DOI:
10.1039/D0NA00357C
(Paper)
Nanoscale Adv., 2020,
2, 3972-3982
Biogenic synthesis of silver nanoparticles using cyanobacterium Leptolyngbya sp. WUC 59 cell-free extract and their effects on bacterial growth and seed germination
Received
4th May 2020
, Accepted 29th July 2020
First published on 30th July 2020
Abstract
The biogenic synthesis of metal nanoparticles (NPs) is of great significance, as it renders clean, biocompatible, innocuous and worthwhile production. Here, we present a clean and sustainable route for the synthesis of silver nanoparticles (Ag NPs) using the cell-free aqueous extract of the cyanobacterium Leptolyngbya sp. WUC 59, isolated from polluted water and identified using a polyphasic approach. The conformation and characterisation of the as-synthesized biogenic Ag NPs was carried out using various sophisticated techniques like UV-visible (UV-Vis), X-ray diffraction (XRD), Fourier transform infrared (FTIR), energy dispersive X-ray spectroscopy (EDS) and high-resolution transmission electron microscopy (HRTEM). The sharp colour change and emergence of a characteristic peak at 430 nm in the UV-Vis spectrum confirm the formation of the Ag NPs. The morphological and physical appearance indicated that the synthesized Ag NPs are crystalline with a typical size of 20–35 nm. Furthermore, the bio-reduced nanoparticles were explored for their antibacterial activity against Bacillus subtilis and Escherichia coli bacteria, seed germination effects and early seedling growth of wheat (Triticum aestivum L.). The Ag NPs significantly suppressed the growth of both Bacillus subtilis and Escherichia coli bacteria with the treatment of 10 mg L−1 concentration within the initial 3 hours. The lower concentration (25 mg L−1) of the synthesized Ag NPs significantly enhanced the seed germination and early seedling growth of wheat in comparison to the control on the 4th and 8th day. The present investigations show that the use of the cyanobacterium Leptolyngbya sp. WUC 59 provides a simple, cost-effective and eco-friendly tool for the synthesis of Ag NPs. Moreover, it could have great potential for use as an alternative to chemical-based bactericides not only in pharmaceutical industries, but also to control bacterial diseases in agricultural crops.
Introduction
Nanotechnology is an emerging area with increased focus in various fields of science, such as material science, medical science and atomic science. It is the science of the synthesis of nanomaterials, and the investigation of their applications with their nanometre dimensions.1,2 Recently, the synthesis of nanomaterials has gained massive consideration across the world because of their intensive applications, ranging from target drug delivery, cancer treatment, biomedicine and environmental remediation to antimicrobial progress.3–5 The most significant perspectives that characterize its appropriateness for these applications are the synthesis procedures. Numerous physical and chemical strategies for synthesizing nanomaterials, such as the reduction of silver salt solution, the thermal decomposition of silver compound and sonication have been discussed in the literature.6,7 However, these methods have terrible impacts on the environment as well as on human health as these strategies discharge huge amount of hazardous chemicals and toxic byproducts.8–11 Consequently, there is an emerging concern to develop green, proficient, innocuous, non-destructive, ecological and biological approaches for the synthesis of nanoparticles (NPs), which will be environmentally friendly.12 In particular, among the various NPs, silver nanoparticles (Ag NPs) are the most commercialised nanomaterials, and the production of these nanoparticles is supposed to escalate in the next few years.13 In the literature, bacteria, fungi, algae and plant extracts are the basic sources for the synthesis of Ag NPs.14,15 The foremost utility of employing these biological microorganisms to synthesize Ag NPs is the presence of bioactive compounds in them, as they afford a platform for different types of nanomaterials.16
Among the various microorganisms, algae are a potential source of metabolites that are essential for the reduction and fabrication of metallic nanoparticles.17 Cyanobacteria, also known as blue-green algae, are photosynthetic prokaryotes. They occupy diverse ecological habitats with widely fluctuating environmental conditions.18 They are good resources of novel secondary metabolites with their diverse applications in the field of agriculture, as nutrient supplements, bioremediation in wastewater treatment, and in the biomedical field as an antimicrobial and anticancer agent.19,20 Cyanobacteria are found to be an excellent biosystem for the intracellular or extracellular production of nanoparticles. There has been a number of reports available in the literature in which cyanobacteria have been employed for the green synthesis of nanoparticles.21 Cyanobacteria produced several biocompounds, such as pigments, proteins, and polysaccharides, which may act as reducing and capping agents during the biofabrication of nanoparticles.22 Recent reports on the synthesis of silver nanoparticles using the cyanobacterial species Oscillatoria limnetica and Desertifilum sp. have been proved to be an eco-friendly and low-priced route of synthesis with their assorted applicability.23,24
The emergence of multi drug-resistant bacteria, with the overuse of antibiotics against infectious diseases, required the development of alternative neutral antibacterial molecules having unique properties to control these types of microorganisms.25 The cyanobacteria-mediated synthesized Ag NPs have significant inhibitory activity toward Gram-negative and Gram-positive bacteria due to their small sizes and large surface-area-to-volume ratios, which eased the broad interactions between Ag NPs and bacteria.26 Current studies suggest that the biosynthesized Ag NPs have effective antimicrobial potential, and could serve as an alternative to developing antimicrobial compounds to solve the problem of drug resistance.21,23 Recently, with the improvement in the methods of NP synthesis, the use of nanotechnology has been astonishingly increased. In order to increase the capability of plants to absorb more water and nutrients from soil, there are many advanced tools in nanotechnology with intensified capacity.27 Razzaq et al.28 suggested that seedlings supplemented with silver nanoparticles increased the shoot/root length, chlorophyll and total protein content. This might be due to the uptake of silver nanoparticles through seeds, and the alteration of membranes and other cell structures, as well as protective mechanisms. It has been reported by many research groups that the effects of nanoparticles on plants depend on their size and reactivity.28,29 In this context, it is helpful to understand the role of Ag NPs during the germination of seeds and the early development of wheat seedlings (Triticum aestivum L).
In light of the benefits of green synthesis over different techniques, herein we have explored the potential of the cell extract from the cyanobacterium Leptolyngbya sp. WUC 59 as the reducing and stabilizing agent for the synthesis of Ag NPs. The effect of synthesized NPs on seed germination and the early development of wheat seedlings, as well as their antibacterial activity against Gram-negative and Gram-positive bacterial strains, has also been explored. The results obtained suggest that the biological precursors are a strong possibility for the synthesis of nanomaterials. These nanomaterials show potential for extensive applications in the future in the field of environment and energy, in addition to medical applications.
Materials and methods
Materials
In this study, analytical grade (Hi-media) chemicals were used for the preparation of culture media and to perform other experiments.
Isolation of cyanobacteria and culture conditions
Leptolyngbya sp. WUC 59 was isolated from water samples collected from a polluted stream (30° 39′ 00.7′′ N; 76° 23′ 47.9′′ E) from the district Fatehgarh Sahib, Punjab (India). Isolation and purification of the organism was performed as per our previous work18 on modified Chu-10 medium.30 The cultured strains were examined periodically using light microscopes to ensure the purity of the cultured biomass. The purified colonies were transferred to sterile 500 mL flasks containing Chu-10 medium (pH 7.8). The cultures were grown under a fluorescent lamp at a light intensity of 2000 ± 200 lux for 12 h light/dark cycle at 26 ± 2 °C for 15 days in BOD incubators (NSW, New Delhi).
