DOI:
10.1039/C9NR08420G
(Paper)
Nanoscale, 2020,
12, 372-379
Orthogonal deposition of Au on different facets of Ag cuboctahedra for the fabrication of nanoboxes with complementary surfaces†
Received
30th September 2019
, Accepted 21st November 2019
First published on 22nd November 2019
Abstract
We report the fabrication of Ag–Au cuboctahedral nanoboxes enclosed by {100} and {111} facets, respectively, through the orthogonal deposition of Au on two different facets of Ag cuboctahedra. Specifically, we titrate aqueous HAuCl4 into an aqueous mixture containing Ag cuboctahedra, ascorbic acid, and NaOH (under basic conditions), in the presence of poly(vinylpyrrolidone) (PVP) and cetyltrimethylammonium chloride (CTAC), respectively. In the case of PVP, the oxidation of Ag was initiated from the {111} facets of the cuboctahedra through the galvanic replacement reaction between Au(III) and Ag, accompanied by the deposition of Au onto the {100} facets. Because the dissolved Ag(I) ions could react with NaOH to form Ag2O on the {111} facets and thus terminate the galvanic reaction, the Au(III) ions would be further reduced by the ascorbate monoanion (HAsc−) to generate Au atoms for their continuing deposition on the {100} facets, converting Ag cuboctahedra to Ag@Au{100} cuboctahedra. Upon the etching of Ag from the core, we obtained Ag–Au cuboctahedral nanoboxes enclosed by {100} facets. In contrast, when CTAC was present, the oxidation of Ag through a galvanic reaction could continuously proceed on {100} facets as the dissolved Ag(I) ions would react with the excessive amount of Cl− ions derived from CTAC to produce soluble AgCl2− ions rather than insoluble Ag2O. As a result, the dissolved Ag(I) and Au(III) ions would be co-reduced by HAsc− for the generation of Ag and Au atoms, followed by their co-deposition onto {111} facets for the generation of Ag@Au{111} concave cuboctahedra. After the removal of Ag from the core by etching, we obtained Ag–Au{111} cuboctahedral nanoboxes enclosed by {111} facets. Both samples of cuboctahedral nanoboxes exhibited strong optical absorption in the infrared region. Interestingly, the cuboctahedral nanoboxes enclosed by {111} facets showed significantly enhanced catalytic activity toward the reduction of 4-nitrophenol by NaBH4 relative to their counterparts encased by {100} facets.
Introduction
The shape of noble-metal nanocrystals has long been identified as a key parameter in controlling their properties toward the development of applications in plasmonics and catalysis.1–4 In particular, it is well established that the distinctive activity and selectivity of metal nanocrystals in catalytic reactions explicitly depend on the arrangement of atoms present on the outermost layer of different facets.5–8 In the past, there has been a growing effort in the rational design and synthesis of metal nanocrystals with well-defined facets. Among these, the seed-mediated growth of metal nanocrystals has shown remarkable success in offering a fine control over the geometry of the products by directly depositing the atoms on the facets of preformed seeds.9–11 It is well known that the introduction of ligands into the growth solution could facilitate their selective chemisorption on different types of facets due to the differences in the binding affinity,12 making it possible to maneuver the growth rates on different facets and thus control the relative surface areas of different facets on the final products.13,14 For example, Xia and co-workers demonstrated the transformation of Ag nanocubes into cuboctahedra by introducing citrate in a reaction solution to bind on the {111} facets of Ag nanocubes.15 It was found that the Ag atoms would be preferentially deposited on the {100} facets to accelerate their growth along the 〈100〉 direction, leading to the evolution of a cube into a cuboctahedron. We reported the reversible transformation between Ag nanoplates and Ag twinned cubes, elucidating the role of Cl− ions in deterring the deposition and etching of Ag atoms along the 〈100〉 direction and allowing the deposition and etching along the 〈111〉 direction.16,17 Recently, Mirkin and co-workers demonstrated the generation of Au octahedra with hollow features by stabilizing the {111} facets of Au concave cubes through the underpotential deposition (UPD) of Ag+ ions,18 during which the growth of Au occurred almost exclusively on the corners of the cubic seeds. On the other hand, many groups have demonstrated the generation of bimetallic core–frame and core–shell nanocrystals by selectively depositing the metal on the small areas of edges and the entire surfaces of preformed nanocrystal seeds with the involvement of different ligands in the reaction solutions.19–25 Despite remarkable success, the previous studies involve the use of nanocrystal seeds, such as cubes, plates, and octahedra, with a single type of facet on their surfaces.
Herein we report the facet-selected, orthogonal deposition of Au on Ag cuboctahedra enclosed by a mix of {100} and {111} facets at a ratio of about 1
:
1.7 in terms of surface area. In a typical synthesis, we dispersed Ag cuboctahedra in an aqueous solution containing ascorbic acid (H2Asc), NaOH, poly(vinylpyrrolidone) (PVP) or cetyltrimethylammonium chloride (CTAC). Our success relies on the use of PVP or CTAC to instigate the Ag oxidation on the {111} or {100} facets, respectively, making it possible to control the deposition pathways for Au atoms on the {100} or {111} facets in the orthogonal manner for the generation of Ag@Au{100} cuboctahedra or Ag@Ag–Au{111} concave cuboctahedra. When Ag in the core is subjected to removal by etching, we demonstrate the transformation of solids into Ag–Au{100} or Ag–Au{111} cuboctahedral nanoboxes with complementary surfaces.
Experimental
Chemicals and materials
Ethylene glycol (EG) was purchased from J. T. Baker. Silver trifluoroacetate (CF3COOAg, 98%), gold(III) chloride trihydrate (HAuCl4·3H2O, 99.9+%), sodium hydrosulfide hydrate (NaHS·xH2O), poly(vinylpyrrolidone) (PVP) with an average molecular weight of 29
000 (PVP–29) or 55
000 (PVP–55), aqueous hydrochloric acid (HCl, 37 wt%), acetone (99.5+%), L-ascorbic acid (H2Asc, 99%), sodium citrate tribasic dihydrate (citrate, 99.0+%), silver nitrate (AgNO3, 99.0+%), aqueous cetyltrimethylammonium chloride solution (CTAC, 25 wt%), aqueous hydrogen peroxide (H2O2, 30 wt%), 4-nitrophenol (4-NP), and sodium borohydride (NaBH4, 99.99%) were purchased from Sigma-Aldrich. Sodium hydroxide (NaOH, 98%) and acetone (HPLC grade, 99.5+%) were obtained from Alfa Aesar. All chemicals were used as received. All the aqueous solutions were prepared using DI water with a resistivity of 18.2 MΩ cm at 25 °C.
Synthesis of Ag nanocubes
We followed the protocol reported by Xia and co-workers for the synthesis of Ag nanocubes with an average edge length of 38.1 ± 2.0 nm.26 The as-obtained Ag nanocubes were washed with acetone and DI water twice and then dispersed in water for further use.
Synthesis of Ag cuboctahedra
We used the as-obtained Ag nanocubes as seeds to grow Ag cuboctahedra.15 In a typical process, 34 μL of the aqueous suspension of Ag nanocubes (approximately 7.10 × 1010 particles per mL) was injected into an aqueous solution in a 23 mL glass vial containing 5 mL of PVP-55 (2 mg mL−1), 100 μL of H2Asc (0.1 M), and 50 μL of citrate (20 mM) under magnetic stirring at room temperature. Next, we used a syringe pump to titrate 1.5 mL of aqueous AgNO3 (1 mM) into the reaction solution at a rate of 5 mL h−1. Upon the completion of titration, the mixture was centrifuged at 1000 rpm for 10 min to precipitate out the larger random particles before the supernatant was centrifuged at 8000 rpm for 10 min to collect the solids. Finally, the as-prepared nanocrystals were washed with water twice before they were re-dispersed in 100 μL of water for further use. The Ag cuboctahedra had an average edge length of 47.2 ± 2.2 nm.
Synthesis of Ag@Au{100} cuboctahedra
In a typical process, 2 mL of aqueous PVP-29 (1 mM) was taken in a 23 mL glass vial, followed by the addition of 0.5 mL of aqueous H2Asc (0.1 M), 0.5 mL of aqueous NaOH (0.2 M), and 30 μL of an aqueous suspension of the Ag cuboctahedra (approximately 4.23 × 1010 particles per mL) under magnetic stirring at room temperature. Next, we introduced 0.4 mL of aqueous HAuCl4 (0.1 mM) into the reaction solution using a syringe pump at an injection rate of 1 mL min−1. After the completion of titration, the reaction solution was left undisturbed for 5 min before we collected the solid products by centrifugation at 6300 rpm for 10 min and then washed with water three times prior to characterization.
Synthesis of Ag–Au{100} cuboctahedral nanoboxes
We mixed 50 μL of the as-prepared Ag@Au{100} cuboctahedra with 50 μL of aqueous NaCl (10 mM), followed by the addition of 0.7 mL of PVP-29 (1 mM) and 0.3 mL of H2Asc (0.1 M) at room temperature. After 20 min of treatment, we collected the particles by centrifugation at 5000 rpm for 15 min, washed with water once, and then introduced into 1 mL of 3% aqueous H2O2 at room temperature. After 3 h, the hollow particles were collected by centrifugation at 13
000 rpm for 10 min, washed with water twice, and then re-dispersed in 50 μL of water for both the characterization and evaluation of catalytic properties.
Synthesis of Ag@Ag–Au{111} concave cuboctahedra
In a typical process, we took 2 mL of aqueous CTAC (0.1 M) in a 23 mL glass vial, followed by the addition of 0.5 mL of aqueous H2Asc (0.1 M), 0.5 mL of aqueous NaOH (0.2 M), and 30 μL of an aqueous suspension of the Ag cuboctahedra (approximately 4.23 × 1010 particles per mL) under magnetic stirring at room temperature. Next, we titrated 0.8 mL of aqueous HAuCl4 (0.1 mM) into the reaction solution using a syringe pump at a rate of 0.02 mL min−1. Immediately after the titration, we collected the particles by centrifugation at 4800 rpm for 11 min and washed with water once for characterization and further use.
Synthesis of Ag–Au{111} cuboctahedral nanoboxes
We dispersed 50 μL of the as-prepared Ag@Au{111} cuboctahedra in 1 mL of 3% aqueous H2O2 for etching at room temperature. After 3 h of etching, we collected the particles by centrifugation at 13
000 rpm for 10 min, washed with water twice, and then re-dispersed in 50 μL of water for the characterization and evaluation of catalytic properties.
Catalytic characterization of Ag–Au{100} and Ag–Au{111} nanoboxes
In a typical experiment, 2 mL of aqueous 4-NP (0.2 mM), 5 mL of water, and 1 mL of aqueous NaBH4 (10 mg mL−1, freshly prepared, ice cold) were added into a 23 mL glass vial under magnetic stirring. After the introduction of the nanoboxes (∼1011 particles), we monitored the reaction as a function of time by withdrawing 0.5 mL of the reaction solution to collect the UV-vis spectrum at each time point.
Instrumentation and characterization
The UV-vis spectra were collected using a Cary 50 spectrometer (Agilent Technologies, Santa Clara, CA). The UV-vis-NIR spectra were collected using a LAMDA 750 (PerkinElmer, Waltham, MA). The nanoparticles in an aqueous suspension were collected using a conventional centrifuge (Eppendorf 5430). The pH values of aqueous solutions were measured using a FE20 FiveEasy pH meter (Mettler Toledo, Columbus, OH). The quantitative measurement of Ag and Au contents in the nanocrystals was performed using an inductively coupled plasma mass spectrometer (NexION 300Q ICP-MS, PerkinElmer, Waltham, MA). Transmission electron microscopy (TEM) images were captured using a Hitachi HT7700 (Tokyo, Japan) operating at 120 kV. Scanning electron microscopy (SEM) images were captured with a Hitachi SU8230 FE-SEM (Tokyo, Japan) operating at 15–20 kV. High-angle annular dark field scanning electron microscopy and elemental mapping images were captured using a Hitachi HD2700 Cs-corrected STEM (Tokyo, Japan) operating at 200 kV.
Results and discussion
Fig. 1 illustrates the two proposed pathways responsible for the orthogonal deposition of Au on the {100} or {111} facets, respectively, of a Ag cuboctahedron for the generation of a Ag–Au{100} or a Ag–Au{111} cuboctahedral nanobox. In a typical synthesis, we dispersed Ag cuboctahedra in an aqueous mixture containing H2Asc, NaOH (at an initial pH of 11.2), and either PVP or CTAC, followed by the titration of aqueous HAuCl4 using a syringe pump at room temperature. Under alkaline conditions, H2Asc would be neutralized into the ascorbic monoanion (HAsc−),27 a true reducing agent. Based on our previous study,28 we argue that the Ag atoms on the {111} facets would be initially oxidized by Au(III) to generate a small amount of Au atoms for their immediate deposition on the {100} facets (or the edges). With the involvement of NaOH in the solution, the resultant Ag(I) ions would react with OH− for the formation of Ag2O on the {111} facets, prohibiting the underlying Ag from further reacting with Au(III) ions. From this time point, the Au(III) ions would be reduced by HAsc− to produce more Au atoms for this subsequent deposition onto the {100} facets conformally.29 Likely, some of the newly deposited Au atoms could migrate to the Ag2O passivated {111} facets through surface diffusion.30 At the end, an Ag cuboctahedron is transformed into an Ag@Au{100} cuboctahedron with the inclusion of some Au atoms in the Ag2O regions at the {111} facets. Because the Ag2O layer can be dissolved in a weak acid,31 we treated the as-obtained products with H2Asc and then etched Ag from the core with aqueous H2O2, leading to the conversion of the Ag@Au{100} cuboctahedron into an Ag–Au{100} cuboctahedral nanobox encased by {100} facets. It is worth noting that some NaCl was added during the weak acid treatment to increase the solubility of Ag2O for an efficient dissolution of the Ag2O layer.32
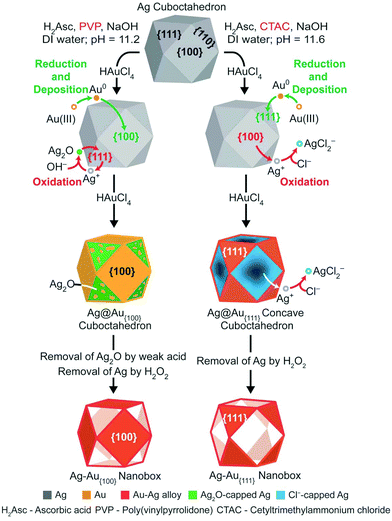 |
| Fig. 1 Schematic diagram that illustrates the two orthogonal pathways for the deposition of Au on Ag cuboctahedra in the presence of PVP and CTAC, respectively, followed by the removal of Ag for the production of cuboctahedral nanoboxes with complementary facets. | |
In comparison, when PVP in the reaction solution is replaced by CTAC, the Cl− ions derived from CTAC could strongly interact with the {100} facets, as reported by others.33 In this case, the oxidation of Ag by Au(III) would be initiated on the {100} facets, which is orthogonal to what is involved in the PVP-based synthesis.34 Additionally, the dissolved Ag(I) ions would form soluble AgCl2− ions rather than react with OH− to produce Ag2O, due to the excessive amount of the Cl− ions involved in the reaction solution.35 As a consequence, both the AgCl2− and Au(III) ions would be co-reduced by HAsc− into Ag and Au atoms, respectively, for their co-deposition onto the Ag cuboctahedra. Because the {100} facets are involved in the oxidation of Ag, we argue that the co-deposition of Au and Ag atoms would be mainly confined to the {111} facets. As HAuCl4 is titrated into the reaction solution, more and more Ag atoms would be carved away from the {100} facets, leading to the generation of an Ag@Ag–Au{111} concave cuboctahedron. After the etching of Ag from the core with aqueous H2O2, Ag–Au{111} cuboctahedral nanoboxes covered by {111} facets will be obtained.
In a typical synthesis, we first produced the Ag nanocubes with an average edge length of 38.1 ± 2.0 nm (Fig. S1†).26 The as-obtained Ag nanocubes were then used as seeds to generate Ag cuboctahedra with an average edge length of 47.2 ± 2.2 nm (Fig. S2†).15 In one set of studies, we dispersed the Ag cuboctahedra in an aqueous solution containing H2Asc, NaOH, and PVP, followed by the titration of aqueous HAuCl4 (0.1 mM) at an initial pH of 11.2. Fig. 2A shows a transmission electron microscopy (TEM) image of the product prepared by adding 0.4 mL of HAuCl4. Fig. 2B shows an aberration-corrected high-angle annular dark-field scanning TEM (HAADF-STEM) image of a single cuboctahedron taken from the sample in Fig. 2A. When aligned along the 〈001〉 zone axis, we could easily resolve the distinctive contrast between the bright region at the center and the darker regions at the four corners, which correspond to the {100} and {111} facets of the cuboctahedron, respectively. Because the heavier Au atoms (which contribute to the mass contrast) were located on the {100} facets that are parallel to the zone axis, we observed brighter contrast at the four edges. Fig. S3A and B† show two atomic-resolution HAADF-STEM images of the as-prepared Ag@Au{100} cuboctahedron in which columns of atoms were visible with no significant presence of an oxide layer. We also performed energy dispersive X-ray spectroscopy (EDS) mapping on the same cuboctahedron to confirm the spatial distribution of each element. Fig. 2C and D show the elemental mapping of Ag and Au, respectively. We noticed that more signals from Ag were distributed at the center face than at the four corners, consistent with the thickness contrast of a cuboctahedron along the 〈001〉 zone axis. On the other hand, the signals from Au were mainly localized at the center face and the four edges but not at the four corners. The Au distribution coincides with the presence of four edges that appear brighter on the HAADF-STEM image in Fig. 2B, confirming the deposition onto the {100} and {110} surfaces. In comparison with the Au signal shown in Fig. 2D, Fig. S4A and B† show the same particle and the elemental mapping of oxygen. Because the oxygen signals were present both outside and inside the nanocrystal, we argue that it is difficult to use EDS to confirm the presence of the oxide layer. Altogether, we were not able to characterize the Ag2O layer underneath Au by STEM and EDS. Fig. 2E and F show the TEM and SEM images of the final nanoboxes from which we clearly observed the formation of {100} facets. Because all facets are well connected in a nanobox, we argue that Au deposition would also occur on the {110} facets of the Ag cuboctahedra, serving as the vertices of cuboctahedral nanoboxes. Using inductively coupled plasma mass spectroscopy (ICP-MS), we determined that the Ag–Au{100} nanoboxes were made of an Au–Ag alloy with a composition of Ag64Au36.
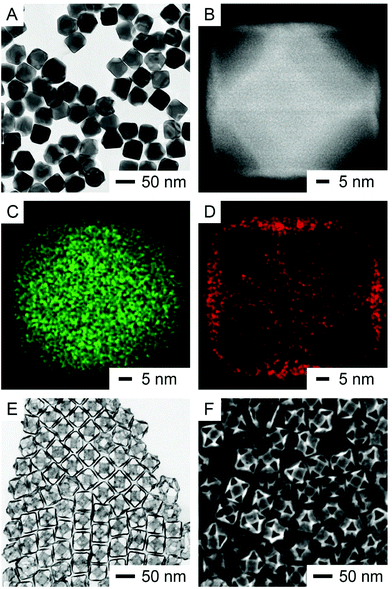 |
| Fig. 2 (A) TEM image of the as-prepared Ag@Au{100} cuboctahedra. (B) HAADF-STEM image of one nanocrystal that was orientated along the 〈001〉 zone axis. (C, D) EDS mapping of the same nanocrystal (green: Ag; red: Au). (E) TEM and (F) SEM images of the resultant Ag–Au{100} cuboctahedral nanoboxes after the removal of Ag. | |
By replacing PVP with CTAC while keeping all other parameters the same, the TEM image of the products obtained after the titration of 0.8 mL of HAuCl4 is shown Fig. 3A. Fig. 3B shows the HAADF-STEM image of one nanoparticle taken from the sample in Fig. 3A. The particle was oriented along the 〈001〉 zone axis, confirming the concave features on the {100} facets of the cuboctahedron due to the removal of Ag from these facets. As shown in Fig. 3C and D, the EDS elemental mapping of Ag and Au of the same nanoparticle indicates that the Ag signals were more intense in the center region within the concave perimeter while the Au signals were located at the edges, especially more so toward the four corners, suggesting the predominant deposition of Au on the {111} facets with very few on the {100} facets. Fig. S5A† shows the HAADF-STEM image of another nanoparticle oriented along the 〈111〉 zone axis, showing a brighter contrast at the {111} facet due to the thickness contrast. In addition, the three {100} surfaces situated at an angle of 120° from each other show a darker contrast, revealing their concavity. As shown by the EDS mapping in Fig. S5B,† the Ag signals are more intense at the center, generally following the contrast distribution in Fig. S5A.† Fig. S5C† shows that the Au signals are somewhat localized at the edges, which reflects the presence of Au atoms on the {111} facets. Away from the edges, the Au signals seem to be spread out across the particle because of the contribution of Au signals from the three {100} facets at the bottom of the cuboctahedron. After the etching of Ag from the core with aqueous H2O2, Fig. 3E and F show the TEM and SEM images of the resultant nanoboxes enclosed by {111} facets, respectively, indicating that the {100} facets of concave cuboctahedra would be covered by a small amount of Au. The ICP-MS measurement indicates that the Ag–Au{111} nanoboxes are made of Ag77Au23. Interestingly, the composition of Au in these nanoboxes prepared with 0.8 mL of HAuCl4 was lower than that in the Ag–Au{100} nanoboxes (with a composition of Ag64Au36) prepared with 0.4 mL of HAuCl4 in the presence of PVP. We suspect that part of this difference arises from the co-deposition of both Ag and Au atoms on the {111} facets of the cuboctahedra during the synthesis involving CTAC.
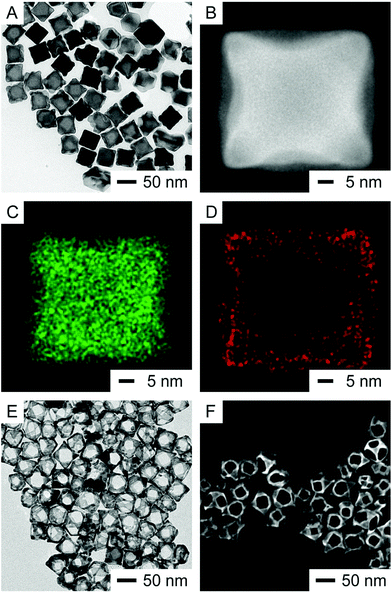 |
| Fig. 3 (A) TEM image of the as-prepared Ag@Ag–Au{111} concave cuboctahedra. (B) HAADF-STEM image of one nanocrystal that was orientated along the 〈001〉 zone axis. (C, D) EDS mapping of the same nanocrystal (green: Ag; red: Au). (E) TEM and (F) SEM images of the resultant Ag–Au{111} cuboctahedral nanoboxes after the etching of Ag. | |
We also used UV-vis-NIR spectroscopy to characterize the optical properties of the resultant cuboctahedral nanoboxes. When Ag@Au{100} cuboctahedra were transformed into Ag–Au{100} nanoboxes, the localized surface-plasmon resonance (LSPR) peak red shifted from 463 nm to 1290 nm, together with the emergence of three other peaks at 845, 925, and 1140 nm as shown in Fig. 4A. In comparison, Fig. 4B shows that the major LSPR peak of Ag@Ag–Au{111} concave cuboctahedra was located at 499 nm, which further red shifted from that of the Ag@Au{100} cuboctahedra located at 463 nm due to the surface concavity. When they were transformed into Ag–Au{111} nanoboxes, the LSPR peak shifted to 1305 nm with another peak appearing at 1175 nm. We could attribute the main LSPR peak of both types of nanoboxes (1295 and 1305 nm for Ag–Au{100} and Ag–Au{111}, respectively) to the electric dipole mode, which has a strong correlation with the dimensions of the nanobox.36 Unfortunately, it remains extremely challenging to measure the thickness of these nanoboxes while the composition can only be obtained by ICP-MS measurements. As such, we cannot perform the discrete dipole approximation (DDA) calculation to make assignments for specific plasmonic modes. Compared to the cubic Au nanoboxes with the LSPR peak located at 1080 nm,29 the LSPR peaks of these two types of Ag–Au cuboctahedral nanoboxes further shifted to the infrared region.
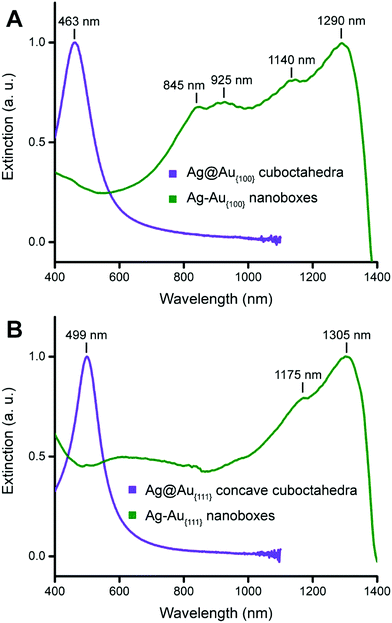 |
| Fig. 4 (A) UV-vis spectra of the Ag@Au{100} cuboctahedra and the corresponding Ag–Au{100} nanoboxes. (B) UV-vis spectra of the Ag@Ag–Au{111} concave cuboctahedra and the corresponding Ag–Au{111} nanoboxes. Each spectrum was normalized to its maximum intensity. | |
We evaluated the catalytic activity of the cuboctahedral nanoboxes using a model reaction based on the reduction of 4-nitrophenol (4-NP) to 4-aminophenol (4-AP) by NaBH4.37 After the introduction of NaBH4, we recorded the UV-vis spectra of 4-NP at different reaction time points (Fig. 5A and C). By following the intensity of the absorption peak at 400 nm, we plotted ln(At/A0) as a function of reaction time (Fig. 5B and D). In both cases, the reduction reaction exhibited first-order kinetics, from which we obtained the rate constant through curve fitting. The rate constants of Ag–Au{100} and Ag–Au{111} cuboctahedral nanoboxes were 0.005 min−1 and 0.213 min−1, respectively, indicting distinctive catalytic activities toward this reduction reaction. We believe that there are several factors that could contribute to this significant difference. Based on our ICP-MS measurements, both Ag–Au{100} and Ag–Au{111} cuboctahedral nanoboxes were made of an Ag–Au alloy with compositions of Ag64Au36 and Ag77Au23, respectively. As many groups argue that Au is a more effective catalyst for the reduction of 4-NP compared to Ag,38–43 it is possible that the difference arises from the fact that the Ag–Au{111} cuboctahedral nanoboxes may consist of Au-dominated outermost surfaces while the Ag–Au{100} cuboctahedral nanoboxes could consist of Ag-dominated surfaces. However, it is indeed extremely difficult to characterize the composition of the outermost surfaces to fully support this argument. On the other hand, we believe that the use of PVP or CTAC in the original synthesis of these nanoboxes could contribute to different arrangements of atoms on the {100} or {111} facets of cuboctahedra, respectively, leading to their distinct catalytic activities. Finally, it is also possible that the dissolved oxygen species in reaction solution could be attributed to different induction times of 4-NP reduction because Ag atoms could leach out into the solution as Ag ions and thus affect the catalytic performance.44,45
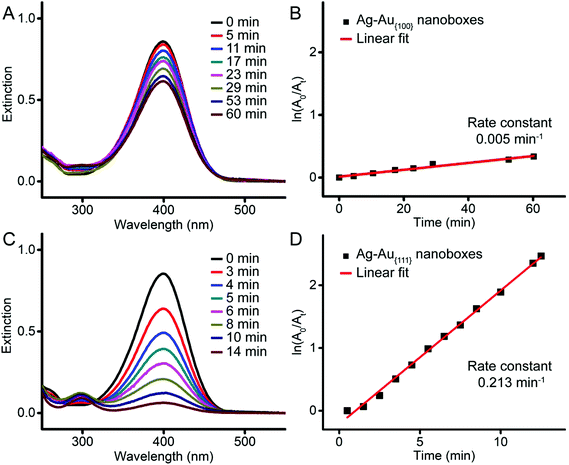 |
| Fig. 5 (A, C) UV-vis spectra recorded at different time points for the reduction of 4-NP by NaBH4 at room temperature, when the Ag–Au{100} and Ag–Au{111} nanoboxes were used as the catalysts, respectively. (B, D) Plots of ln(A0/At) as a function of time for the peaks located at 400 nm in (A) and (C), respectively. | |
Conclusions
We have demonstrated the transformation of Ag cuboctahedra into Ag–Au cuboctahedral nanoboxes with complementary facets by including either PVP or CTAC to a reaction solution containing Ag cuboctahedra, H2Asc, and NaOH at a pH value around 11, followed by the titration of aqueous HAuCl4. When PVP was involved, the oxidation of Ag would be initiated on the {111} facets for the deposition of Au on the {100} facets in an orthogonal manner, transforming Ag cuboctahedra into Ag@Au{100} cuboctahedra and then cuboctahedral nanoboxes enclosed by the {100} facets after the etching of Ag by H2O2. In comparison, when CTAC was added to the reaction solution, the oxidation of Ag would continuously progress on the {100} facets for the generation of concavities. Concomitantly, the dissolved Ag(I) and Au(III) ions would be co-reduced by chemical reduction for the generation of Ag and Au atoms, followed by their co-deposition on the {111} facets for the generation of Ag@Au{111} concave cuboctahedra and the resultant Ag–Au{111} cuboctahedral nanoboxes enclosed by {111} facets after the removal of Ag. Both types of cuboctahedral nanoboxes exhibit strong LSPR properties in the infrared region. On the other hand, cuboctahedral nanoboxes enclosed by the {111} facets exhibit catalytic activities toward the reduction of 4-NP by NaBH4.
Conflicts of interest
There are no conflicts to declare.
Acknowledgements
We thank the National Science Foundation (CHE-1708300) for the financial support of this project. We also acknowledge the use of the material characterization facility at the Institute of Electronics and Nanotechnology (IEN) at GT.
References
- M. Rycenga, C. M. Cobley, J. Zeng, W. Li, C. H. Moran, Q. Zhang, D. Qin and Y. Xia, Controlling the Synthesis and Assembly of Silver Nanostructures for Plasmonic Applications, Chem. Rev., 2011, 111, 3669–3712 CrossRef CAS PubMed.
- C. J. Orendorff, T. K. Sau and C. J. Murphy, Shape-Dependent Plasmon-Resonant Gold Nanoparticles, Small, 2006, 2, 636–639 CrossRef CAS PubMed.
- N. Tian, Z.-Y. Zhou, S.-G. Sun, Y. Ding and Z. L. Wang, Synthesis of Tetrahexahedral Platinum Nanocrystals with High-Index Facets and High Electro-Oxidation Activity, Science, 2007, 316, 732–735 CrossRef CAS PubMed.
- A. R. Tao, S. Habas and P. Yang, Shape Control of Colloidal Metal Nanocrystals, Small, 2008, 4, 310–325 CrossRef CAS.
- M. H. Huang and P.-H. Lin, Shape-Controlled Synthesis of Polyhedral Nanocrystals and Their Facet-Dependent Properties, Adv. Funct. Mater., 2012, 22, 14–24 CrossRef CAS.
- C.-Y. Chiu, P.-J. Chung, K.-U. Lao, C.-W. Liao and M. H. Huang, Facet-Dependent Catalytic Activity of Gold Nanocubes, Octahedra, and Rhombic Dodecahedra toward 4-Nitroaniline Reduction, J. Phys. Chem. C, 2012, 116, 23757–23763 CrossRef CAS.
- S. Xie, S.-I. Choi, X. Xia and Y. Xia, Catalysis on faceted noble-metal nanocrystals: both shape and size matter, Curr. Opin. Chem. Eng., 2013, 2, 142–150 CrossRef.
- Q. Zhang and H. Wang, Facet-Dependent Catalytic Activities of Au Nanoparticles Enclosed by High-Index Facets, ACS Catal., 2014, 4, 4027–4033 CrossRef CAS.
- Y. Xia, K. D. Gilroy, H.-C. Peng and X. Xia, Seed-Mediated Growth of Colloidal Metal Nanocrystals, Angew. Chem., Int. Ed., 2017, 56, 60–95 CrossRef CAS PubMed.
- W. Niu, L. Zhang and G. Xu, Seed-mediated growth of noble metal nanocrystals: crystal growth and shape control, Nanoscale, 2013, 5, 3172–3181 RSC.
- Y. Wu, X. Sun, Y. Yang, J. Li, Y. Zhang and D. Qin, Acc. Chem. Res., 2017, 50, 1774–1784 CrossRef CAS PubMed.
-
A. W. Adamson and A. P. Gast, Physical chemistry of surfaces, Interscience, New York, 1967 Search PubMed.
- B. Nikoobakht and M. A. El-Sayed, Preparation and Growth Mechanism of Gold Nanorods (NRs) Using Seed-Mediated Growth Method, Chem. Mater., 2003, 15, 1957–1962 CrossRef CAS.
- S. E. Habas, H. Lee, V. Radmilovic, G. A. Somorjai and P. Yang, Shaping binary metal nanocrystals through epitaxial seeded growth, Nat. Mater., 2007, 6, 692 CrossRef CAS PubMed.
- Y. Wang, D. Wan, S. Xie, X. Xia, C. Z. Huang and Y. Xia, Synthesis of Silver Octahedra with Controlled Sizes and Optical Properties via Seed-Mediated Growth, ACS Nano, 2013, 7, 4586–4594 CrossRef CAS PubMed.
- J. Zhang, J. Liu, Z.-X. Xie and D. Qin, HAuCl4: A Dual Agent for Studying the Chloride-Assisted Vertical Growth of Citrate-Free Ag Nanoplates with Au Serving as a Marker, Langmuir, 2014, 30, 15520–15530 CrossRef CAS PubMed.
- C.-W. Wang, X. Sun, H.-T. Chang and D. Qin, Generation of Enzymatic Hydrogen Peroxide to Accelerate the Etching of Silver Nanocrystals with Selectivity, Chem. Mater., 2016, 28, 7519–7527 CrossRef CAS.
- M. R. Langille, M. L. Personick, J. Zhang and C. A. Mirkin, Bottom-Up Synthesis of Gold Octahedra with Tailorable Hollow Features, J. Am. Chem. Soc., 2011, 133, 10414–10417 CrossRef CAS PubMed.
- D. Kim, Y. W. Lee, S. B. Lee and S. W. Han, Convex Polyhedral Au@Pd Core–Shell Nanocrystals with High-Index Facets, Angew. Chem., Int. Ed., 2012, 51, 159–163 CrossRef CAS PubMed.
- S. Xie, N. Lu, Z. Xie, J. Wang, M. J. Kim and Y. Xia, Synthesis of Pd-Rh Core–Frame Concave Nanocubes and Their Conversion to Rh Cubic Nanoframes by Selective Etching of the Pd Cores, Angew. Chem., Int. Ed., 2012, 51, 10266–10270 CrossRef CAS PubMed.
- R. G. Weiner, M. R. Kunz and S. E. Skrabalak, Seeding a New Kind of Garden: Synthesis of Architecturally Defined Multimetallic Nanostructures by Seed-Mediated Co-Reduction, Acc. Chem. Res., 2015, 48, 2688–2695 CrossRef CAS PubMed.
- J. Li, X. Sun and D. Qin, Ag-Enriched Ag-Pd Bimetallic Nanoframes and Their Catalytic Properties, ChemNanoMat, 2016, 2, 494–499 CrossRef CAS.
- N. S. R. Satyavolu, L. H. Tan and Y. Lu, DNA-Mediated Morphological Control of Pd–Au Bimetallic Nanoparticles, J. Am. Chem. Soc., 2016, 138, 16542–16548 CrossRef CAS PubMed.
- L. Zhang, Z. Xie and J. Gong, Shape-Controlled Synthesis of Au-Pd Bimetallic Nanocrystals for Catalytic Applications, Chem. Soc. Rev., 2016, 45, 3916–3934 RSC.
- X. Sun, D. Li, Y. Ding, W. