DOI:
10.1039/C9NH00577C
(Review Article)
Nanoscale Horiz., 2020,
5, 202-217
State-of-the-art iron-based nanozymes for biocatalytic tumor therapy
Received
2nd September 2019
, Accepted 4th November 2019
First published on 5th November 2019
Abstract
Biocatalytic tumor therapy with artificial nanoscale enzyme-mimics (nanozymes) is an emerging strategy for the therapeutic intervention of a variety of malignant conditions, which can provide the combined benefits of enzyme-dependent biocatalytic activities and nanotechnology. Generally, these novel nanocatalysts employ particular multivalent ions as the catalytic center; they have demonstrated high catalytic efficiencies, due to which they are even more potent than natural enzymes, and they also exhibit better in vivo stability, functional versatility and lower manufacturing cost. It is well established that a malignant tumor has many enzymatic mutations, which provides ample opportunities for the development of biocatalytic tumor therapy with nanozymes. Most of the current research in this area revolves around iron-based nanostructures due to their easy manufacturing process, intrinsic biocompatibility, promising physical properties, and versatile and efficient catalytic activity. A great variety of studies in the recent decade have consistently demonstrated the translational potential of iron-based nanozymes for tumor therapy. Therefore, it would be of great practical significance to summarize the previous reports on the treatment-facilitative effects and tumor cell-damaging mechanisms of iron-based nanozymes, which primarily include tumor hypoxia amelioration, Fenton-enhanced ROS damage and the activation of the ferroptosis pathway. Additionally, we also discuss the critical issues that may affect their clinical translation and future development. We hope that these iron-based nanozymes can overcome the limitations of conventional therapies and open new avenues for tumor treatment.
1. Introduction to the concept of nanozymes
Enzymes are an important class of proteins in biological organisms, which are responsible for catalyzing various biochemical reactions therein and they are essentially needed to support vital biological processes, including metabolism, detoxification and biosynthesis.1–4 Numerous studies have collectively demonstrated that enzymes are capable of accelerating the rate of certain biochemical reactions without undergoing permanent structural or chemical changes themselves, thus allowing these reactions to occur under mild conditions for sustaining normal physiological activities.5,6 Due to the critical roles of enzymes in the proper functioning of the human body, enzyme defects, such as enzymatic deficiency and abnormal functioning, would severely interfere with the biochemical cascades and cause metabolic disorders, which may drastically increase the risk of serious adverse effects.7–10 Nevertheless, the cell/tissue-damaging effects caused by enzymatic interference can also be exploited to inhibit the growth of tumor cells.11–13 Previous works have confirmed that the metabolic pattern in tumor cells changes dramatically in comparison with that in healthy cells, such as hypoxia, the high local concentration of H2O2 and acidic extracellular pH, which provide many avenues for the development of potential antitumor therapies.14 Some of the tumor-related enzymatic targets include peroxidase, catalase, selenocysteine, tyrosine kinase, cyclin, histone deacetylase and immune checkpoints, which have already demonstrated promising tumor-inhibiting efficacy in several preliminary studies.15–22 However, the clinical implementation of the existing enzyme formulations is limited by their susceptibility to the denaturation effects induced by temperature, pH, and degradation enzymes as well as the manufacturing issues associated with their cost-effectiveness and purification. Consequently, it would be of great clinical significance to develop new synthetic biocatalysts to mimic the functions of naturally occurring enzymes for inducing the catalytic interference of biochemical cascades in tumor cells.
As a result of the recent advances in nanotechnology and biochemical engineering, a wide array of synthetic enzyme mimics have already been reported with high catalytic efficiency, excellent biostability and low production cost, which are contending alternatives to human-derived enzymes in biomedical applications.23–25 As early as 1993, Tokuyama et al. first reported the biocatalytic activity of a fullerene derivative on biomacromolecules, in which the fullerene-based nanoagent could catalyze the decomposition of DNA for tumor inhibition.26 Follow-up studies further revealed that chemically modified fullerene could also demonstrate superoxide dismutase-like catalytic activity and serve as a potential antioxidant. The concept of “nanozymes” first appeared in 2004 in a report by Manea et al., in which the authors conjugated gold nanoparticles with ZnII-based ligands and used them for the cleavage of phosphate diesters.27 In the report by Yan et al. in 2007, the researchers further extended the role of nanoparticles from being mere enzyme carriers to catalytic converters.28 The research on nanozymes has progressed remarkably in the past decade, and a great variety of nanoparticulate substances have been found with varying degrees of biocatalytic activities; they can be classified into the following 3 categories: noble metal-based nanozymes (e.g., gold, silver, platinum and palladium),29 metal oxide-based nanozymes (e.g., Fe3O4, V2O5, CeO2 and iron-chelated nanocomposites)30 and carbon-based nanozymes (e.g., carbon nanotubes, graphene oxide and carbon dots).31,32
2. Overview of the biomedical applications of iron-based nanomaterials
As mentioned above, iron-based nanomaterials (INs) are some of the most successful examples of translational drugs in the pharmaceutical industry thus far, and several iron-based nanoformulations have already been approved by FDA for diagnostic and therapeutic applications on human patients.33–35 From an overall perspective, iron-based nanostructures have demonstrated excellent biocompatibility, electromagnetic functionality and biochemical activity both in vitro and in vivo. In comparison with small-molecule anticancer drugs administered via conventional routes, INs accumulate more effectively at the tumor site due to the enhanced permeability and retention (EPR) effect and via controllable magnetic guidance.36–39 INs can also be easily endowed with a variety of new functionalities due to their versatile surface chemistry, which is highly desirable for the development of personalized medicine.40,41 Furthermore, most INs have intrinsic contrast ability required for the in situ imaging of tumor tissues, which allows monitoring the malignant progression, as well as determining the therapeutic response.42–44 In addition to these functional benefits, there are a lot of convenient synthesis methods for the rapid large-scale manufacture of INs at relatively low costs, and the products may have excellent morphological and compositional consistency.45–47 These remarkable properties make INs highly relevant for antitumor applications.
The continuous research in this area has revealed more exciting capabilities of iron-based nanoplatforms, which could be exploited for the development of more advanced antitumor therapeutics with unprecedented performance. In the study by Chen et al. in 2012, the authors discovered that Fe3O4 and Fe2O3 nanoparticles demonstrated catalase-like biocatalytic activity under physiological pH (mildly basic) and converted the excessive reactive oxygen species (e.g. H2O2) in the tumor cells to oxygen and water and consequently, significantly relieving the oxidative stress therein.48 Additionally, it was observed that the catalytic activity of INs was highly dependent on the environmental pH, as experimental evidence confirmed that, under acidic pH, the catalytic property of the Fe3O4 nanoparticles switched to a peroxidase-like pattern, and the intratumoral H2O2 was converted into hyper-reactive hydroxyl radicals (˙OH). This phenomenon could significantly enhance the ROS-induced cellular damage and can potentially improve the efficacy of some ROS-dependent therapies. Based on the collective insights from recent reports, this review seeks to provide a comprehensive summary of the diagnostic/therapeutic implementations of biocatalytic iron-based nanosystems, with special emphasis on their material composition and antitumor mechanisms. It is anticipated that these technological details would facilitate the development of more advanced iron-based nanozymes with enhanced antitumor potency and theranostic capability (Scheme 1).
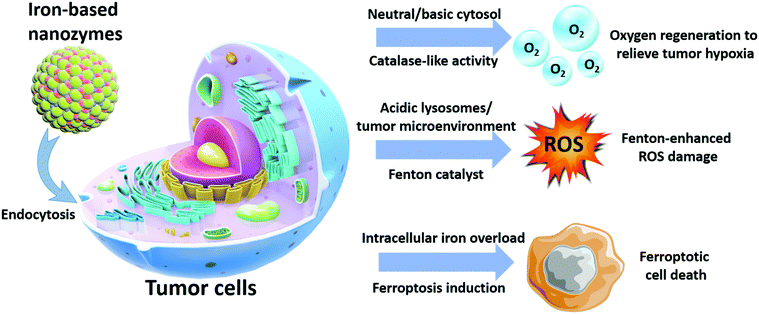 |
| Scheme 1 Antitumor mechanisms of iron-based nanozymes. | |
3. Mechanistic analysis of iron-based nanozyme-mediated biocatalysis
The mechanism of the iron-based nanozyme-mediated biocatalytic process has already been demonstrated in series of reports, in which the kinetic patterns were almost identical to that of typical enzymes.49–51 Nevertheless, the field of synthetic nanozymes is still a relatively new field, and most details on their dynamics and kinetics remain underexplored. It is widely accepted that the biocatalytic properties of iron-based nanozymes are profoundly dependent upon the pH conditions of the surrounding environment. Typically, under acidic pH, these nanozymes demonstrate peroxidase-like catalytic activity towards H2O2, which would lead to an increase in local oxidative stress and toxic risk. In comparison, when the environmental pH is neutral or mildly basic, the iron-based nanozyme would act as a catalase and convert H2O2 into biologically safe species, including oxygen and water. Both catalytic routes are shown below.
Peroxidase-like biocatalytic reactions of iron-based nanozymes under acidic pH:
| H2O2 + Fe2+ → Fe3+ + HO− + HO | (1) |
| R˙ + Fe3+ → Fe2+ + non-radical product (termination) | (3) |
| H2O2 + HO˙ → HO2˙ + H2O | (4) |
| Fe2+ + HO˙ → Fe3+ + HO− | (5) |
Peroxidases are a large group of enzymes that employ hydrogen peroxide or organic hydroperoxides as the substrate molecules and convert the hydroperoxide functional groups into free radicals to react with other hydrogen donors for their reduction in biological organisms. The catalytic activity of peroxidases usually stems from their porphyrin heme cofactor. As for those iron-based nanozymes with peroxidase-like catalytic activity, the optimal catalytic conditions are similar to those of natural peroxidases, such as horseradish peroxidase, which favor an ambient temperature in the range from 37 to 40 °C and acidic pH in the range of 3–6.5. It can be observed from the reaction route shown above that during the nanozyme-mediated peroxidase-like reduction of H
2O
2, the Fe
2+ ions are firstly oxidized by H
2O
2 to generate hydroxyl radicals and Fe
3+. The hydroxyl radicals then capture protons from hydrogen donors and reduce to water, while the Fe
3+ ions recycle into Fe
2+ by reacting with the reductive products to complete the cycle.
Catalase-like biocatalytic reactions of iron-based nanozymes under neutral/basic pH:
| H2O2 + Fe3+ → H2O + FeOOH2+ | (6) |
| Fe2+ + H2O2 → Fe3+ + HO− + OH˙ | (8) |
| OH˙ + HO2˙/O2− → H2O + O2 | (10) |
Catalase is another type of heme-group containing enzymes that relieve oxidative stress in biological organisms and is capable of degrading H
2O
2 into water and oxygen. It is known that iron oxide-based nanozymes demonstrate similar catalytic activities under neutral and basic pH. The shift in their catalytic patterns when the pH value increases is possibly due to the accelerated rate of
reactions (6) and (7), which leads to the enhanced production of HO
2˙. HO
2˙ is then ionized into O
2− through
reaction (9), and the HO
2˙/O
2− complex later reacts with OH˙ to give water and oxygen, while the Fe
2+ ions regenerate into Fe
3+ after oxidation by environmental H
2O
2viareaction (8).