Identification of Leptolyngbya sp. WUC 59
The cyanobacterium was identified on the basis of its morphological character,31 combined with partial 16S rRNA gene sequencing. Genomic DNA extraction was done by the HiPurA™ Plant Genomic DNA Miniprep Purification Spin Kit (HiMedia, Mumbai, India). A cyanobacterial 16S rRNA gene fragment of 1350 nucleotides was amplified using 5 pmol of the universal primers 27F (5′-AGAGTTTGATCCTGGCTCAG-3′) and 1492R (5′-TACGGTTACCTTGTTACGACT-3′), corresponding to the E. coli 16S rRNA gene. The total PCR reaction mixture was 50.0 μL, comprising 200 μmol L−1 dNTPs, 50 μmol L−1 each for the primer, 1× PCR buffer, 3 U Taq polymerase, and 100 ng genomic DNA. The thermo-cycling conditions involved an initial denaturation at 94 °C for 4 min, followed by 35 cycles of 1 min at 94 °C, 1 min at 52 °C, and 2 min at 72 °C, and a final extension at 72 °C for 8 min. The gel-purified product was obtained using the Gene JET Gel Extraction Kit (Fermentas, Lithuania). The sequencing was done using the Big Dye® Terminator v3.1 Cycle Sequencing Kit and an ABI Prism 310 Genetic Analyzer (Applied Biosystems, USA). The sequences were analyzed using the gapped BLASTn search algorithm, and aligned to the near neighbours. The evolutionary history of the organism was inferred using the Neighbour-Joining method,32 and the evolutionary distances were computed using the Kimura 2-parameter method.33 The phylogenetic tree was constructed using MEGA X.34
Preparation of cell-free extracts
The biomass of a 15 day-old culture of Leptolyngbya sp. WUC 59 was harvested by centrifugation at 5000 rpm for 15 min, and the pellets obtained were washed with distilled water at least twice to remove the nutrients from the culture medium. The pellets were air-dried, and the dried pellets were pulverized using pestle and mortar. An aqueous cell-free extract was prepared by mixing 50 mg of fine powder with 25 mL of double distilled (DD) water, and then incubating the mixture at 50 °C. After 24 h, the aqueous extract was again centrifuged at 10
000 rpm for 15 min, and the obtained supernatant was stored in a refrigerator at 4 °C for further use.
Biosynthesis of silver nanoparticles
The synthesis of silver nanoparticles was performed by adding 1 mL of cell extract (dropwise) into the 100 mL of 1 mM AgNO3 solution in a 250 mL conical flask. The reaction mixture was kept at 70 °C for 15 min on constant mechanical stirring. The complete reduction of Ag ions into Ag NPs was observed within 10 min, which indicated the rapid synthesis of Ag NPs. The synthesis of the nanoparticles was monitored using a UV-Vis spectrophotometer. The reaction mixture was centrifuged at 15
000 rpm for 30 min at 4 °C, and the supernatant was discarded to collect the pellet. The collected pellet was washed with DD water several times to remove impurities, and with 90% ethanol to get the pure Ag NPs powder.
Characterization of Ag NPs
Fourier transform infrared (FTIR) spectra were recorded on a PerkinElmer RX1 FTIR spectrometer. X-ray diffraction (XRD) patterns were obtained using a PAN analytical system DY 3190 X-diffractometer. Transmission electron microscopic (TEM) studies were performed using a model MIC JEM 2100. UV-Vis spectra were recorded in a quartz cuvette using a Shimadzu-UV 2600 spectrophotometer. The feasible elemental chemical composition was analysed with energy dispersive X-ray (EDX) spectroscopy (Oxford instruments: X-Max 51 – XMX0004). The average crystallite size and polydispersity index (PDI) of the Ag NPs were estimated using a Zetasizer Nano (Malvern-ZEN-1690) apparatus.
Antibacterial activity of Ag NPs
The antibacterial properties of the as-synthesized biogenic Ag NPs were examined by carrying out a set of investigations to evaluate the growth of both Gram-positive (Bacillus subtilis) and Gram-negative (Escherichia coli) bacterial strains. The strain of both kinds of microorganisms were cultured in 5 mL LB medium (Hi Media) aseptically under laminar air-flow, followed by its sub-culturing into 50 mL LB of four sets of Ag NPs having concentrations of 2 mg L−1, 5 mg L−1, 8 mg L−1, and 10 mg L−1. At the same time, a control experiment having no Ag NPs was likewise done. The optical densities of the pathogens were recorded and plotted as a function of time. The bacterial expansion was observed (after interims of 2 h) by recording the OD600 nm in the UV-Vis spectrophotometer for the control and samples. The last OD600 nm was recorded after 24 h of growth.
Effect of synthesised Ag NPs on seed germination and early seedling growth
Seeds of Triticum aestivum L. (Wheat cv PBW 660) were sterilized by washing with ethanol for about 5 min, followed by thoroughly washing with dd H2O. For this experiment, the desired concentrations were prepared by mixing the powdered Ag NPs in DD water. The seeds were placed on four layers of Whatman filter paper fitted in Petri-dishes, and were soaked with 25, 50, 75, 100 and 150 mg L−1 aqueous solutions of synthesised Ag NPs. A completely randomized design with three replications was employed for the germination test with 25 seeds in each plate. After 4 and 8 days, the seed germination rate and the seedling growth (in terms of the root and shoot length), the fresh weight and dry weight were measured. As a control, 5 mL of DD water was used without the addition of Ag NPs.
Statistical analysis
All data presented in the figures are expressed as a mean of three independent replicates (mean ± SEM). The data were analyzed by applying ANOVA and Tukey's post hoc test at 95% significance level using GraphPad Prism 8.3 software (GraphPad Software, Inc., La Jolla, CA, USA).
Results and discussion
The organism used in the present study for the biogenic synthesis of silver nanoparticles (Ag NPs) was isolated and purified from polluted water samples, and was identified using the polyphasic approach. The purified axenic culture of the isolated cyanobacterial isolate was observed under a light microscope for its phenotypic characterization. The isolate was unbranched, filamentous, and non-heterocystous. The filaments were long and arranged in parallel with a thin firm sheath. The trichome was pale to a bright blue-green in colour, and it was straight or bent with nearly round ended cells. The cell had dimensions of 1.82 ± 1 μm width and 1.43 ± 1 μm length. It was constricted at the cross wall with a rounded apical cell. The cyanobacterium belongs to the family Leptolyngbyaceae, order Synechococcales of Cyanophyceae, and is identified as Leptolyngbya sp. on the basis of its morphological characters (inset, Fig. 1). For further identification of the test organism, the partial 16S rRNA gene sequence (1350 bp) was determined and the BLASTn results revealed 99% similarity of the obtained sequence with Leptolyngbya sp. PCC 73110 NIES-3276 (LC485949). The phylogenetic tree of the 16S rRNA gene sequences aligned with the sequences of the cyanobacterial strains obtained from the NCBI GenBank showed grouping of Leptolyngbya sp. WUC 59 into the supported sequence cluster (Fig. 1). The nucleotide sequence obtained was deposited in the NCBI GenBank database under the accession number MT231937.
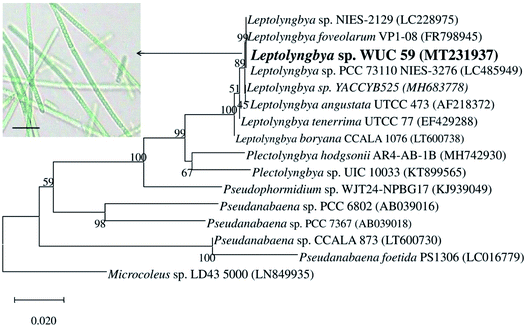 |
| Fig. 1 Phylogenetic tree showing the relationship of Leptolyngbya sp. WUC 59 (inset: microphotograph) with closely related taxa based on partial (1350 bp) 16S rRNA gene sequence. | |
After mixing the Leptolyngbya sp. WUC 59 cell-free extracts with the AgNO3 solution, the colourless solution of AgNO3 changed to a pale yellow colour, and then to a reddish brown colour within the initial 15 minutes of the reaction. The aforesaid change in colour might be the reason for the plasma resonance in Ag NPs.35 UV-visible spectroscopy was used to determine the bioreduction of Ag NPs from the Ag+ ions. Fig. 2a displays λmax at 430 nm in the absorption spectrum of the biogenic synthesized nanoparticles, which was due to plasmon resonance of Ag NPs. The UV-Vis spectra of the extract showed a peak at 325 nm, which may be due to the presence of mycosporine-like amino acids (MAAs) in the cell extract. MAA is a water-soluble compound present in cyanobacteria and absorbs specific UV-Vis radiation in the range of 280–320 nm.36 The potential of various cyanobacterial species to synthesize Ag NPs by crude extracts has been reported by many workers.21,23,24,26,37 However, the mechanism of the biogenic synthesis of Ag NPs using crude extracts of cyanobacteria is still not very clear. Tentatively, the role of the reducing agents/biomolecules present in the cell extracts of cyanobacteria, such as sugars, proteins, peptides, polysaccharides, pigments, and others has been implicated in the reduction of Ag+ ions.21,37 Ali et al.38 proposed that nitrate reductase enzyme played a role in the reduction of silver ions by cyanobacteria growing in the presence of nitrogen. The enzyme nitrate reductase converts nitrate (NO3−) to nitrite (NO2−). During this reduction process in which nitrate was converted into nitrite, the electron could be transferred to the silver ion (Ag+), which then finally reduced to metallic silver (Ag0).37 The organism used in the present study was raised in a culture medium supplemented with nitrate. Consequently, the nitrate reductase enzyme could be involved in the reduction of silver ions most likely during the process of synthesizing Ag NPs by the crude cell extract of this organism.
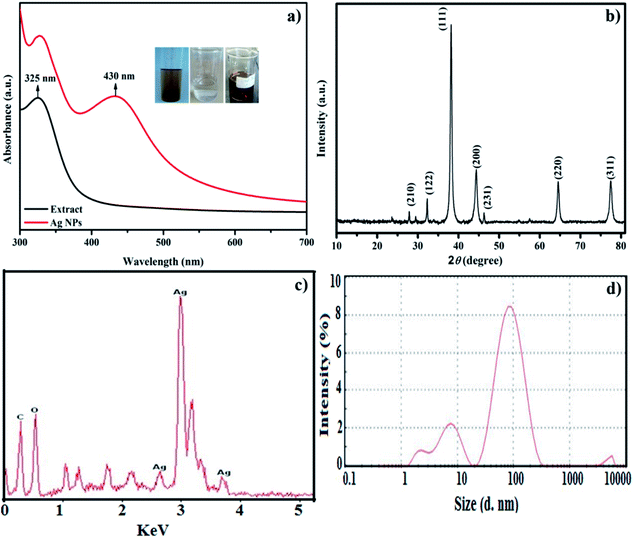 |
| Fig. 2 (a) UV-Vis spectra of extract (black line), Ag NPs (red line); (b) XRD pattern of Ag NPs; (c) EDX spectrum of Ag NPs, and (d) DLS size distribution image of the Ag NPs. | |
The stability of Ag NPs is a significant variable, particularly when they are utilized in biomedical applications. So as to consider the stability, the NPs-containing solution was kept in the dark at ambient conditions. The UV-Vis spectra of NPs were recorded between 300 nm and 700 nm at various time stretches from start to 30 days. Nevertheless, the absorption band broadened and the intensity was reduced with no change in the absorption band position, even after 30 days. The reduction and broadening in intensity might be due to the agglomeration of NPs. These results stipulate the higher stability of the Ag NPs prepared using the cyanobacterium Leptolyngbya sp. WUC 59. Furthermore, reusability studies were also achieved by performing the synthesis in triplicate in a period of 60 days. Still, there was no appreciable alteration in the optical spectra, showing that the as-synthesised Ag NPs were stable and well-dispersed.
The powdered XRD pattern of the synthesized Ag NPs was recorded between 10° to 80° at 2θ, and is demonstrated in Fig. 2b. The diffraction pattern attained for the synthesized nanoparticles was in good agreement with JCPDS card number 04-0783 with a face-centred cubic structure of Ag NPs. The reflection peaks showed Ag NPs at 2θ = 27.9 (3.19 Å), 32.3 (2.76 Å), 38.2 (2.34 Å), 44.4 (2.03 Å), 46.3 (1.95 Å), 64.7 (1.43 Å) and 77.6 (1.23 Å) with indices (210), (122), (111), (200), (231), (220) and (311), respectively. The Debye–Scherer equation was used to calculate the average crystallite size of the Ag NPs.39
where
D = average crystallite size (nm),
k = 0.9 (Scherer constant),
λ = 0.15406 (wavelength of X-rays),
β = FWHM (radians),
θ = angle of the X-ray diffraction peak. The crystallite size of the biogenic synthesized Ag particles was found to be 32 nm. The EDX spectra showed a strong Ag signal at 3 keV, along with other smaller peaks (
Fig. 2c). The presence of other smaller peaks in the EDX spectra of the synthesized Ag NPs may be due to the presence of biomolecules or chemical constituents of the extract that are often bound to the surface of the Ag NPs. The size distribution of the biogenic Ag NPs was determined with DLS (
Fig. 2d). The polydispersity index (PDI) of the silver nanoparticle suspension is 0.652, indicating that the as-prepared particles are of uniform size. The distribution profile indicates that the average size of the Ag NPs was found to be 35.84 nm, which is in good agreement with the particle size obtained from XRD.
The FTIR measurement was carried out to determine the interaction of the as-synthesized Ag NPs and biomolecules present in the extract. The absorption bands at 3851, 3741 and 3613 cm−1 in the FTIR spectrum (Fig. 3) may be due to the hydroxyl (OH) stretching vibration of the alcohol and phenols in the nanomaterial. The bands at 2930, 2859, 2311 and 1743 cm−1 indicate the presence of the C–H stretching, C
N stretching and C–C stretching (non-conjugated) vibrational modes, respectively. The absorption peak at 1648 cm−1 indicates C–N and C–C stretching vibrations, representing the presence of proteins.40 The bands at 1522, 1462, 1366 and 911 cm−1 specify the presence of C–N stretching and N–H stretching in the amide linkages, N
O symmetry stretching in the nitro compounds, and the bonding of metal particles with oxygen, respectively.41 Therefore, it may be confirmed from the FTIR study that the extract efficiently played the dual role of capping and reducing agent. Moreover, the study revealed that there were great interactions with the Ag NPs and biomolecules of the extract. The results of the present study concurred with the findings of other reports, which revealed that the biomolecules present in the cell extracts could reduce Ag+ ions.23,24,42
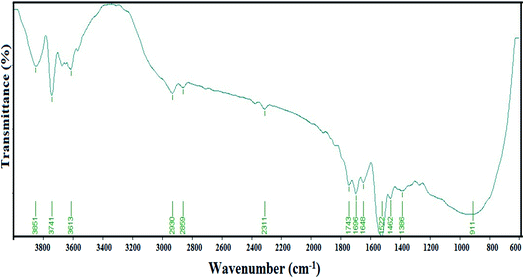 |
| Fig. 3 FTIR spectra of biogenic Ag NPs. | |
The high-resolution transmission electron microscopy (HRTEM) technique has been used to study the morphology and particle size of the as-synthesized Ag NPs. As can be seen (Fig. 4a–c) from the morphology of the Ag NPs, most of the nanoparticles were found to be of spherical shape with the particle size varying from 20–35 nm. The selected area electron diffraction (SAED) pattern (Fig. 4d) has confirmed the crystalline nature of the as-obtained Ag NPs. The presence of a quasi-ring-like diffraction pattern indicating the polycrystalline structure and the (111), (200) and (311) rings were indexed to the face-centered cubic (fcc) crystal structure of Ag, which are in good agreement with the XRD results.43,44
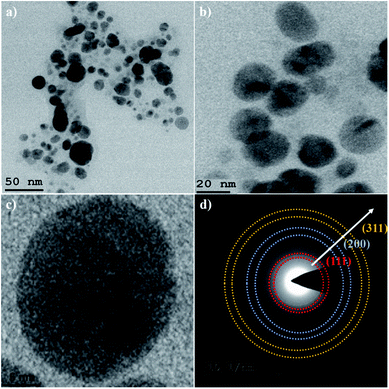 |
| Fig. 4 HRTEM images of Ag NPs at: (a) 50 nm; (b) 20 nm; (c) 5 nm bar scale and (d) SAED pattern of Ag NPs. | |
During the present study, the antibacterial activities of the biosynthesized Ag NPs were investigated for B. subtilis and E. coli. The growth rates of the selected bacterial strains were measured by their optical densities at regular intervals after treatment with a graded concentration of Ag NPs from 0 to 10 mg mL−1 (Fig. 5a and b). The results of the present study suggest that the synthesized Ag NPs were able to significantly inhibit the growth of both Gram-positive and Gram-negative bacterial strains within the first 3 hours of the experiment at 8 and 10 mg L−1 concentrations. The minimum inhibitory concentration (MIC) was found to be 8 mg L−1 for both B. subtilis and E. coli. The present investigation has confirmed that 8 mg L−1 concentration of biogenic Ag NPs synthesized using the cell-free extract of Leptolyngbya sp. WUC 59 was sufficient to act as viable bactericides.
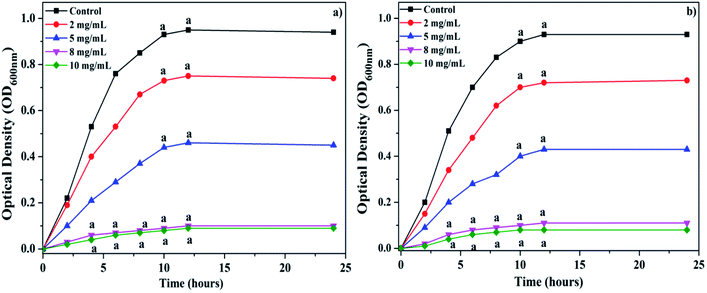 |
| Fig. 5 Growth curves of (a) B. subtilis and (b) E. coli bacterial strains at different concentrations of Ag NPs. Data at different time intervals with the same lower case letters are not significantly different from each other at the 95% confidence level (p < 0.05). | |
The antibacterial results in this investigation are in good agreement with previous reports.45,46 The precise mechanism of the mode of antibacterial action of Ag NPs is still not well described.37 It has been hypothesized that the small-sized nanoparticles may easily penetrate through the bacterial cell wall, and then interact with the various cytoplasmic biomolecules inside the cell. Roy et al.37 explained that the biocompounds that surround the surface, the charge and the size of the particles play important roles in the attraction between the bacterial cells and NPs, as well as their penetration in the cell wall. On the cell surface, adhesion of NPs to the bacterial cell wall causes a disruption of the cell integrity and finally cell death. This is achieved by disrupting the permeability of the membrane and respiratory functions via membrane depolarization triggered by the nanoparticles.47,48 It has been suggested by earlier reports that depending upon the damage of the bacterial cell wall, Ag NPs can penetrate the cell wall. The Ag NPs could then affect the functions of important biomolecules, such as DNA, proteins, lipids, and respiratory enzymes that cause oxidative stress by liberating ROS and damage of nucleic acids and proteins, which may lead to the death of the bacterial cell.37,49 Comparable antibacterial properties of Ag NPs were also described by Li et al.50 They have shown in their study that nanoparticles may enter the bacterial membrane and bring about the hindrance of typical cell growth, ultimately causing cell death. The Ag NPs synthesized from different cyanobacterial species and their applications have been compared with the present study, and are summarized in Table 1.
Table 1 Ag NPs synthesized employing various cyanobacterial species with their applications
Cyanobacterial species |
Shape of NPs |
Size (nm) |
Application |
References |
Desertifilum sp. |
Spherical |
4–26 |
Antibacterial and cytotoxicity |
23
|
Oscillatoria limnetica
|
Quasi-spherical |
3.30–17.97 |
Antibacterial and cytotoxicity |
24
|
Microcoleus sp. |
Spherical |
44–79 |
Antibacterial |
51
|
Nostoc commune
|
Approx. spherical |
15–54 |
Antibacterial sterilizing agent of seed crops against phytopathogenic fungi |
52
|
Anabaena doliolum
|
Spherical |
10–50 |
Antibacterial |
53
|
Anabaena sp. |
Irregular |
24.13 |
Antibacterial |
54
|
Synechocystis sp. 48–3 |
Irregular |
14.64 |
Antibacterial |
54
|
Synechococcus sp. |
Spherical |
15.2–266.7 |
Antibacterial and photocatalytic |
55
|
Nostoc sp. |
Agglomerated |
51–100 |
Antibacterial and antifungal |
56
|
Phormidium sp. |
Spherical |
5–10 |
Antibacterial |
57
|
Scytonema sp. |
Spherical |
11.5–12 |
Antibacterial |
57
|
Leptolyngbya JSC-1 |
Spherical |
12–50 |
Antibacterial and anticancer |
58
|
Leptolyngbya sp. WUC 59 |
Spherical |
20–35 |
Antibacterial and seed germination |
Present work |
The effects of the synthesized Ag NPs on the growth of wheat seedlings were examined by evaluating their effect on the germination percentage, root and shoot length, and the fresh and dry weights (root and shoot) of the plant at a concentration ranging from 0 to 150 mg L−1 on the 4th and 8th day of treatment (Fig. 6). The physio-chemical properties of the particles (size of NPs, shape and surface coating) and experimental conditions (concentration of NPs, exposure time, method of exposure, and cell type or plant species) are the major factors that determine the stimulatory or inhibitory effect of NPs on plant growth.59–61 A few studies have previously reported that seed treatment with nanoparticles stimulated seed germination by inducing a range of biochemical changes in the seed, such as breaking of dormancy, hydrolysis or metabolization of inhibitors, imbibitions and enzyme activation.62,63 The results of the present study indicate that Ag NPs at lower concentration slightly promote the seed germination and early seedling growth of wheat in comparison to the control. The synthesized Ag NPs either had no significant negative effect on seed germination with 25 to 75 mg L−1, or caused a significant reduction in germination with 100–150 mg L−1, in comparison to the control (Fig. 6a). Higher concentrations (>75 mg L−1) were found to significantly inhibit the seed germination in this study. Variable responses of different plants to Ag NPs have been reported by different investigators.28,64,65 The germination of the seed is the beginning of a physiological process that requires water imbibitions. However, the precise mechanism of the effect of Ag NPs on seed germination is not clearly understood. Mahakham et al.66 have proposed a hypothetical mechanism of Ag NPs inducing the seed germination of rice. These authors hypothesized that Ag NPs can enhance seed germination with at least three possible ways, including (i) the creation of the seed coat nanopores, (ii) a mild stress-induced agent or reactive oxygen species generating agent, and (iii) a nanocatalyst for enhancing starch-degrading enzyme activity. Initially, Ag NPs could interact with the cell wall of the seed coat and penetrate the seed coat by creating small pores that increase the water uptake efficiency of the seeds. During this initial phase of imbibitions, the enhanced water uptake can stimulate the higher metabolic activity and starch hydrolysis of the seed. The penetrated Ag NPs could fasten the starch hydrolysis by forming the NPs-amylase complex with α-amylase, and generate a greater amount of available sugar to support the initial embryo growth. Inside the seed, Ag NPs could generate ROS to induce the mild oxidative stress by acting as signalling molecules, and participate in the loosening of the cell wall and weakening of the endosperm. The significant increase in the shoot length of 3.0 cm and 8.0 cm at 25 mg L−1 concentration of Ag NPs was observed on the 4th and 8th day, respectively, in comparison to the control (Fig. 6b). A significant decrease in the shoot length was observed upon further increasing the concentration of Ag NPs. A similar trend was observed in the effect of Ag NPs on the root length. On the 4th and 8th days, a significant increase in the root length was observed at 25 mg L−1 concentration of Ag NPs in comparison to the control (Fig. 6b). Conversely, the higher concentration of Ag NPs caused a significant reduction in the root, as well as the shoot length. Dimkpa et al.67 reported that Ag NPs reduced the length of the shoots and roots of wheat in a dose-dependent manner. Significantly higher fresh and dry weights of the root and shoot biomasses were observed with 25 mg L−1 Ag NPs on the 4th and 8th days of growth, as compared to the control. Further increase in the Ag NPs concentration (50–150 mg L−1) caused a significant progressive reduction in these growth parameters of wheat (Fig. 6c and d). During the present study, the possible reason behind the enhanced seedling growth rate at the lower concentration (25 mg L−1) of Ag NPs could be the efficient water uptake by the treated seeds, as Ag NPs can penetrate through the seed coat and may induce the growth of the embryo. During penetration, Ag NPs may cause more new pores in the seed coat that remain helpful in fast imbibitions, efficiently leading to fast germination and growth rate. Most of the previous studies suggest that the effect of the Ag NPs on the growth of the plant was concentration-dependent.68 We also observed similar results in the present study, which showed that higher concentrations had inhibitory effects, while lower concentrations enhanced the growth of wheat. Similar effects of Ag NPs on seed germination have been reported in other crops by different workers.28,62,69
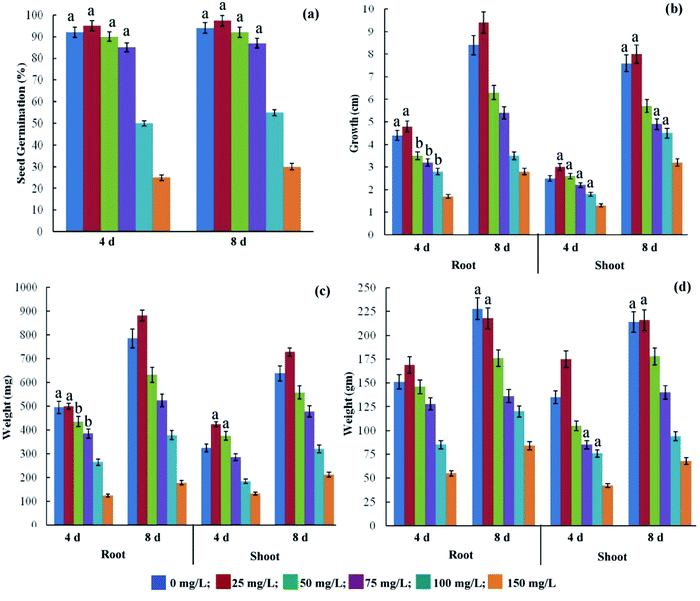 |
| Fig. 6 Effect of different concentrations (0, 25, 50, 75, 100, 150 mg L−1) of synthesised Ag NPs (SNPs) on wheat seed germination (a), root and shoot length (b), fresh weights of root and shoot (c), and the dry weights of the root and shoot (d) on the 4th and 8th days of treatment. Data at different concentrations of Ag NPs with the same lower case letters are not significantly different from each other at the 95% confidence level (p < 0.05). | |
Conclusions
In summary, we have successfully synthesized Ag NPs using the cyanobacterium Leptolyngbya WUC 59 cell-free extract. The appearance of a characteristic peak at 430 nm in the UV-Vis spectra confirmed the formation of Ag NPs. The crystallinity of the as-synthesized Ag NPs was investigated by powdered XRD technique, and observed to be face centered cubic (fcc) structure. HRTEM images verified that biogenic Ag NPs have a spherical shape with an average size of 20–35 nm. In addition, when applied as an antibacterial agent, the biogenic Ag NPs displayed appreciable antibacterial activity against both Gram-positive (B. subtilis) and Gram negative (E. coli) bacteria. Furthermore, the as-synthesized Ag NPs have shown significant potential in enhancing the seed germination and early seedling development of wheat (Triticum aestivum L.) at lower concentration (25 mg L−1). The Ag NPs accomplished from the cyanobacterium Leptolyngbya WUC 59 cell-free extract have massive potential, and might have outstanding influence on agricultural and pharmaceutical industries as biocontrol agents in the future.
Author contributions
All of the authors contributed to the data analysis, drafting, or revising of the manuscript. All authors approved the final manuscript, and agree to be accountable for all aspects of the work.
Conflicts of interest
All three authors declare no conflict of interest.
Acknowledgements
The authors (Y. S. and S. K.) are thankful to the Vice-chancellor, Sri Guru Granth Sahib World University, Fatehgarh Sahib, Punjab, for providing the necessary research facilities. The author (R. S. S.) would like to thank the Principal, Patel Memorial National College, Rajpura, Punjab, for the required laboratory facilities.
References
- K. S. Siddiqi and A. Husen, Recent advances in plant-mediated engineered gold nanoparticles and their application in biological system, J. Trace Elem. Med. Biol., 2017, 40, 10–23 CrossRef CAS.
- M. Behravan, A. H. Panahi, A. Naghizadeh, M. Ziaee, R. Mahdavi and A. Mirzapour, Facile green synthesis of silver nanoparticles using Berberis vulgaris leaf and root aqueous extract and its antibacterial activity, Int. J. Biol. Macromol., 2019, 124, 148–154 CrossRef CAS.
- R. R. Arvizo, S. Bhattacharyya, R. A. Kudgus, K. Giri, R. Bhattacharya and P. Mukherjee, Intrinsic therapeutic applications of noble metal nanoparticles: past, present and future, Chem. Soc. Rev., 2012, 41(7), 2943–2970 RSC.
- D. Chen, X. Qiao, X. Xiu and J. Chen, Synthesis and electrical properties of uniform silver nanoparticles for electronic applications, J. Mater. Sci., 2009, 44, 1076–1081 CrossRef CAS.
- Y. Huang, H. Chang and W. Tan, Cancer cell targeting using multiple aptamers conjugated on nanorods, Anal. Chem., 2008, 80(3), 567–572 CrossRef CAS.
- J. Xie, J. Y. Lee, D. I. C. Wang and Y. P. Ting, Silver Nanoplates: From biological to biomimetic Synthesis, ACS Nano, 2007, 1(5), 429–439 CrossRef CAS.
- J. Xie, J. Y. Lee, D. I. Wang and Y. P. Ting, Identification of active biomolecules in the high-yield synthesis of single-crystalline gold nanoplates in algal solutions, Small, 2007, 3, 672–682 CrossRef CAS.
- S. Ahmed, M. Ahmad, B. L. Swami and S. Ikram, A review on plants extract mediated synthesis of silver nanoparticles for antimicrobial applications: A green expertise, J. Adv. Res., 2016, 7(1), 17–28 CrossRef CAS.
- M. Hasan, W. Yang, Y. Ju, X. Chu, Y. Wang, Y. Deng, N. Mahmood and Y. Hou, Biocompatibility of iron carbide and detection of metals ions signalling proteomic analysis via HPLC/ESI-Orbitrap, Nano Res., 2017, 10, 1912–1923 CrossRef CAS.
- Z. Ji, X. Jin, S. George, T. Xia, H. Meng, X. Wang, E. Suarez, H. Zhang, E. M. V. Hoek, H. Godwin, A. Ne and J. I. Zink, Dispersion and stability optimization of TiO2 nanoparticles in cell culture media, Environ. Sci. Technol., 2010, 44(19), 7309–7314 CrossRef CAS.
- R. Hao, J. Yu, Z. G. Ge, L. Y. Zhao, F. G. Sheng, L. L. Xu, G. J. Li and Y. L. Hou, Developing Fe3O4 nanoparticles into an efficient multimodality imaging and therapeutic probe, Nanoscale, 2013, 5, 11954–11963 RSC.
- H. Zulfiqar, Z. Ayesha, N. Rasheed, Z. Ali, K. Mehmood, A. Mazher and N. Mahmood, Synthesis of silver nanoparticles using Fagonia cretica and their antimicrobial activities, Nanoscale Adv., 2019, 1, 1707–1713 RSC.
- C. Larue, H. Castillo-Michel, S. Sobanska, L. Cecillon, S. Bureau, V. Barthes, L. Ouerdane, M. Carriere and G. Sarret, Foliar exposure of the crop Lactuca sativa to silver nanoparticles: Evidence for internalization and changes in Ag speciation, J. Hazard. Mater., 2014, 264, 98–106 CrossRef CAS.
- K. Vijayaraghavan, S. P. Kamala Nalini, N. Udaya Prakash and D. Madhankumar, Biomimetic synthesis of silver nanoparticles by aqueous extract of Syzygium aromaticum, Mater. Lett., 2012, 75, 33–35 CrossRef CAS.
- S. Palanisamy, P. Rajasekar, G. Vijayaprasath, G. Ravi, R. Manikandan and N. M. Prabhu, A green route to synthesis silver nanoparticles using Sargassum polycystum and its antioxidant and cytotoxic effects: An in vitro analysis, Mater. Lett., 2017, 189, 196–200 CrossRef CAS.
- R. Kaur, S. Kaushal and P. Singh, Biogenic synthesis of silver nanoparticles-SnZrMoP nanocomposite and its application for disinfection and detoxification of water, Mater. Adv., 2020, 1–10 CAS.
- N. F. Mohd, P. Rishikesh, S. Mohd, V. Ajit, B. Ishan and P. Ram, Facile Algae-Derived Route to Biogenic Silver Nanoparticles: Synthesis, Antibacterial, and Photocatalytic Properties, Langmuir, 2015, 31, 11605–11612 CrossRef.
- Y. Singh, J. I. S. Khattar, D. P. Singh, P. Rahi and A. Gualti, Limnology and cyanobacterial diversity of high-altitude lakes of Lahaul-Spiti in Himachal Pradesh, India, J. Biosci., 2014, 39, 643–657 CrossRef CAS.
- K. Godlewska, I. Michalak, P. Pacyga, S. Basladynska and K. Chojnacka, Potential applications of cyanobacteria: Spirulina platensis filtrates and homogenates in agriculture, J. Microbiol. Biotechnol., 2019, 35, 1–18 CrossRef CAS.
- Y. Ai, S. Lee and J. Lee, Drinking water treatment residuals from cyanobacteria bloom-affected areas: Investigation of potential impact on agricultural land application, Sci. Total Environ., 2020, 706, 1–11 CrossRef.
-
J. Pathak, Rajneesh, A. S. Sonker, D. Kumar, V. Singh, R. P. Sinha, Recent Developments in Green Synthesis of Metal Nanoparticles Utilizing Cyanobacterial Cell Factories, in Nanomaterials in Plants, Algae and Microorganisms, ed. D. K. Tripathi, P. Ahmad, S. Sharma, D. K. Chauhan, N. K. Dubey, Academic Press, 2019, pp. 237–265 Search PubMed.
- A. K. Shukla and S. Iravani, Metallic nanoparticles: green synthesis and spectroscopic characterization, Environ. Chem. Lett., 2017, 15, 223–231 CrossRef CAS.
- R. Hamida, N. E. Abdelmeguid, M. A. Ali and M. M. Bin-Meferij, Synthesis of silver nanoparticles using a novel cyanobacteria Desertifilum sp. extract: Their antibacterial and cytotoxicity effects, Int. J. Nanomed., 2020, 15, 49–63 CrossRef CAS.
- R. A. Hamouda, M. H. Hussein, R. A. Abo-elmagd and S. S. Bawazir, Synthesis and biological characterization of silver nanoparticles derived from the cyanobacterium Oscillatoria limnetica, Sci. Rep., 2019, 9, 1–17 CrossRef CAS.
- A. H. Holmes, L. S. Moore, A. Sandsfjord, M. Steinbakk, S. Regmi, A. Karkey, P. J. Guerin and L. J. V. Piddock, Understanding the mechanisms and drivers of antimicrobial resistance, Lancet, 2016, 387, 176–187 CrossRef CAS.
- G. Singh, P. K. Babele, S. K. Shahi, R. P. Sinha, M. B. Tyagi and A. Kumar, Green synthesis of silver nanoparticles using cell extracts of Anabaena doliolum and screening of its antibacterial and antitumor activity, J. Microbiol. Biotechnol., 2014, 24, 1354–1367 CrossRef CAS.
- S. Singh, D. K. Tripathi, N. K. Dubey and D. K. Chauhan, Effects of nano-materials on seed germination and seedling growth: striking the slight balance between the concepts and controversies, Mater. Focus, 2016, 5, 195–201 CrossRef CAS.
- A. Razzaq, R. Ammara, H. M. Jhanzab, T. Mahmood, A. Hafeez and S. Hussain, A novel nanomaterial to enhance growth and yield of wheat, J. Nanosci. Tech., 2016, 2, 55–58 Search PubMed.
- J. Singh, S. Kumar, A. Alok, S. K. Upadhyay, M. Rawat, D. C. W. Tsang, N. Bolan and K. Kim, The potential of green synthesized zinc oxide nanoparticles as nutrient source for plant growth, J. Cleaner Prod., 2019, 214, 1061–1070 CrossRef CAS.
- R. S. Safferman and M. E. Morris, Growth characteristics of the blue-green algal virus LPP-1, J. Bacteriol., 1964, 88, 771–775 CrossRef CAS.
-
J. Komarek and K. Anagnostidis, Cyanoprokaryota 2. Teil: Oscillatoriales, in Süsswasserflora von Mitteleuropa 19/2B, ed. B. Büdel, L. Krienitz, G. Gärtner and M. Schagerl, Elsevier/Spektrum, Heidelberg, 2005 Search PubMed.
- N. Saitou and M. Nei, The neighbor-joining
method: A new method for reconstructing phylogenetic trees, Mol. Biol. Evol., 1987, 4, 406–425 CAS.
- M. Kimura, A simple method for estimating evolutionary rate of base substitutions through comparative studies of nucleotide sequences, J. Mol. Evol., 1980, 16, 111–120 CrossRef CAS.
- S. Kumar, G. Stecher, M. Li, C. Knyaz and K. Tamura, MEGA X: molecular evolutionary genetics analysis across computing platforms, Mol. Biol. Evol., 2018, 35, 1547–1549 CrossRef CAS.
- T. V. Shahbazyan, I. E. Perakis and J. Y. Bigot, Size-dependent surface plasmon dynamics in metal nanoparticles, Phys. Rev. Lett., 1998, 81, 3120 CrossRef CAS.
- E. Nazifi, N. Wada, M. Yamaba, T. Asano, T. Nishiuchi, S. Matsugo and T. Sakamoto, Glycosylated porphyra-334 and palythine-threonine from the terrestrial cyanobacterium Nostoc commune, Mar. Drugs, 2013, 11, 3124–3154, DOI:10.3390/md11093124.
- A. Roy, O. Bulut, S. Some, A. K. Mandal and M. D. Yilmaz, Green synthesis of silver nanoparticles: Biomolecule-nanoparticle organizations targeting antimicrobial activity, RSC Adv., 2019, 9, 2673–2702 RSC.
- D. M. Ali, M. Sasikala, M. Gunasekaran and N. Thajuddin, Biosynthesis and characterization of silver nanoparticles using marine cyanobacterium Oscillatoria willei NTDM01, Digest Journal of Nanomaterials and Biostructures, 2011, 6, 385–390 Search PubMed.
- B. D. Hall, D. Zanchet and D. Ugarte, Estimating nanoparticle size from diffraction measurements, J. Appl. Crystallogr., 2000, 33, 1335–1341 CrossRef CAS.
- P. Periakaruppan, P. Gnanaprakasam, R. Emmanuel and A. Selvaraj, Green synthesis of silver nanoparticles from leaf extract of Mimusops elengi Linn. for enhanced antibacterial activity against multi drug resistant clinical isolates, Colloids Surf., B, 2013, 108, 255–259 CrossRef.
- K. Jyoti, M. Baunthiyal and A. Singh, Characterization of silver nanoparticles synthesized using Urtica dioica Linn. leaves and their synergistic effects with antibiotics, J. Radiat. Res. Appl. Sci., 2016, 9, 217–227 CrossRef CAS.
- N. E. El-Naggar, A. Mohamedin, S. S. Hamza and A. D. Sherief, Extracellular biofabrication, characterization, and antimicrobial efficacy of silver nanoparticles loaded on cotton fabrics using newly isolated Streptomyces sp. SSHH-1E, J. Nanomater., 2016, 1–17 Search PubMed.
- Y. Cai, X. Piao, W. Gao, Z. Zhang, E. Nie and Z. Sun, Large-scale and facile synthesis of silver nanoparticles via a microwave method for a conductive pen, RSC Adv., 2017, 7(54), 34041–34048 RSC.
- P. R. A. F. Garcia, O. Prymak, V. Grasmik, K. Pappert, W. Wlysses, L. Otubo, M. Epple and C. L. P. Oliveira, An in situ SAXS investigation of the formation of silver nanoparticles and bimetallic silver–gold nanoparticles in controlled wet-chemical reduction synthesis, Nanoscale Adv., 2020, 2, 225–238 RSC.
- U. B. Jagtap and V. A. Bapat, Green synthesis of silver nanoparticles using Artocarpus heterophyllus Lam, seed extract and its antibacterial activity, Ind. Crops Prod., 2013, 46, 132–137 CrossRef CAS.
- Y. He, X. Li, Y. Zheng, Z. Wang, Z. Ma, Q. Yang, B. Yao, Y. Zhao and H. Zhang, A green approach for synthesizing silver nanoparticles, and their antibacterial and cytotoxic activities, New J. Chem., 2018, 42, 2882–2888 RSC.
- C. Krishnaraj, E. G. Jagan, S. Rajasekar, P. Selvakumar, P. T. Kalaichelvan and N. Mohan, Synthesis of silver nanoparticles using Acalypha indica leaf extracts and its antibacterial activity against water borne pathogens, Colloids Surf., B, 2010, 76, 50–56 CrossRef CAS.
- T. A. Abalkhil, S. A. Alharbi, S. H. Salmen and M. Wainwright, Bactericidal activity of biosynthesized silver nanoparticles against human pathogenic bacteria, Biotechnol. Biotechnol. Equip., 2017, 31, 411–417 CrossRef CAS.
- S. Kaushal, P. K. Sharma, S. K. Mittal and P. P. Singh, A novel zinc oxide–zirconium (IV) phosphate nanocomposite as antibacterial material with enhanced ion exchange properties, Colloids Interface Sci. Commun., 2015, 7, 1–6 CrossRef CAS.
- W. R. Li, X. B. Xie, Q. S. Zeng, Y. Sheng, O. Yang and Y. B. Chen, Antibacterial activity and mechanism of silver nanoparticles on Escherichia coli, Appl. Microbiol. Biotechnol., 2010, 85, 1115–1122 CrossRef CAS.
- S. S. Sudha, K. Rajamanickam and J. Rengaramanujam, Microalgae mediated synthesis of silver nanoparticles and their antibacterial activity against pathogenic bacteria, Indian J. Exp. Biol., 2013, 52, 393–399 Search PubMed.
- F. M. Morsy, N. A. Nafady, M. H. Abd-Alla and D. A. Elhady, Green synthesis of silver nanoparticles by water soluble fraction of the extracellular polysaccharides/matrix of the cyanobacterium Nostoc commune and its application as a potent fungal surface sterilizing agent of seed crops, Univers. J. Microbiol. Res., 2014, 2, 36–43 CAS.
- G. Singh, P. K. Babele, S. K. Shahi, R. P. Sinha, M. B. Tyagi and A. Kumar, Green synthesis of silver nanoparticles using cell extracts of Anabaena doliolum and screening of its antibacterial and antitumor activity, J. Microbiol. Biotechnol., 2014, 24, 1354–1367 CrossRef CAS.
- V. Patel, D. Berthold, P. Puranik and M. Gantar, Screening of cyanobacteria and microalgae for their ability to synthesize silver nanoparticles with antibacterial activity, Biotechnol. Rep., 2015, 5, 112–119 CrossRef.
- S. Keskin, N. Oya, N. Koçberber Kılıç, G. Donmez and T. Tekinay, Green synthesis of silver nanoparticles using cyanobacteria and evaluation of their photocatalytic and antimicrobial activity, J. Nano Res., 2016, 40, 120–127 Search PubMed.
- A. S. Sonker, J. Pathak, V. K. Kannaujiya and R. P. Sinha, Characterization and in vitro antitumor, antibacterial and antifungal activities of green synthesized silver nanoparticles using cell extract of Nostoc sp. strain HKAR-2, Can. J. Biotechnol., 2017, 1, 26–37 CrossRef.
- S. A. Rashed, S. A. Shehri and N. M. S. Moubayed, Extracellular biosynthesis of silver nanoparticles from Cyanobacteria, Biomed. Res., 2018, 29, 2859–2862 CrossRef.
- S. Zada, A. Ahmad, S. Khan, X. Yu, K. Chang, A. Iqbal, A. Ahmad, S. Ullah, M. Raza, A. Khan, S. Ahmad and P. Fu, Biogenic synthesis of silver nanoparticles using extracts of Leptolyngbya JSC-1 that induce apoptosis in HeLa cell line and exterminate pathogenic bacteria, Artif. Cells, Nanomed., Biotechnol., 2018, 46, 471–480 CrossRef.
- P. Cvjetko, A. Milosic, A. Domijan, I. V. Vrcek, S. Tolic, P. P. Stefanic, I. Letofsky-Papst, M. Tkalec and B. Balen, Toxicity of silver ions and differently coated silver nanoparticles in Allium cepa roots, Ecotoxicol. Environ. Saf., 2017, 137, 18–28 CrossRef CAS.
- K. Kettler, K. Veltman, D. Van de Meent, A. Van Wezel and A. J. Hendriks, Cellular uptake of nanoparticles as determined by particle properties, experimental conditions, and cell type, Environ. Toxicol. Chem., 2014, 33, 481–492 CrossRef CAS.
- R. Amooaghaie, M. R. Saeri and M. Azizi, Synthesis, characterization and biocompatibility of silver nanoparticles synthesized from Nigella sativa leaf extract in comparison with chemical silver nanoparticles, Ecotoxicol. Environ. Saf., 2015, 120, 400–408 CrossRef CAS.
- A. Yan and Z. Chen, Impacts of Silver Nanoparticles on Plants: A focus on the phytotoxicity and underlying mechanism, Int. J. Mol. Sci., 2019, 20, 1003 CrossRef CAS.
- P. Solanki and J. S. Laura, Effect of ZnO nanoparticles on seed germination and seedling growth in wheat (Triticum aestivum), J. Pharmacogn. Phytochem., 2018, 7, 2048–2052 CAS.
- J. Yang, F. Jiang, C. Ma, Y. Rui, M. Rui, M. Adeel, W. Cao and B. Xing, Alteration of crop yield and quality of wheat upon exposure to silver nanoparticles in a life cycle study, J. Agric. Food Chem., 2018, 66, 2589–2597 CrossRef CAS.
- A. A. Al-Huqail, M. M. Hatata, A. A. Al-Huqail and M. M. Ibrahim, Preparation, characterization of silver phyto nanoparticles and their impact on growth potential of Lupinus termis L. seedlings, Saudi J. Biol. Sci., 2018, 25, 313–319 CrossRef CAS.
- W. Mahakham, A. K. Sarmah, S. Maensiri and P. Theerakulpisut, Nanopriming technology for enhancing germination and starch metabolism of aged rice seeds using phytosynthesized silver nanoparticles, Sci. Rep., 2017, 7, 8263 CrossRef.
- C. O. Dimkpa, J. E. McLean, N. Martineau, D. W. Britt, R. Haverkamp and A. J. Anderson, Silver nanoparticles disrupt wheat (Triticum aestivum L.) growth in a sand matrix, Environ. Sci. Technol., 2013, 47, 1082–1090 CrossRef CAS.
- A. Razzaq, R. Ammara, H. M. Jhanzab, T. Mahmood, A. Hafeez and S. Hussain, A novel nanomaterial to enhance growth and yield of wheat, J. Nanosci. Tech., 2016, 2, 55–58 Search PubMed.
- P. Sharma, M. Deepesh Bhatt, M. G. H. Zaidi, P. Pardha Saradhi, P. K. Khanna and S. Arora, Silver nanoparticle-mediated enhancement in growth and antioxidant status of Brassica juncea, Appl. Biochem. Biotechnol., 2012, 167, 2225–2233 CrossRef CAS.
|
This journal is © The Royal Society of Chemistry 2020 |
Click here to see how this site uses Cookies. View our privacy policy here.