.-F. Liao, X.-D. Wang, H.-Y. Chen and D.-B. Kuang, Constructing CsPbBrxI3-x Nanocrystals/Carbon Nanotube Composites with Improved Charge Transfer and Light Harvesting for Enhanced Photoelectrochemical Activity, J. Mater. Chem. A, 2019, 7, 5409–5415 RSC.
- K. P. Bera, G. Haider, Y.-T. Huang, P. K. Roy, C. R. P. Inbaraj, Y.-M. Liao, H.-I. Lin, C.-H. Lu, C. Shen, W. Y. Shih, W.-H. Shih and Y.-F. Chen, Graphene Sandwich Stable Perovskite Quantum dot Light Emissive Ultrasensitive and Ultrafast Broadband Vertical Phototransistors, ACS Nano, 2019, 13, 12540–12552 CrossRef CAS.
- J. Liu, Y. Zhong, P. Lu, Y. Hong, J. W. Y. Lam, M. Faisal, Y. Yu, K. S. Wong and B. Z. Tang, A Superamplification Effect in the Detection of Explosives by a Fluorescent Hyberbranched Poly(silylenephenylene) with Aggregation-Enhanced Emission Characteristics, Polym. Chem., 2010, 1, 426–429 RSC.
- X. L. Li, Y. Jia and A. Y. Gao, Tailored Single-Walled Carbon Nanotube CdS Nanoparticle Hybrids for Tunable Optoelectronic Devices, ACS Nano, 2009, 4, 506–512 CrossRef PubMed.
- R. Robel, B. A. Bunker and P. V. Kamat, Single-Walled Carbon Nanotube CdS Nanocomposites as Light Harvesting Assemblies Photoinduced Charge Transfer Interactions, Adv. Mater., 2005, 17, 2458–2463 CrossRef.
Footnote |
† Electronic supplementary information (ESI) available. See DOI: 10.1039/c9na00766k |
|
This journal is © The Royal Society of Chemistry 2020 |
Click here to see how this site uses Cookies. View our privacy policy here.