Nevertheless, despite the mechanistic similarities between the peroxidase-like biocatalytic activity of iron-based nanozymes and the Fenton reactions catalyzed by conventional iron-based Fenton agents, only a minimal amount of iron ions were observed during the iron-based nanozyme-catalyzed ROS conversion, which suggested the existence of new catalytic pathways.52 A possible reason for these observations is that their crystal structures consist of both Fe2+ and Fe3+ ions, whose relative distribution ratio could also affect the eventual catalytic efficiency.48,53,54 Specifically, under acidic pH, Fe2+ ions are the dominant catalytic species during the H2O2 to ˙OH conversion, and therefore increasing the Fe2+ content in the iron-based nanozymes could significantly enhance their catalytic efficiency in the Fenton reactions. On the contrary, when the environmental pH changes to neutral or basic, the Fe3+ content in the iron-based nanozymes becomes the dominant catalytic species, favoring the catalase-like decomposition of H2O2. Further quantitative analysis revealed that, although trace amounts of iron ions were released during the reactions, the primary active sites were still the Fe atoms on the nanoparticle surface, which are similar to the iron-chelated heme groups in both peroxidase and catalase.55,56 These insights demonstrate that the catalytic properties of iron-based nanozymes can be tuned via surface engineering to meet the demands of specific applications.
4. Tumor hypoxia reversal based on catalase-like biocatalytic activity
A defining feature of various malignant tumors is that the tumor cells are highly resistant to apoptosis and proliferate in an uncontrolled manner.57,58 The rapid development of the malignant tissues would outgrow the blood supply, resulting in the emergence of oxygen-deprived tumor regions, in which the oxygen level is significantly lower than that in the healthy tissues.59,60 The resulting tumor hypoxia fundamentally changes many aspects of tumor cell behavior and is also associated with metabolic remodeling.61,62 For instance, tumor cells frequently exhibit high rates of glycolysis instead of oxidative phosphorylation to generate sufficient ATP to sustain their bioactivities. Moreover, clinical evidence also indicates that the hypoxic tumor regions are more prone to necrosis, which may further limit the efficacy of existing antitumor therapies.63,64 Tumor hypoxia is particularly counter-productive towards oxygen-consuming treatment modalities, such as photodynamic therapy and radiotherapy.65,66 Due to the important role of hypoxia in tumor treatment resistance, it is highly valuable to develop oxygen-generating agents to locally reverse the oxygen-deprived state in tumors.
4.1 Iron oxide-based nanozymes
Previous studies have confirmed that tumor cells frequently secrete large amounts of hydrogen peroxide due to their rapid proliferation and intense metabolism.67–70 Consequently, there has been continual interest in using iron-based nanozymes to decompose intratumoral H2O2 for in situ oxygen replenishment by exploiting their catalase-like catalytic activity. The technological feasibility and potential therapeutic benefit of this strategy were comprehensively demonstrated in the study by Li et al., in which the authors deposited Fe3O4 nanoparticles onto photothermal PtFe substrates for enhanced photothermal therapy against deep pancreatic cancer (Fig. 1).71 Experimental results revealed that biocatalysis-induced oxygen replenishment significantly reduced hypoxia-induced resistance to treatment. Moreover, the authors reported that the catalase-like catalytic efficiency of Fe3O4 could further be augmented through direct electron transfer between the Fe3O4 nanoparticles and the PtFe substrate under near-infrared light. In another study by Yang et al., the authors loaded amine-functionalized iron oxide nanoparticles into microbubbles, which could release hydroxyl radicals in the lysosomal compartment for biocatalytic therapy.72 After their release into the tumor cytosol, the iron oxide nanoparticles reacted with cytosolic H2O2 to produce oxygen in a self-immolating manner, which significantly improved the long-term biosafety of the iron-oxide-based nanoformulation. Recent investigations offer new opportunities to further enhance the catalytic activity via surface engineering. In a study by Fan et al. in 2017, the authors reported that the catalase-like catalytic efficiency of Fe3O4 nanozymes could be enhanced by more than 20 folds by simply modifying their surface with histidine residues.73 According to the mechanistic investigation of the catalytic process, the authors concluded that the possible mechanism underlying the drastic increase in H2O2 affinity and catalytic efficiency is that the imidazole side-chains in the histidine moieties bind to H2O2via hydrogen bonding, the configuration of which is very similar to that of the endogenous enzymes. These insights can potentially facilitate the development of new methods for improving the biocatalytic efficiency of iron oxide-based nanozymes in the reoxygenation of hypoxic tumors.
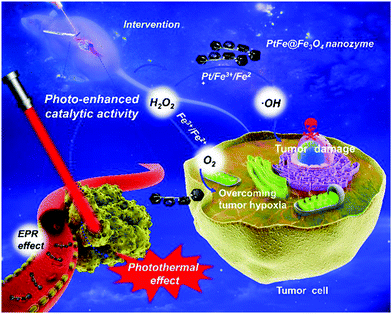 |
| Fig. 1 Schematic illustration of the photo-enhanced catalase-like activity of Fe3O4 nanoparticles deposited on PtFe substrates. The PtFe substrate absorbs the incident NIR light to enhance the catalytic efficiency of the Fe3O4 nanoparticles, while oxygen generated by the Fe3O4-catalyzed H2O2 decomposition reduces the hypoxia-induced tumor resistance towards photothermal therapy, thereby achieving complementary benefits in the treatment of deep pancreatic tumor. Copyright 2019 Wiley. Reproduced with permission from Angew. Chem., Int. Ed.71 | |
4.2 Prussian blue-based nanozymes
In addition to iron oxide nanostructures, other iron-containing nanocompounds have demonstrated H2O2-decomposition capability and could be exploited for tumor reoxygenation to facilitate oxygen-consuming therapies. Typically, it has been reported that Prussian blue nanostructures (Fe4[Fe(CN)6]3, PB) have multi-enzyme-like capabilities, including those of peroxidase, catalase and superoxide dismutase, using which ROS could be converted to less cytotoxic compounds.74–76 Consequently, Prussian blue nanoparticles (PBN) could be used as ROS scavengers to relieve the oxidative stress, while also offering the promise of in situ oxygen regeneration. The multienzyme-like catalytic activity of PBNs is likely caused by the abundant redox potential among the different compositional domains including Prussian blue, Prussian white, Berlin green and Prussian yellow (Fig. 2).77 A typical example in this field was reported by Peng et al., in which the authors coated Prussian blue/manganese dioxide hybrid nanoparticles (PBMn) with erythrocyte membranes and used them for combinational chemotherapy/photothermal therapy against MCF-7 tumors in mouse models.78 This nanosystem showed a high loading capacity for doxorubicin, as the amphiphilic anticancer drug could be intercalated into the RBC membrane, tethered onto the PBMn surface or dissolved in the encapsulated liquid drop. Under the illumination of the 808 nm laser, the PBMn core could generate heat via thermal relaxation79–81 while converting intratumoral H2O2 into oxygen. The photothermal heating and oxygen generation also disrupted the RBC membrane coating, leading to a rapid release of the encapsulated DOX molecules. In another report by Zhou et al., the authors modified Prussian blue nanocubes (PBNCs) with hyaluronic acid for concurrent photodynamic/photothermal therapy.82 The hyaluronic acid ligands could enhance the aqueous dispersity of the PBNs while also endowing the targeting effect against CD44-overexpressing tumors. Under the near-infrared laser at 808 nm, the PBNs could confer strong photothermal damage to the tumor cells for therapeutic purposes. Meanwhile, the PBNs could catalyze the decomposition of intratumoral H2O2 into oxygen, which was subsequently converted into cytotoxic singlet oxygen via the NIR-induced energy transfer process in the PBN substrate for photodynamic therapy. The report by Luo et al. further demonstrated that the catalase-like biocatalytic activity of Prussian blue nanoparticles could be combined with endogenous enzymes for enhanced antitumor efficacy. Specifically, the authors loaded glucose oxidase (GOD) into hollow Prussian blue nanoshells to achieve a cascading therapeutic effect, in which the GOD first decomposed intratumoral glucose to limit the tumor energy supply while also generating H2O2 as the by-product. H2O2 was then turned into oxygen by the Prussian blue nanoshells to reduce the hypoxia-induced tumor resistance towards photothermal therapy.
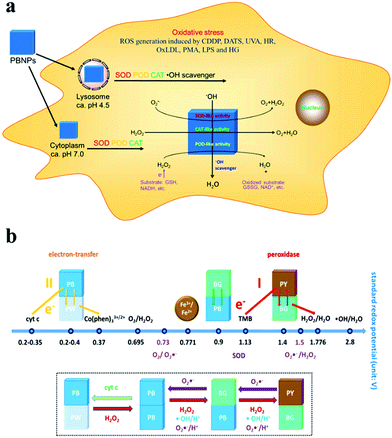 |
| Fig. 2 Schematic illustration of the (a) biological impact and (b) biocatalytic mechanism of Prussian blue nanoparticles in H2O2-rich tumor intracellular environment. Reproduced with permission from ref. 77. Copyright © 2016, American Chemical Society. | |
4.3 Nanocarriers loaded with biocatalytic iron species
By taking advantage of the advances in nanofabrication technologies, it is now possible to integrate iron-based enzyme mimics with other nanocarriers for enhanced therapeutic benefit. One of the most noteworthy examples is the mesoporous silica nanoparticle (MSN), which has shown excellent biocompatibility and well-characterized therapeutic performance in drug delivery applications.83,84 Typically, in a recent report by Wang et al., the authors prepared a Prussian blue/mesoporous silica core/shell nanoplatform and loaded it with zinc phthalocyanine (ZnPc) for NIR-actuated photothermal/photodynamic therapy.85 The PB core functioned as both the NIR-responsive heat source and the H2O2-to-O2 catalyst, resulting in a dramatic local temperature increase and oxygen replenishment. Due to the overlap in the light absorption spectra of PB and ZnPc, the NIR laser treatment also readily activated the ZnPc molecules to more efficiently produce singlet oxygen, leading to enhanced PDT efficacy. It should also be noted that this nanoplatform was constructed with only FDA-approved compounds, which is highly preferable for clinical translation. Yang et al. coated Prussian blue nanoparticles with Chlorin e6 (Ce6)-anchored periodic mesoporous organosilica (PMO) to biocatalytically enhance photodynamic therapy.86 The addition of PMO coating greatly facilitated the loading of the Ce6 photosensitizers. The PB core could not only decompose H2O2 in the tumor intracellular environment to generate oxygen, but also sequentially converted the oxygen into cytotoxic singlet oxygen by receiving photon energy from Ce6 via energy transfer. Besides the Prussian blue nanostructures, researchers found that some other types of iron-containing nanoparticles also possessed catalase-like biocatalytic activity against H2O2, which could be used for the reoxygenation of hypoxic tumor tissues. For instance, manganese/iron oxide nanoparticles, a class of known T2 contrast agents, were exploited by Yin et al. as a potential catalase-like biocatalyst against hypoxic tumors.87 The authors coated polyvinyl pyrrolidone (PVP)-stabilized manganese ferrite (MnFe2O4) nanozymes with tetrakis(4-carboxyphenyl)porphyrin (TCPP)-based MOF for enhanced photodynamic therapy, which could simultaneously regulate tumor hypoxia and the redox potential in a continuous manner. Experimental results confirmed that the MnFe2O4@MOF hybrid structure had catalase/glutathione peroxidase dual enzyme-like catalytic property, which could rapidly generate oxygen to reverse the tumor hypoxia while consuming the intracellular glutathione (a major antioxidant capable of scavenging ROS generated via PDT) to amplify the PDT damage. Both enzymatic features greatly contributed to the eventual success of the treatment and significantly prolonged the survival of the tumor-bearing mouse models. Alternatively, Yang et al. devised an iron-based catalase-like nanozyme using a DNA polymer (Fig. 3).88 The DNA nanostructure was synthesized via the self-regulated coordination of calcium ions (Ca2+) and AS1411 DNA G quadruplexes, and both Ce6 (photosensitizer) and hemin (iron-chelated porphyrin) were efficiently encapsulated in the cavities. Upon reaching the hypoxic tumor microenvironment, the iron ions in the hemin molecules could catalyze the decomposition of H2O2 to enhance the photodynamic efficiency. Hu et al. developed a Fe(III)-coordinated nanoscale carboxylate MOF to encapsulate Ce6 for switchable photodynamic therapy. Under normal conditions, the PDT activity of the Ce6 molecules was in a switched-off state as they were isolated from oxygen by the MOF structure. Upon reaching the H2O2-rich tumor intracellular environment, the Fe3+-mediated oxygen generation led to the collapse of the MOF and the release of the Ce6 molecules, allowing PDT to be activated specifically in the H2O2-elevated tumor region with sufficient oxygen supply. In addition to these synthetic nanobiomaterials, Zhang et al. demonstrated that human-derived holo-lactoferrin also has catalase-like enzymatic capability due to the chelated iron ions.89 When holo-lactoferrin was used as a molecular ligand to functionalize liposomes, it not only confer targeting effect against tumor cells overexpressing transferrin receptors through specific ligand–receptor binding, but also served as a biocatalyst for the conversion of H2O2 to oxygen to ameliorate tumor hypoxia, which reduced the hypoxia-induced tumor resistance to concurrent radiotherapy.
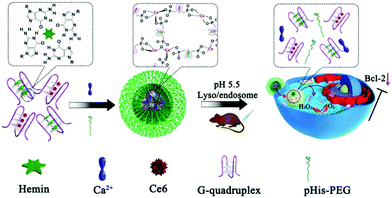 |
| Fig. 3 Structural composition of the iron-loaded G-quadruplex-based DNA nanopolymer. The iron-containing hemin molecules act as a catalase-like catalyst for the conversion of H2O2 to oxygen for enhanced ROS production. Reproduced with permission from ref. 88. Copyright © 2018, American Chemical Society. | |
5. Fenton-enhanced ROS damage with iron-based nanozymes
It is well acknowledged that many antitumor therapies, such as photodynamic therapy, radiotherapy and artemisinin derivatives, elevate the cellular level of reactive oxygen species (ROS) to exert cell-damaging effects.90,91 ROS confer indiscriminate damage to various biomolecules and subcellular organelles and play an important part in the execution of autophagy and apoptosis. It has been observed that tumor cells express an increased amount of antioxidants, such as GSH and bioreductive proteins, to counter the enhanced oxidative stress, which may potentially compromise the efficacy of these ROS-dependent therapies.92,93 Consequently, new treatment approaches are critically needed to enhance the cytotoxic potency of ROS. Fenton reaction-based therapy is a promising approach among emerging therapeutic strategies. Previous studies have revealed that H2O2 in tumor cells is a rather poor oxidant with low cytotoxicity. However, H2O2 can be converted into hydroxyl radicals (˙OH) when catalyzed by Fe2+ ions viareaction (5),94,95 leading to higher reactivity against various biomolecules and potentially greater cytotoxicity. Typically, the hydroxyl radicals react with almost all kinds of biomacromolecules, including carbohydrates, proteins, nucleotides and lipids, and cause severe adverse effects, such as lipid peroxidation, DNA mutation and protein damage.96,97 Meanwhile, the Fe3+ ions (oxidation product) react with H2O2 and are recycled into Fe2+, leading to the efficient regeneration of the Fenton catalyst.98 Based on this concept, a plethora of nanozymes have been reported for tumor therapy through the in situ generation of hydroxyl radicals via an iron-catalyzed Fenton reaction.
5.1 Iron oxide-based Fenton nanocatalyst
Due to the less difficult mass-scale preparation process of iron oxide nanoparticles, as well as their well-characterized biochemical properties, they were employed as the first-generation Fenton catalysts for ROS-based tumor therapy.40,99,100 In 2013, Huang et al. first reported a superparamagnetic iron oxide nanoparticle (SPION)-based platform for enhancing the antitumor efficacy of β-lapachone (β-lap).101 SPIONs could gradually degrade in the tumor intracellular environment to release iron ions, and β-lap is an FDA-approved anticancer drug that can generate H2O2 in tumor cells. The results demonstrated that the β-lap-induced H2O2 could be then converted to hydroxyl radicals when catalyzed by the released iron ions, and the sequential action of β-lap and SPION eventually led to amplified ROS stress, thus enhancing the therapeutic effect. In another report by Ma et al., the authors used iron oxide nanoparticles as carriers for the EPR-facilitated tumor-targeted delivery of cisplatin(IV) prodrug, which caused potent oxidative damage in the tumor cells (Fig. 4).102 The kinetically inert cisplatin(IV) prodrug showed low cytotoxicity under normal conditions during transportation but could be activated by intracellular bioreductive agents, such as glutathione, to form a more cytotoxic cisplatin version (Pt(II)), which led to the in situ generation of abundant superoxide anions (O2˙−). The newly generated O2˙− was then dismutated by the endogenous superoxide dismutase into H2O2. Meanwhile, the iron oxide nanocarrier hydrolyzed in the acidic tumor intracellular environment in a self-immolating manner and released Fe2+/Fe3+ ions into the tumor cytosol to catalyze the conversion of cisplatin-induced H2O2 into highly toxic hydroxyl radicals via the Fenton reaction. The combination of cisplatin(IV) prodrug and self-immolating iron oxide nanoparticles was beneficial in enhancing the antitumor efficacy while lowering the collateral damage to healthy cells. A similar damage-amplification mechanism was also employed by Hauser et al. for enhanced radiotherapy. In this study, the authors conjugated iron oxide nanoparticles with a cell-penetrating peptide (TAT), which could facilitate the lysosomal escape of the nanoparticles after endocytic uptake. Moreover, as radiotherapy could promote mitochondrial respiration in tumor cells and increase the production of superoxide anions, the TAT-modified iron oxide nanoparticles were capable of achieving a synergistic effect with radiation treatment by catalyzing the conversion of the emerging ROS into hydroxyl radicals.103
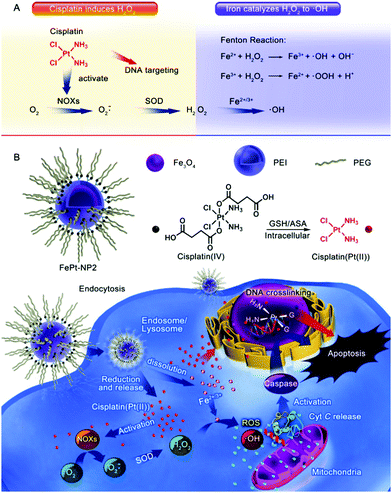 |
| Fig. 4 Schematic illustration of the (A) sequential synergy between cisplatin-enabled chemotherapy and iron-catalyzed Fenton reaction and (B) the process of nanoplatform construction and its therapeutic mechanism. Reproduced with permission from ref. 102. Copyright © 2017, American Chemical Society. | |
5.2 Non-conventional iron-based nanostructures
The initial success of Fenton reaction-dependent tumor therapy with iron oxide nanoparticles greatly inspired the research on iron-based Fenton biocatalysts, which revealed the therapeutic potential of several other iron nanostructures. For instance, Liu et al. developed a therapeutic nanoplatform to simultaneously disrupt the metabolic and ROS homeostasis in tumor cells, which essentially consisted of amorphous iron oxide nanoparticles (AIO NP) encapsulating an MCT4-targeted siRNA (siMCT4).104 The AIO NPs rapidly decomposed in the acidic tumor environment to catalyze the production of hydroxyl radicals, while the simultaneous release of siMCT4 was capable of blocking the excretion of lactate and H+ to exacerbate tumor acidity, leading to accelerated Fe release and enhanced oxidative stress. Alternatively, Tang et al. synthesized an antiferromagnetic pyrite-based antitumor nanosystem with Fenton-based biocatalytic and photothermal capabilities (Fig. 5).105 The FeS2 content could be oxidized by the excessive H2O2 in the tumor microenvironment to initiate the Fenton reaction, and the intrinsic photothermal effect of the FeS2 nanocubes under NIR illumination could further accelerate the Fenton process to enhance the production rate of hydroxyl radicals. Meanwhile, the increasing valence state of the iron ions on the nanoparticle surface after H2O2 oxidation contributed to enhanced T1 and T2 signals under MR imaging, which could be used to guide the combinational therapy. Instead of the iron compounds discussed above, Zhang et al. prepared iron nanometallic glass to induce Fenton reactions in tumor cells, which showed significantly higher reactivity than crystalline iron nanostructures.106 The high activity and versatility demonstrated by these examples may facilitate the development of more potent biocatalytic therapies.
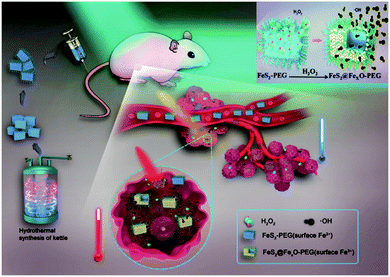 |
| Fig. 5 Surface oxidization mechanism of the FeS2-PEG nanoparticles and the self-enhancing combinational ROS and photothermal therapy. Reproduced with permission from ref. 105. Copyright © 2017 WILEY-VCH Verlag GmbH & Co. KGaA, Weinheim. | |
5.3 Synergistic interaction between iron oxide nanocatalyst and endogenous enzymes
Iron-based nanozymes have also been frequently combined with other nanobiomaterials for additional functionality and potential benefits. For instance, as the Fenton reaction is an H2O2-consuming process, the therapeutic efficacy of Fenton catalysts is intrinsically restricted by the limited amount of endogenous H2O2 in tumor tissues.107 A promising strategy to sustain the ˙OH production is to develop composite nanomaterials integrated with H2O2-evolving endogenous enzymes. In the study by Huo et al., the authors reported a viable approach to replenish intratumoral H2O2 using glucose oxidase (GOD) (Fig. 6).11 GOD is a natural enzyme capable of oxidizing glucose into D-glucono-δ-lactone while producing H2O2 as a by-product. Dendritic mesoporous silica nanoparticles with a large pore size (∼40 nm) were employed as the catalyst support for both GOD and the ultrasmall Fe3O4 nanoparticles (diameter: 2 nm). After being taken in by the tumor cells, GOD could continuously catalyze the glucose oxidation reaction and produce H2O2 to support the iron-mediated Fenton reaction. An additional benefit of this design is that D-glucono-δ-lactone cannot be metabolized by the tumor cells as an energy source. Therefore, the GOD treatment may also deplete the intratumoral glucose to achieve a tumor starvation effect for enhanced growth inhibition. The sequential interaction between GOD and iron-based Fenton catalysts was also demonstrated in another report by Feng et al., in which the authors conjugated GOD onto the surface of Fe3O4-doped polypyrrole nanoparticles.108 In addition to the increase in H2O2 supply via GOD-mediated glucose oxidation, the photothermal effect of the polypyrrole substrate under NIR illumination in both the first and second biowindows was exploited to improve the disproportionation efficiency of H2O2 to enhance the generation of hydroxyl radicals. Meanwhile, the composite nanoplatform could be used for photoacoustic and MR imaging of the tumor tissues, owing to the polypyrrole and Fe3O4 components, respectively. Alternatively, Ke et al. encapsulated GOD and Fe3O4 nanoparticles into camptothecin prodrug-conjugated polymersomes for combinational chemodynamic therapy/chemotherapy.109 As the camptothecin molecules were conjugated to the polymer backbone via ROS-sensitive thioketal linkers, it was anticipated that the hydroxyl radicals generated via the Fenton reaction would also trigger the release of CPT for tumor-specific treatment.
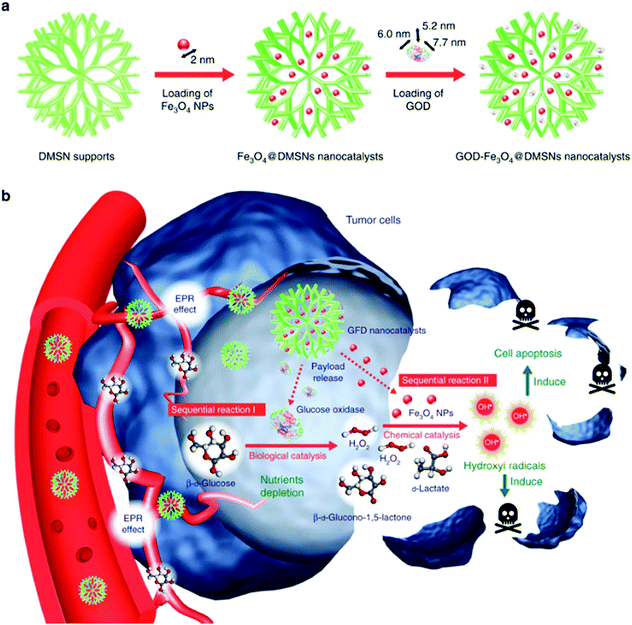 |
| Fig. 6 (a) Fabrication process and (b) the therapeutic mechanism of the GOD/Fe3O4 NP-loaded dendritic mesoporous silica nanoparticles. GOD first decomposes intratumoral glucose to produce H2O2, which is then converted into highly cytotoxic ˙OH by the Fe3O4 nanocatalysts, leading to sequentially enhanced therapeutic efficacy against tumor cells. Reproduced with permission from ref. 11. Copyright © 2017, Springer Nature. | |
5.4 Integration of iron-based nanozyme with other biocatalytic metallic species
There are also reports on the combination of iron with other metallic compounds to enhance their therapeutic performance. For instance, MnO2, an inorganic compound that can be decomposed to release oxygen, is often integrated with an iron-based Fenton catalyst for enhanced ROS therapy.110,111 Moreover, Mn ions have Fenton-like catalytic activity similar to iron ions, which can potentially improve the generation efficiency of hydroxyl radicals.112,113 In the study by Feng et al., the authors used a MnO2 shell to encapsulate GOD-modified Fe5C2 nanoparticles for ROS therapy of tumors (Fig. 7).114 The decomposition of MnO2 after endocytosis could relieve the tumor of hypoxia while releasing the GOD bound on the Fe5C2 surface. Tumor reoxygenation by MnO2 decomposition facilitated GOD-mediated glucose oxidation, leading to an increase in H2O2 content in the tumor cytosol, as well as depletion of glucose for tumor starvation. The elevated H2O2 level was also beneficial for the Fe/Mn-catalyzed Fenton reaction to generate the cytotoxic ˙OH radicals. MnFe2O4 nanoparticle is another efficient catalyst for H2O2-to-O2 conversion, and its efficacy in biocatalytic tumor therapy was demonstrated in a recent report by Kim et al., in which the authors revealed that MnFe2O4 nanoparticles could continuously decompose intratumoral H2O2 and produce O2 to overcome hypoxia-induced PDT resistance.115 Similar Fenton-enhancing mechanisms have also been realized using Fe2O3/Gd2O3 hybrid nanoparticles by Shen et al.116 Recently, Xiao et al. demonstrated that introducing selenium into Fe/Mn-based Fenton nanocatalysts could further enhance cell apoptosis via ˙OH production.117 Specifically, selenium could promote the production of superoxide anion radicals in tumor cells, which were later converted to H2O2 by superoxide dismutase (SOD) to sustain the Fe/Mn-mediated Fenton reaction.
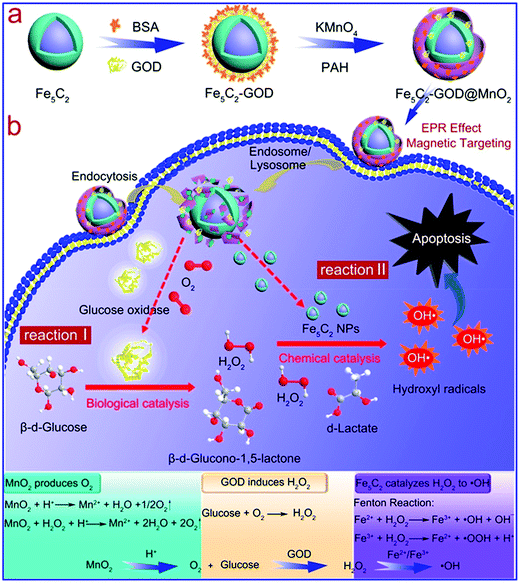 |
| Fig. 7 Schematic illustration of the (a) construction of the GOD-loaded Fe5C2/MnO2 core/shell nanosystem and (b) the sequential synergy between the catalytic activities of GOD and Fe5C2. Reproduced from ref. 114. Copyright © 2018, American Chemical Society. | |
5.5 Fenton nanocatalyst employing single iron atom as the active site
It is well-established that size is a crucial factor in determining the catalytic efficiency of metal-based catalysts, for which the specific activity per metal atom usually increases when the nanoparticle size decreases.118–120 Consequently, there has been an increasing trend in developing single-atom Fenton catalysts for tumor therapy by using coordinated iron ions as the catalytically active site, which is anticipated to provide enhanced activity or selectivity. In a recent study by Dong et al., the authors reported a single-atom liposomal Fenton catalyst based on the complexation between gallic acid and a ferrous ion (GA–Fe(II)), which could enhance the efficacy of concurrent chemotherapy and radiotherapy (Fig. 8).121 Taking advantage of the gallic acid-mediated conversion of Fe3+ to Fe2+ and the formation of the GA–Fe(II) nanocomplex, the liposomal nanoplatform showed significantly improved catalytic stability compared to free Fe2+ ions and could continuously catalyze H2O2 to ˙OH conversion via the Fenton reaction. Moreover, the liposomal nanocarrier was also loaded with L-buthionine sulfoximine (GSH inhibitor) to deplete the intratumoral antioxidants and amplify the ROS damage. In another study by Ding et al., the authors developed a polymeric nanoparticle through the coordination between Fe2+ ions and 1,5-bis[(L-proline-1-yl) diazen-1-ium-1,2-diol-O2-yl]-2,4-dinitrobenzene (BPDB), which is capable of releasing NO after reacting with glutathione.122 In the H2O2-rich tumor microenvironment, the Fe2+ ions could convert H2O2 into hydroxyl radicals via the Fenton reaction to exert potent ROS damage. Moreover, it also generated superoxide anions via the Haber–Weiss reaction with H2O2, which sequentially reacted with NO generated during the reduction of BPDB to produce the cytotoxic peroxynitrite anions, thereby achieving synergistic NO- and biocatalysis-enabled ROS therapy. Alternatively, Ranji-Burachaloo et al. synthesized a novel iron-coordinated MOF via a hydrothermal method with FeCl3 and 2-aminoterephthalic acid.123 The release of the iron ions from the MOF could be triggered by the acidic tumor microenvironment, consequently limiting Fenton-induced ROS damage only to the tumor area.
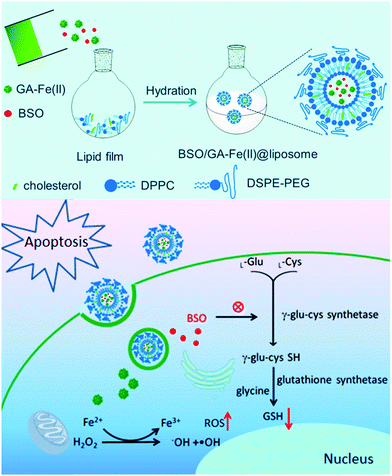 |
| Fig. 8 The preparation process of iron-loaded liposomes and the mechanism underlying the amplification of Fenton-induced ROS stress due to GSH depletion. Reproduced with permission from ref. 121. Copyright © 2019, American Chemical Society. | |
6. Ferroptosis activation with iron-based nanozymes
Most of the iron-based therapeutic approaches discussed above exert cell-damaging effects by activating the intrinsic apoptosis pathways. Nevertheless, due to the heterogeneity and complexity of the tumor microenvironment, as well as the counteractive resistance induced by monoclonal evolution, the efficacy of the apoptosis-dependent therapies often abates on prolonged treatment.124–126 Therefore, it is important to find new molecular mechanisms to trigger cell death in order to address these critical challenges.
6.1 Definition of ferroptosis and its therapeutic relevance
Ferroptosis is a recently discovered form of iron-dependent regulated cell death that features impaired antioxidant defenses and accumulation of lipid peroxides, which is fundamentally different from other known forms of regulated cell death both in terms of the morphological features and the biochemical machinery involved.127,128 The term “ferroptosis” was coined by Stockwell et al. in 2012 and describes a series of catastrophic events initiated by the failure of glutathione peroxidase 4 (GPX4), which leads to the uncontrolled accumulation of lipid peroxides and eventually results in cell death.129 This process is also found to be iron-dependent and can be shut down by using iron chelators to scavenge the excessive iron.130 Clinical investigations have confirmed that ferroptosis is particularly relevant to pathological cell death. For instance, it has already been confirmed that ferroptosis is involved in the neuronal damaging process related to Alzheimer's Disease (AD), for which abnormal iron accumulation has been consistently observed in the brain tissue of AD patients. Iron accumulation is usually associated with enhanced ROS generation and GSH depletion, which would lead to the death of neurons. Meanwhile, several iron-chelating agents have shown promise in countering ferroptosis-induced neurotoxicity and reducing the risk of cognitive decline.131 As for the relationship between ferroptosis and cancers, recent studies revealed that ferroptosis is a tumor suppressor that removes impaired or nutrient-deprived cells,127 which is also involved in key tumor suppressor pathways as ferroptosis sensitivity could be altered by the activity of p53 and KEAP1/NRF2.132,133 Moreover, ferroptosis is involved in interferon-gamma (IFNg)-mediated tumor suppression; increasing the dietary polyunsaturated fatty acid (PUFA) or iron intake could enhance the susceptibility of tumor cells to the pro-ferroptotic activity of CD8+ -T cell-dependent immune therapy.134,135 Although the actual mechanism of ferroptosis is still largely unclear, it has already been confirmed that iron and lipid peroxide are the two major components in the ferroptotic pathway, and some regulatory strategies against the two targets have shown promise in tumor treatment in a series of preliminary studies.136,137 As already discussed above, the iron atoms enhance the oxidative stress in tumor cells, and several molecular pathways that may dynamically regulate iron metabolism in the tumor microenvironment to ensure the survival of the tumor cells have been identified. It was also observed that the iron level in malignant cells is usually higher than that in healthy cells, which may render them more susceptible to ferroptotic cell death if the intracellular iron can be activated to enhance the oxidative stress. Recent investigations have revealed that the ferroptosis process could not only be regulated using small-molecule agents that target key components in the execution pathway,138,139 but also be controlled using iron-containing nanomaterials.140,141 These nanoagents are anticipated to deliver exogenous iron into the tumor intracellular environment to induce an iron overload and initiate ferroptosis. Although using iron-based nanoformulations for ferroptosis therapy is still a relatively new strategy, it has already demonstrated several major therapeutic benefits, including low treatment resistance, minimal systemic toxicity and tumor-specific toxic effects. In this section, we will provide a concise yet comprehensive review of the design, synthesis and antitumor mechanisms of some recently reported iron-based ferroptosis-inducing nanoplatforms, which may facilitate the future development of more effective ferroptosis therapies.
6.2 Ferroptosis-dependent tumor therapy via iron-based nanozymes
A typical ferroptosis-inducing strategy and its benefits were excellently demonstrated in a recent report by Shen et al., in which the authors synthesized Fenton reaction-activatable Fe3O4/Gd2O3 hybrid nanoparticles and loaded them with cisplatin for the ferroptosis treatment of orthotopic brain tumors.116 Due to their small size (∼6.6 nm) and the lactoferrin conjugates, the composite nanoparticles could readily cross the blood–brain-barrier and were taken up by the tumor cells, which were then degraded in the tumor lysosomes, releasing both the cisplatin drug and the iron ions. The increase in the concentration of iron ions in the tumor intracellular environment eventually led to the accumulation of reactive oxygen species and initiated the ferroptosis process, which was confirmed both in vitro and in vivo. Moreover, the authors also demonstrated that the efficacy of ferroptosis therapy could be improved by the cisplatin-enhanced Fenton-reaction of the released iron ions. Alternatively, the study by Zhang et al. also demonstrated that Fe3O4 nanoclusters coated with pre-engineered leukocyte membranes could induce ferroptosis in tumor cells via the iron-catalyzed generation and accumulation of cytotoxic ROS. Moreover, this biomimetic magnetosome was also loaded with TGF-β inhibitor (Ti) and PD-1 antibody (Pa) to modulate the tumor immune environment, which could concurrently enhance H2O2 production in polarized M1 macrophages and facilitate the initiation and propagation of ROS damage, leading to synergistic ferroptosis-mediated immunotherapy.142 Zheng et al. reported an alternative strategy to induce ferroptosis in tumor cells by using a single-iron-atom-chelated MOF instead of iron/iron oxide nanostructures, which was also loaded with a p53 plasmid for a therapy-enhancing “bystander” effect (Fig. 9).143 After cellular uptake, the MOF supported iron atoms could catalyze the production of cytotoxic ROS via the Fenton reaction, and the p53 plasmid could inhibit the SLC7A11 gene in the tumor cells to deplete GSH, which inhibited the elimination of lipid peroxides and enhanced the efficacy of the ferroptosis-based therapy.
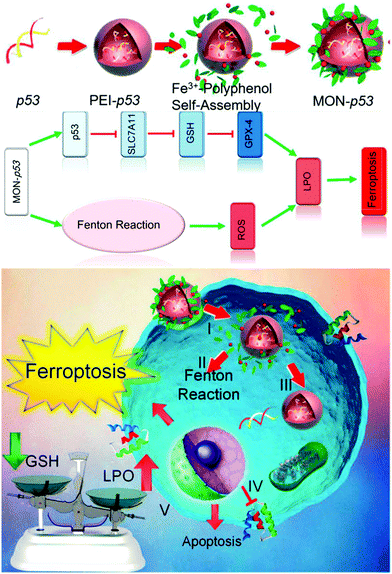 |
| Fig. 9 The synthesis procedure of the iron-chelated MOF and its molecular mechanism in inducing ferroptosis in the tumor cells. Reproduced with permission from ref. 143. Copyright © 2017, American Chemical Society. | |
7. Theranostic exploitation of iron-based nanozymes
Previous investigations have already demonstrated that iron-based nanostructures usually possess intrinsic MRI capability for tumor diagnosis, and the recent research inputs on iron-based nanozymes have revealed more exciting opportunities to further expand the range of applicable imaging modalities. Typically, the nanozyme-mediated catalase-like H2O2-to-O2 conversion is a gas-generating process, and the resultant oxygen bubbles can be exploited to enhance the sensitivity of ultrasound imaging. In a recent report by Li et al., the authors constructed Fe3O4/PLGA polymersomes by embedding Fe3O4 nanoparticles into the hydrophobic layers, while H2O2 and trisodium diphosphate (stabilizing agent) were loaded into the central liquid drop.144 The self-assembled polymersome structure could be disrupted with ultrasound at the diagnostic level, and the released H2O2 was decomposed into water and oxygen due to the biocatalytic effect of Fe3O4 nanoparticles, which facilitated the production of hydroxyl radicals. Meanwhile, the generation of oxygen could also improve the imaging sensitivity of the ultrasound.145 An alternative theranostic strategy based on iron-containing nanozymes was reported by Liu et al., in which 2,2′-azino-bis(3-ethylbenzothiazoline-6-sulfonic acid) (ABTS) was into an iron-chelated MOF that could be activated in the tumor-microenvironment for photoacoustic imaging (PAI)/photothermal therapy/chemodynamic therapy (Fig. 10). Taking advantage of the peroxidase-like biocatalytic activity of the iron-chelated MOF, the authors reported that the MOF-based nanozyme could catalytically oxidize ABTS into oxidized ABTS in the H2O2-rich tumor microenvironment to specifically switch on its PAI capability, while the hydroxyl radicals could, at the same time, destroy the surrounding tumor cells.146 These studies collectively demonstrate the application potential of iron-based nanozymes in tumor theranostics, which may afford new opportunities to facilitate their clinical translation.
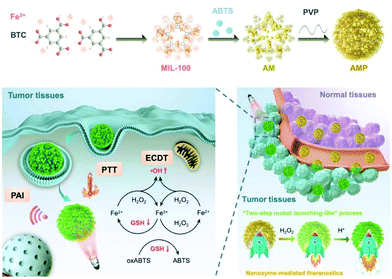 |
| Fig. 10 Construction of the ABTS-loaded iron-chelated MOF, and the tumor-responsive nanozyme-enabled theranostics. Reproduced with permission from ref. 146. © 2019 WILEY-VCH Verlag GmbH & Co. KGaA, Weinheim. | |
8. Outlook on the future development of iron-based nanozymes as therapeutic biocatalysts
As demonstrated by the discussions above, iron-based nanozymes can be used to catalyze a variety of biochemical reactions that are highly relevant in cancer treatment, which offer direct or indirect enhancement in the eventual efficacy. Nevertheless, despite the significant achievements in this field, the whole concept of using nanoscale enzyme-mimetics to biochemically interfere with the ability of tumor cells to adapt and survive is still far from mature, and there are still some challenges that remain to be addressed before these biocatalytic agents can be used on patients.
Although a growing body of evidence from both experimental and clinical studies has attested the relative safety of iron-based nanostructures for biomedical applications in vivo, it should be noted that most of the present studies are focusing on their contrasting capability for MR imaging, while their performance and safety issues as direct therapeutic agents or drug delivery carriers remain insufficiently investigated. Due to the unique interaction patterns with biomolecules and the surrounding cellular/tissue environment, as well as the biocatalysis-enabled cell-damaging mechanisms, the potential biological impact of iron-based nanozymes is distinctively different from other iron-containing nanoformulations and should be studied on a case-by-case basis.147,148 For instance, the premature leakage of biocatalytic iron ions could lead to undesired off-target toxic effects in normal cells and tissues and cause severe collateral damage. Meanwhile, it has been theorized that iron ions could catalyze a variety of biochemical reactions besides the catalase- and peroxidase-like activities discussed above, which may induce unexpected biological effects in the complex in vivo environment. To overcome these limitations, it is important to optimize the delivery process of the nanozymes and concentrate the catalytic species in tumor tissues using targeting ligands and stimuli-responsive mechanisms, as well as to improve their reaction selectivity to yield the desired product and exclude unpredictable consequences. Several nanotechnology-enabled strategies have recently been reported to realize such goals. Typically, Hu et al. reported a NIR-assisted Fe-dependent Fenton nanocatalyst for tumor-targeted therapy, which was composed of an upconverting nanoparticle (UCNP) core with mesoporous silica shell loaded with Fe2+.149 The silica shells were further modified with a Ru2+-complex that binds specifically to the mitochondrial DNA in tumor cells. According to their report, conventional Fenton reaction systems are favored at pH around 3–4, and their reaction rates would drop significantly in the tumor microenvironment (pH ∼ 6.5). However, the ˙OH generation rate by iron-based catalysts increased significantly when UV-vis light was added for external actuation. Consequently, the Fenton-catalytic activity of the Fe2+ ions in this biocatalytic nanosystem was suppressed due to insufficient H2O2 supply and neutral pH under normal conditions. After successfully reaching the H2O2-rich mitochondrial compartment of the tumor cells, the UCNP core could convert the incoming NIR light into ultraviolet rays to activate the Fe2+ ions and initiate the photo-assisted Fenton reaction, consequently limiting the ROS damage to the NIR-treated region. It is highly anticipated that such fabrication and modification technologies enhance both the accuracy and safety of iron-based nanozyme-mediated tumor therapy.
Another major concern in the therapeutic implementation of iron-based nanozymes is the balance between the sustainability of the treatment and the degradability of the nanosystem in the biological environment. Taking the Fenton reaction as an example, although the iron catalyst could be recovered through the redox cycle, it may still suffer from degradation and dissipation in biological conditions, leading to diminished catalytic efficacy under prolonged treatment. Nevertheless, the nanozymes should be degradable or clearable to minimize their long-term nano-cytotoxicity to comply with the current FDA regulations on nanoscale contrast agents.150 Solving these dilemmas would require new technological innovations in the design, synthesis and modification strategies of nanozymes, as well as meticulous investigations on their efficacy and safety in the clinical environment.
9. Conclusions
In conclusion, the potential antitumor effects of iron-based nanozymes provide ample opportunities for the development of novel tumor therapies and are expected to contribute to continuous improvement in treatment efficacy against a variety of tumor conditions. Taking advantage of their intrinsic enzyme-mimetic biocatalytic activities, these emerging nanoformulations have been used for reoxygenating the hypoxic tumor microenvironment to enhance the efficacy of other therapeutic modalities, such as photodynamic therapy, photothermal therapy, chemotherapy and radiotherapy, or to exert direct cytotoxic damage on tumor cells by readily initiating apoptosis or ferroptosis. Nevertheless, several challenges still exist for the clinical translation of iron-based nanozymes, including in vivo safety, biocatalytic selectivity/efficiency and catalyst stability, which would require the coordinated effort of both material scientists and biomedical researchers.
Abbreviations
IN | Iron-based nanomaterial |
EPR effect | Enhanced permeability and retention effect |
ROS | Reactive oxygen species |
PB | Prussian blue |
PBN | Prussian blue nanoparticle |
PBMN | Prussian blue/manganese dioxide hybrid nanoparticle |
PBNC | Prussian blue nanocubes |
GOD | Glucose oxidase |
MSN | Mesoporous silica nanoparticle |
ZnPc | Zinc phthalocyanine |
PDT | Photodynamic therapy |
Ce6 | Chlorin e6 |
MOF | Metal–organic framework |
TCPP | Tetrakis(4-carboxyphenyl)porphyrin |
GSH | Glutathione |
SPION | Superparamagnetic iron oxide nanoparticle |
β-lap | β-Lapachone |
AIO NP | Amorphous iron oxide nanoparticle |
NIR | Near-infrared light |
SOD | Superoxide dismutase |
GA | Gallic acid |
BPDB | 1,5-Bis[(L-proline-1-yl) diazen-1-ium-1,2-diol-O2-yl]-2,4-dinitrobenzene |
AD | Alzheimer's disease |
PUFA | Polyunsaturated fatty acid |
ABTS | 2,2′-Azino-bis(3-ethylbenzothiazoline-6-sulfonic acid) |
PAI | Photoacoustic imaging |
UCNP | Upconverting nanoparticle |
Conflicts of interest
There are no conflicts to declare.
Acknowledgements
This work was financially supported by National Key Technology R&D Program of China (2017YFB0702603, 2016YFC1100300), Natural Science Foundation of China (51602034, 51603024, 11832008 and 51773023), Fundamental Research Funds for the Central Universities (2018CDQYSM0036, and 2019CDYGYB004), People's Livelihood Special Innovation Projects of Chongqing CSTC (cstc2017shmsA1089) and Chongqing Research Program of Basic Research and Frontier Technology (Grant No. cstc2018jcyjAX0580). This research is also supported by the Singapore Agency for Science, Technology and Research (A*STAR) AME IRG grant (A1883c0005), and the Singapore National Research Foundation Investigatorship (NRF-NRFI2018-03).
Notes and references
- J. A. Harrigan, X. Jacq, N. M. Martin and S. P. Jackson, Nat. Rev. Drug Discovery, 2018, 17, 57–77 CrossRef CAS PubMed.
- I. Piazza, K. Kochanowski, V. Cappelletti, T. Fuhrer, E. Noor, U. Sauer and P. Picotti, Cell, 2018, 172, 358–372 CrossRef CAS PubMed.
- K. L. Dunbar, D. H. Scharf, A. Litomska and C. Hertweck, Chem. Rev., 2017, 117, 5521–5577 CrossRef CAS PubMed.
- P. Mews, G. Donahue, A. M. Drake, V. Luczak, T. Abel and S. L. Berger, Nature, 2017, 546, 381–386 CrossRef CAS PubMed.
- J. M. Choi, S. S. Han and H. S. Kim, Biotechnol. Adv., 2015, 33, 1443–1454 CrossRef CAS.
- A. Kuchler, M. Yoshimoto, S. Luginbuhl, F. Mavelli and P. Walde, Nat. Nanotechnol., 2016, 11, 409–420 CrossRef CAS.
- H. M. Kang, S. H. Ahn, P. Choi, Y. A. Ko, S. H. Han, F. Chinga, A. S. D. Park, J. L. Tao, K. Sharma, J. Pullman, E. P. Bottinger, I. J. Goldberg and K. Susztak, Nat. Med., 2015, 21, 37–46 CrossRef CAS.
- W. X. Zong, J. D. Rabinowitz and E. White, Mol. Cell, 2016, 61, 667–676 CrossRef CAS.
- P. L. Sulkowski, C. D. Corso, N. D. Robinson, S. E. Scanlon, K. R. Purshouse, H. W. Bai, Y. F. Liu, R. K. Sundaram, D. C. Hegan, N. R. Fons, G. A. Breuer, Y. B. Song, K. Mishra-Gorur, H. M. De Feyter, R. A. de Graaf, Y. V. Surovtseva, M. Kachman, S. Halene, M. Gunel, P. M. Glazer and R. S. Bindra, Sci. Transl. Med., 2017, 9, 15 Search PubMed.
- S. Lovric, S. Goncalves, H. Y. Gee, B. Oskouian, H. Srinivas, W. I. Choi, S. Shril, S. Ashraf, W. Z. Tan, J. Rao, M. Airik, D. Schapiro, D. A. Braun, C. E. Sadowski, E. Widmeier, T. Jobst-Schwan, J. M. Schmidt, V. Girik, G. Capitani, J. H. Suh, N. Lachaussee, C. Arrondel, J. Patat, O. Gribouval, M. Furlano, O. Boyer, A. Schmitt, V. Vuiblet, S. Hashmi, R. Wilcken, F. P. Bernier, A. M. Innes, J. S. Parboosingh, R. E. Lamont, J. P. Midgley, N. Wright, J. Majewski, M. Zenker, F. Schaefer, N. Kuss, J. Greil, T. Giese, K. Schwarz, V. Catheline, D. Schanze, I. Franke, Y. Sznajer, A. S. Truant, B. Adams, J. Desir, R. Biemann, Y. Pei, E. Ars, N. Lloberas, A. Madrid, V. R. Dharnidharka, A. M. Connolly, M. C. Willing, M. A. Cooper, R. P. Lifton, M. Simons, H. Riezman, C. Antignac, J. D. Saba and F. Hildebrandt, J. Clin. Invest., 2017, 127, 912–928 CrossRef.
- M. F. Huo, L. Y. Wang, Y. Chen and J. L. Shi, Nat. Commun., 2017, 8, 357 CrossRef.
- B. Ogretmen, Nat. Rev. Cancer, 2018, 18, 33–50 CrossRef CAS PubMed.
- T. Y. Wang, J. F. Fahrmann, H. Lee, Y. J. Li, S. C. Tripathi, C. Y. Yue, C. Y. Zhang, V. Lifshitz, J. Song, Y. Yuan, G. Somlo, R. Jandial, D. Ann, S. Hanash, R. Jove and H. Yu, Cell Metab., 2018, 27, 136–150 CrossRef CAS PubMed.
- Z. Dong, Z. Yang, Y. Hao and L. Feng, Nanoscale, 2019, 11, 16164–16186 RSC.
- J. Bi, T.-A. Ichu, C. Zanca, H. Yang, W. Zhang, Y. Gu, S. Chowdhry, A. Reed, S. Ikegami, K. M. Turner, W. Zhang, G. R. Villa, S. Wu, O. Quehenberger, W. H. Yong, H. I. Kornblum, J. N. Rich, T. F. Cloughesy, W. K. Cavenee, F. B. Furnari, B. F. Cravatt and P. S. Mischel, Cell Metab., 2019, 30(3), 525–538 CrossRef CAS.
- P. A. Janne, J. C. H. Yang, D. W. Kim, D. Planchard, Y. Ohe, S. S. Ramalingam, M. J. Ahn, S. W. Kim, W. C. Su, L. Horn, D. Haggstrom, E. Felip, J. H. Kim, P. Frewer, M. Cantarini, K. H. Brown, P. A. Dickinson, S. Ghiorghiu and M. Ranson, N. Engl. J. Med., 2015, 372, 1689–1699 CrossRef PubMed.
- C. M. Doskey, V. Buranasudja, B. A. Wagner, J. G. Wilkes, J. Du, J. J. Cullen and G. R. Buettner, Redox Biol., 2016, 10, 274–284 CrossRef CAS.
- C. Glorieux and P. B. Calderon, Biol. Chem., 2017, 398, 1095–1108 CAS.
- W. C. Stafford, X. X. Peng, M. H. Olofsson, X. N. Zhang, D. K. Luci, L. Lu, Q. Cheng, L. Tresaugues, T. S. Dexheimer, N. P. Coussens, M. Augsten, H. S. M. Ahlzen, O. Orwar, A. Ostman, S. Stone-Elander, D. J. Maloney, A. Jadhav, A. Simeonov, S. Linder and E. S. J. Arner, Sci. Transl. Med., 2018, 10, 13 Search PubMed.
- T. Otto and P. Sicinski, Nat. Rev. Cancer, 2017, 17, 93–115 CrossRef CAS PubMed.
- P. A. Jones, J. P. J. Issa and S. Baylin, Nat. Rev. Genet., 2016, 17, 630–641 CrossRef CAS.
- A. Ribas and J. D. Wolchok, Science, 2018, 359, 1350–1355 CrossRef CAS.
- Z. Y. Zhang, Acc. Chem. Res., 2017, 50, 122–129 CrossRef CAS PubMed.
- J. C. Li, J. G. Huang, Y. Lyu, J. S. Huang, Y. Y. Jiang, C. Xie and K. Y. Pu, J. Am. Chem. Soc., 2019, 141, 4073–4079 CrossRef CAS.
- H. Wei and E. Wang, Chem. Soc. Rev., 2013, 42, 6060–6093 RSC.
- H. Tokuyama, S. Yamago, E. Nakamura, T. Shiraki and Y. Sugiura, J. Am. Chem. Soc., 1993, 115, 7918–7919 CrossRef CAS.
- F. Manea, F. B. Houillon, L. Pasquato and P. Scrimin, Angew. Chem., Int. Ed., 2004, 43, 6165–6169 CrossRef CAS.
- L. Gao, J. Zhuang, L. Nie, J. Zhang, Y. Zhang, N. Gu, T. Wang, J. Feng, D. Yang, S. Perrett and X. Yan, Nat. Nanotechnol., 2007, 2, 577–583 CrossRef CAS.
- D. Avnir, Adv. Mater., 2018, 30, 1706804 CrossRef PubMed.
- R. Walther, A. K. Winther, A. S. Fruergaard, W. van den Akker, L. Sorensen, S. M. Nielsen, M. T. J. Olesen, Y. T. Dai, H. S. Jeppesen, P. Lamagni, A. Savateev, S. E. L. Pedersen, C. K. Frich, C. Vigier-Carriere, N. Lock, M. Singh, V. Bansal, R. L. Meyer and A. N. Zelikin, Angew. Chem., Int. Ed., 2019, 58, 278–282 CrossRef CAS.
- S. Deng, A. L. Fu, M. Junaid, Y. Wang, Q. Yin, C. Fu, L. Liu, D. S. Su, W. P. Bian and D. S. Pei, Biomaterials, 2019, 206, 61–72 CrossRef CAS PubMed.
- Y. Song, K. Qu, C. Zhao, J. Ren and X. Qu, Adv. Mater., 2010, 22, 2206–2210 CrossRef CAS PubMed.
- K. Ulbrich, K. Hola, V. Subr, A. Bakandritsos, J. Tucek and R. Zboril, Chem. Rev., 2016, 116, 5338–5431 CrossRef CAS PubMed.
- D. L. Ni, W. B. Bu, E. B. Ehlerding, W. B. Cai and J. L. Shi, Chem. Soc. Rev., 2017, 46, 7438–7468 RSC.
- S. M. Dadfar, K. Roemhild, N. I. Drude, S. von Stillfried, R. Knuchel, F. Kiessling and T. Lammers, Adv. Drug Delivery Rev., 2019, 138, 302–325 CrossRef CAS.
- M. Arruebo, R. Fernández-Pacheco, M. R. Ibarra and J. Santamaría, Nano Today, 2007, 2, 22–32 CrossRef.
- E. B. Ehlerding, P. Grodzinski, W. Cai and C. H. Liu, ACS Nano, 2018, 12, 2106–2121 CrossRef CAS PubMed.
- G. De Crozals, R. Bonnet, C. Farre and C. Chaix, Nano Today, 2016, 11, 435–463 CrossRef CAS.
- K. Zhu, Y. M. Ju, J. J. Xu, Z. Y. Yang, S. Gao and Y. L. Hou, Acc. Chem. Res., 2018, 51, 404–413 CrossRef CAS PubMed.
- T. Kang, F. Li, S. Baik, W. Shao, D. Ling and T. Hyeon, Biomaterials, 2017, 136, 98–114 CrossRef CAS PubMed.
- W. J. Stark, P. R. Stoessel, W. Wohlleben and A. Hafner, Chem. Soc. Rev., 2015, 44, 5793–5805 RSC.
- L. Hajba and A. Guttman, Biotechnol. Adv., 2016, 34, 354–361 CrossRef CAS PubMed.
- K. Li, H. Nejadnik and H. E. Daldrup-Link, Drug Discovery Today, 2017, 22, 1421–1429 CrossRef CAS PubMed.
- H. Huang and J. F. Lovell, Adv. Funct. Mater., 2017, 27, 1603524 CrossRef.
- D. Kim, K. Shin, S. G. Kwon and T. Hyeon, Adv. Mater., 2018, 30, 1802309 CrossRef.
- C. Song, W. Sun, Y. Xiao and X. Shi, Drug Discovery Today, 2019, 24, 835–844 CrossRef CAS.
- S. M. Dadfar, K. Roemhild, N. I. Drude, S. von Stillfried, R. Knüchel, F. Kiessling and T. Lammers, Adv. Drug Delivery Rev., 2019, 138, 302–325 CrossRef CAS.
- Z. Chen, J.-J. Yin, Y.-T. Zhou, Y. Zhang, L. Song, M. Song, S. Hu and N. Gu, ACS Nano, 2012, 6, 4001–4012 CrossRef CAS PubMed.
- Q. Wang, Z. Yang, X. Zhang, X. Xiao, C. K. Chang and B. Xu, Angew. Chem., Int. Ed., 2007, 46, 4285–4289 CrossRef CAS.
- H. Wang, K. Wan and X. Shi, Adv. Mater., 2018, 1805368 Search PubMed.
- B. Jiang, D. Duan, L. Gao, M. Zhou, K. Fan, Y. Tang, J. Xi, Y. Bi, Z. Tong, G. F. Gao, N. Xie, A. Tang, G. Nie, M. Liang and X. Yan, Nat. Protoc., 2018, 13, 1506–1520 CrossRef CAS PubMed.
- L. Gao, K. Fan and X. Yan, Theranostics, 2017, 7, 3207–3227 CrossRef CAS PubMed.
- X. Li and J. Paier, J. Phys. Chem. C, 2016, 120, 1056–1065 CrossRef CAS.
- J. He, X. Yang, B. Men and D. Wang, J. Environ. Sci., 2016, 39, 97–109 CrossRef.
- S. Liu, F. Lu, R. Xing and J.-J. Zhu, Chem. – Eur. J., 2011, 17, 620–625 CrossRef CAS.
- H. Wang, H. Jiang, S. Wang, W. Shi, J. He, H. Liu and Y. Huang, RSC Adv., 2014, 4, 45809–45815 RSC.
- P. J. Burke, Trends, Cancer, 2017, 3, 857–870 CAS.
- Q. Cui, J. Q. Wang, Y. G. Assaraf, L. Ren, P. Gupta, L. Y. Wei, C. R. Ashby, D. H. Yang and Z. S. Chen, Drug Resist. Updates, 2018, 41, 1–25 CrossRef PubMed.
- M. D. Buck, R. T. Sowell, S. M. Kaech and E. L. Pearce, Cell, 2017, 169, 570–586 CrossRef CAS PubMed.
- S. Galadari, A. Rahman, S. Pallichankandy and F. Thayyullathil, Free Radical Biol. Med., 2017, 104, 144–164 CrossRef CAS.
- J. N. Liu, W. B. Bu and J. L. Shi, Chem. Rev., 2017, 117, 6160–6224 CrossRef CAS PubMed.
- Y. L. Dai, C. Xu, X. L. Sun and X. Y. Chen, Chem. Soc. Rev., 2017, 46, 3830–3852 RSC.
- I. Milisav, B. Poljsak and S. Ribaric, Apoptosis, 2017, 22, 265–283 CrossRef CAS PubMed.
- B. Muz, P. de la Puente, F. Azab and A. K. Azab, Hypoxia, 2015, 3, 83–92 CrossRef PubMed.
- W. P. Fan, B. Yung, P. Huang and X. Y. Chen, Chem. Rev., 2017, 117, 13566–13638 CrossRef CAS PubMed.
- G. S. Song, L. Cheng, Y. Chao, K. Yang and Z. Liu, Adv. Mater., 2017, 29, 26 Search PubMed.
- T. P. Szatrowski and C. F. Nathan, Cancer Res., 1991, 51, 794–798 CAS.
- E. Panieri and M. M. Santoro, Cell Death Dis., 2016, 7, e2253 CrossRef CAS PubMed.
- Q. Chen, C. Liang, X. Sun, J. Chen, Z. Yang, H. Zhao, L. Feng and Z. Liu, Proc. Natl. Acad. Sci. U. S. A., 2017, 114, 5343–5348 CrossRef CAS PubMed.
- C. Lennicke, J. Rahn, R. Lichtenfels, L. A. Wessjohann and B. Seliger, Cell Commun. Signaling, 2015, 13, 39 CrossRef PubMed.
- S. Li, L. Shang, B. Xu, S. Wang, K. Gu, Q. Wu, Y. Sun, Q. Zhang, H. Yang, F. Zhang, L. Gu, T. Zhang and H. Liu, Angew. Chem., Int. Ed., 2019, 58, 12624–12631 CrossRef CAS PubMed.
- F. Yang, M. Li, H. Cui, T. Wang, Z. Chen, L. Song, Z. Gu, Y. Zhang and N. Gu, Sci. China Mater., 2015, 58, 467–480 CrossRef CAS.
- K. Fan, H. Wang, J. Xi, Q. Liu, X. Meng, D. Duan, L. Gao and X. Yan, Chem. Commun., 2017, 53, 424–427 RSC.
- J. J. X. Wu, X. Y. Wang, Q. Wang, Z. P. Lou, S. R. Li, Y. Y. Zhu, L. Qin and H. Wei, Chem. Soc. Rev., 2019, 48, 1004–1076 RSC.
- J. X. Chen, Q. Q. Wang, L. Huang, H. Zhang, K. Rong, H. Zhang and S. J. Dong, Nano Res., 2018, 11, 4905–4913 CrossRef CAS.
- M. A. Komkova, E. E. Karyakina and A. A. Karyakin, J. Am. Chem. Soc., 2018, 140, 11302–11307 CrossRef CAS.
- W. Zhang, S. Hu, J.-J. Yin, W. He, W. Lu, M. Ma, N. Gu and Y. Zhang, J. Am. Chem. Soc., 2016, 138, 5860–5865 CrossRef CAS.
- J. Peng, Q. Yang, W. Li, L. Tan, Y. Xiao, L. Chen, Y. Hao and Z. Qian, ACS Appl. Mater. Interfaces, 2017, 9, 44410–44422 CrossRef CAS.
- Y. X. Zhu, H. R. Jia, G. Y. Pan, N. W. Ulrich, Z. Chen and F. G. Wu, J. Am. Chem. Soc., 2018, 140, 4062–4070 CrossRef CAS.
- W. Tian, Y. Y. Su, Y. Tian, S. J. Wang, X. D. Su, Y. Liu, Y. L. Zhang, Y. X. Tang, Q. Q. Ni, W. F. Liu, M. Dang, C. Y. Wang, J. J. Zhang, Z. G. Teng and G. M. Lu, Adv. Sci., 2017, 4, 10 Search PubMed.
- Y. J. Liu, G. M. Shu, X. Li, H. B. Chen, B. Zhang, H. Z. Pan, T. Li, X. Q. Gong, H. J. Wang, X. L. Wu, Y. Dou and J. Chang, Adv. Funct. Mater., 2018, 28, 12 Search PubMed.
- B. Zhou, B.-P. Jiang, W. Sun, F.-M. Wei, Y. He, H. Liang and X.-C. Shen, ACS Appl. Mater. Interfaces, 2018, 10, 18036–18049 CrossRef CAS PubMed.
- J. Florek, R. Caillard and F. Kleitz, Nanoscale, 2017, 9, 15252–15277 RSC.
- J. Wen, K. Yang, F. Y. Liu, H. J. Li, Y. Q. Xu and S. G. Sun, Chem. Soc. Rev., 2017, 46, 6024–6045 RSC.
- D. Wang, R. Shi, J. Zhou, S. Shi, H. Wu, P. Xu, H. Wang, G. Xia, T. E. Barnhart, W. Cai, Z. Guo and Q. Chen, iScience, 2018, 9, 14–26 CrossRef CAS.
- Z. L. Yang, W. Tian, Q. Wang, Y. Zhao, Y. L. Zhang, Y. Tian, Y. X. Tang, S. J. Wang, Y. Liu, Q. Q. Ni, G. M. Lu, Z. G. Teng and L. J. Zhang, Adv. Sci., 2018, 5, 1700847 CrossRef.
- S.-Y. Yin, G. Song, Y. Yang, Y. Zhao, P. Wang, L.-M. Zhu, X. Yin and X.-B. Zhang, Adv. Funct. Mater., 2019, 29, 1901417 CrossRef.
- Y. Yang, W. Zhu, L. Feng, Y. Chao, X. Yi, Z. Dong, K. Yang, W. Tan, Z. Liu and M. Chen, Nano Lett., 2018, 18, 6867–6875 CrossRef CAS.
- Z. Zhang, J. Yang, Q. Min, C. Ling, D. Maiti, J. Xu, L. Qin and K. Yang, Small, 2019, 15, e1803703 CrossRef.
- C. Gorrini, I. S. Harris and T. W. Mak, Nat. Rev. Drug Discovery, 2013, 12, 931–947 CrossRef CAS.
- I. I. C. Chio and D. A. Tuveson, Trends Mol. Med., 2017, 23, 411–429 CrossRef CAS.
- J. F. Quinn, M. R. Whittaker and T. P. Davis, Polym. Chem., 2017, 8, 97–126 RSC.
- S. L. Cramer, A. Saha, J. Y. Liu, S. Tadi, S. Tiziani, W. P. Yan, K. Triplett, C. Lamb, S. E. Alters, S. Rowlinson, Y. J. Zhang, M. J. Keating, P. Huang, J. DiGiovanni, G. Georgiou and E. Stone, Nat. Med., 2017, 23, 120–127 CrossRef CAS.
- M. Cheng, C. Lai, Y. Liu, G. M. Zeng, D. L. Huang, C. Zhang, L. Qin, L. Hu, C. Y. Zhou and W. P. Xiong, Coord. Chem. Rev., 2018, 368, 80–92 CrossRef CAS.
- Y. N. Yang, J. Tang, P. L. Abbaraju, M. Jambhrunkar, H. Song, M. Zhang, C. Lei, J. Y. Fu, Z. Y. Gu, Y. Liu and C. Z. Yu, Angew. Chem., Int. Ed., 2018, 57, 11764–11769 CrossRef CAS.
- L. S. Lin, J. B. Song, L. Song, K. M. Ke, Y. J. Liu, Z. J. Zhou, Z. Y. Shen, J. Li, Z. Yang, W. Tang, G. Niu, H. H. Yang and X. Y. Chen, Angew. Chem., Int. Ed., 2018, 57, 4902–4906 CrossRef CAS.
- K. W. Chang, Z. H. Liu, X. F. Fang, H. B. Chen, X. J. Men, Y. Yuan, K. Sun, X. J. Zhang, Z. Yuan and C. F. Wu, Nano Lett., 2017, 17, 4323–4329 CrossRef CAS.
- X. Qian, J. Zhang, Z. Gu and Y. Chen, Biomaterials, 2019, 211, 1–13 CrossRef CAS.
- B. H. Kim, N. Lee, H. Kim, K. An, Y. I. Park, Y. Choi, K. Shin, Y. Lee, S. G. Kwon, H. B. Na, J.-G. Park, T.-Y. Ahn, Y.-W. Kim, W. K. Moon, S. H. Choi and T. Hyeon, J. Am. Chem. Soc., 2011, 133, 12624–12631 CrossRef CAS.
- V. F. Cardoso, A. Francesko, C. Ribeiro, M. Banobre-Lopez, P. Martins and S. Lanceros-Mendez, Adv. Healthcare Mater., 2018, 7, 1700845 CrossRef.
- G. Huang, H. Chen, Y. Dong, X. Luo, H. Yu, Z. Moore, E. A. Bey, D. A. Boothman and J. Gao, Theranostics, 2013, 3, 116–126 CrossRef CAS.
- P. a. Ma, H. Xiao, C. Yu, J. Liu, Z. Cheng, H. Song, X. Zhang, C. Li, J. Wang, Z. Gu and J. Lin, Nano Lett., 2017, 17, 928–937 CrossRef CAS.
- A. K. Hauser, M. I. Mitov, E. F. Daley, R. C. McGarry, K. W. Anderson and J. Z. Hilt, Biomaterials, 2016, 105, 127–135 CrossRef CAS.
- Y. Liu, X. Ji, W. W. L. Tong, D. Askhatova, T. Yang, H. Cheng, Y. Wang and J. Shi, Angew. Chem., Int. Ed., 2018, 57, 1510–1513 CrossRef CAS.
- Z. Tang, H. Zhang, Y. Liu, D. Ni, H. Zhang, J. Zhang, Z. Yao, M. He, J. Shi and W. Bu, Adv. Mater., 2017, 29, 1701683 CrossRef.
- C. Zhang, W. Bu, D. Ni, S. Zhang, Q. Li, Z. Yao, J. Zhang, H. Yao, Z. Wang and J. Shi, Angew. Chem., Int. Ed., 2016, 55, 2101–2106 CrossRef CAS.
- Z. Tang, Y. Liu, M. He and W. Bu, Angew. Chem., Int. Ed., 2019, 58, 946–956 CrossRef CAS.
- W. Feng, X. Han, R. Wang, X. Gao, P. Hu, W. Yue, Y. Chen and J. Shi, Adv. Mater., 2019, 31, 1805919 Search PubMed.
- W. Ke, J. Li, F. Mohammed, Y. Wang, K. Tou, X. Liu, P. Wen, H. Kinoh, Y. Anraku, H. Chen, K. Kataoka and Z. Ge, ACS Nano, 2019, 13, 2357–2369 CAS.
- B.-J. Hong, J. Kim, H. Jeong, S. Bok, Y.-E. Kim and G. O. Ahn, Radiat. Oncol. J., 2016, 34, 239–249 CrossRef.
- A. Z. Abbasi, C. R. Gordijo, M. A. Amini, A. Maeda, A. M. Rauth, R. S. DaCosta and X. Y. Wu, Cancer Res., 2016, 76, 6643–6656 CrossRef CAS PubMed.
- D. Wu, X. Duan, Q. Guan, J. Liu, X. Yang, F. Zhang, P. Huang, J. Shen, X. Shuai and Z. Cao, Adv. Funct. Mater., 2019, 29, 1900095 CrossRef.
- X. Li, Z. Ao, J. Liu, H. Sun, A. I. Rykov and J. Wang, ACS Nano, 2016, 10, 11532–11540 CrossRef CAS.
- L. Feng, R. Xie, C. Wang, S. Gai, F. He, D. Yang, P. Yang and J. Lin, ACS Nano, 2018, 12, 11000–11012 CrossRef CAS.
- J. Kim, H. R. Cho, H. Jeon, D. Kim, C. Song, N. Lee, S. H. Choi and T. Hyeon, J. Am. Chem. Soc., 2017, 139, 10992–10995 CrossRef CAS.
- Z. Shen, T. Liu, Y. Li, J. Lau, Z. Yang, W. Fan, Z. Zhou, C. Shi, C. Ke, V. I. Bregadze, S. K. Mandal, Y. Liu, Z. Li, T. Xue, G. Zhu, J. Munasinghe, G. Niu, A. Wu and X. Chen, ACS Nano, 2018, 12, 11355–11365 CrossRef CAS.
- J. Xiao, G. Zhang, R. Xu, H. Chen, H. Wang, G. Tian, B. Wang, C. Yang, G. Bai, Z. Zhang, H. Yang, K. Zhong, D. Zou and Z. Wu, Biomaterials, 2019, 216, 119254 CrossRef CAS.
- X.-F. Yang, A. Wang, B. Qiao, J. Li, J. Liu and T. Zhang, Acc. Chem. Res., 2013, 46, 1740–1748 CrossRef CAS.
- Y. Chen, S. Ji, C. Chen, Q. Peng, D. Wang and Y. Li, Joule, 2018, 2, 1242–1264 CrossRef CAS.
- S. Cao, F. Tao, Y. Tang, Y. Li and J. Yu, Chem. Soc. Rev., 2016, 45, 4747–4765 RSC.
- Z. L. Dong, L. Z. Feng, Y. Chao, Y. Hao, M. C. Chen, F. Gong, X. Han, R. Zhang, L. Cheng and Z. Liu, Nano Lett., 2019, 19, 805–815 CrossRef.
- Y. Hu, T. Lv, Y. Ma, J. Xu, Y. Zhang, Y. Hou, Z. Huang and Y. Ding, Nano Lett., 2019, 19, 2731–2738 CrossRef CAS.
- H. Ranji-Burachaloo, F. Karimi, K. Xie, Q. Fu, P. A. Gurr, D. E. Dunstan and G. G. Qiao, ACS Appl. Mater. Interfaces, 2017, 9, 33599–33608 CrossRef CAS PubMed.
- I. A. Cree and P. Charlton, BMC Cancer, 2017, 17, 8 CrossRef PubMed.
- B. Wang, J. M. Rosano, R. Cheheltani, M. P. Achary and M. F. Kiani, Expert Opin. Drug Delivery, 2010, 7, 1159–1173 CrossRef CAS.
- C. B. He, J. Q. Lu and W. B. Lin, J. Controlled Release, 2015, 219, 224–236 CrossRef CAS.
- B. R. Stockwell, J. P. F. Angeli, H. Bayir, A. I. Bush, M. Conrad, S. J. Dixon, S. Fulda, S. Gascon, S. K. Hatzios, V. E. Kagan, K. Noel, X. J. Jiang, A. Linkermann, M. E. Murphy, M. Overholtzer, A. Oyagi, G. C. Pagnussat, J. Park, Q. Ran, C. S. Rosenfeld, K. Salnikow, D. L. Tang, F. M. Torti, S. V. Torti, S. Toyokuni, K. A. Woerpel and D. D. Zhang, Cell, 2017, 171, 273–285 CrossRef CAS.
- V. S. Viswanathan, M. J. Ryan, H. D. Dhruv, S. Gill, O. M. Eichhoff, B. Seashore-Ludlow, S. D. Kaffenberger, J. K. Eaton, K. Shimada, A. J. Aguirre, S. R. Viswanathan, S. Chattopadhyay, P. Tamayo, W. S. Yang, M. G. Rees, S. X. Chen, Z. V. Boskovic, S. Javaid, C. Huang, X. Y. Wu, Y. Y. Tseng, E. M. Roider, D. Gao, J. M. Cleary, B. M. Wolpin, J. P. Mesirov, D. A. Haber, J. A. Engelman, J. S. Boehm, J. D. Kotz, C. S. Hon, Y. Chen, W. C. Hahn, M. P. Levesque, J. G. Doench, M. E. Berens, A. F. Shamji, P. A. Clemons, B. R. Stockwell and S. L. Schreiber, Nature, 2017, 547, 453–457 CrossRef CAS.
- S. J. Dixon, K. M. Lemberg, M. R. Lamprecht, R. Skouta, E. M. Zaitsev, C. E. Gleason, D. N. Patel, A. J. Bauer, A. M. Cantley, W. S. Yang, B. Morrison, 3rd and B. R. Stockwell, Cell, 2012, 149, 1060–1072 CrossRef CAS.
- W. S. Yang and B. R. Stockwell, Trends Cell Biol., 2016, 26, 165–176 CrossRef CAS.
- J. R. Wu, Q. Z. Tuo and P. Lei, J. Mol. Neurosci., 2018, 66, 197–206 CrossRef CAS.
- R. Kang, G. Kroemer and D. Tang, Free Radic. Biol. Med., 2019, 133, 162–168 CrossRef CAS.
- S. J. Dixon and B. R. Stockwell, Annu. Rev. Cancer Biol., 2019, 3, 35–54 CrossRef.
- W. Wang, M. Green, J. E. Choi, M. Gijón, P. D. Kennedy, J. K. Johnson, P. Liao, X. Lang, I. Kryczek, A. Sell, H. Xia, J. Zhou, G. Li, J. Li, W. Li, S. Wei, L. Vatan, H. Zhang, W. Szeliga, W. Gu, R. Liu, T. S. Lawrence, C. Lamb, Y. Tanno, M. Cieslik, E. Stone, G. Georgiou, T. A. Chan, A. Chinnaiyan and W. Zou, Nature, 2019, 569, 270–274 CrossRef CAS.
- B. R. Stockwell and X. Jiang, Cell Metab., 2019, 30, 14–15 CrossRef CAS.
- A. R. Bogdan, M. Miyazawa, K. Hashimoto and Y. Tsuji, Trends Biochem. Sci., 2016, 41, 274–286 CrossRef CAS PubMed.
- S. J. Dixon and B. R. Stockwell, Nat. Chem. Biol., 2013, 10, 9 CrossRef PubMed.
- Y. Xie, W. Hou, X. Song, Y. Yu, J. Huang, X. Sun, R. Kang and D. Tang, Cell Death Differ., 2016, 23, 369 CrossRef CAS.
- M. Gao, P. Monian, N. Quadri, R. Ramasamy and X. Jiang, Mol. Cell, 2015, 59, 298–308 CrossRef CAS PubMed.
- Z. Y. Shen, J. B. Song, B. C. Yung, Z. J. Zhou, A. G. Wu and X. Y. Chen, Adv. Mater., 2018, 30, 15 Search PubMed.
- M. Liu, B. Liu, Q. Liu, K. Du, Z. Wang and N. He, Coord. Chem. Rev., 2019, 382, 160–180 CrossRef CAS.
- F. Zhang, F. Li, G.-H. Lu, W. Nie, L. Zhang, Y. Lv, W. Bao, X. Gao, W. Wei, K. Pu and H.-Y. Xie, ACS Nano, 2019, 13, 5662–5673 CrossRef CAS PubMed.
- D. W. Zheng, Q. Lei, J. Y. Zhu, J. X. Fan, C. X. Li, C. Li, Z. Xu, S. X. Cheng and X. Z. Zhang, Nano Lett., 2017, 17, 284–291 CrossRef CAS PubMed.
- W.-P. Li, C.-H. Su, Y.-C. Chang, Y.-J. Lin and C.-S. Yeh, ACS Nano, 2016, 10, 2017–2027 CrossRef CAS PubMed.
- D. W. Jiang, D. L. Ni, Z. T. Rosenkrans, P. Huang, X. Y. Yan and W. B. Cai, Chem. Soc. Rev., 2019, 48, 3683–3704 RSC.
- F. Liu, L. Lin, Y. Zhang, Y. Wang, S. Sheng, C. Xu, H. Tian and X. Chen, Adv. Mater., 2019, 31, 1902885 CrossRef CAS PubMed.
- H. Lin, Y. Chen and J. L. Shi, Chem. Soc. Rev., 2018, 47, 1938–1958 RSC.
- B. W. Yang, Y. Chen and J. L. Shi, Prog. Biochem. Biophys., 2018, 45, 237–255 Search PubMed.
- P. Hu, T. Wu, W. Fan, L. Chen, Y. Liu, D. Ni, W. Bu and J. Shi, Biomaterials, 2017, 141, 86–95 CrossRef CAS.
- H. Soo Choi, W. Liu, P. Misra, E. Tanaka, J. P. Zimmer, B. Itty Ipe, M. G. Bawendi and J. V. Frangioni, Nat. Biotechnol., 2007, 25, 1165 CrossRef.
Footnote |
† These authors contributed equally to this work. |
|
This journal is © The Royal Society of Chemistry 2020 |
Click here to see how this site uses Cookies. View our privacy policy here.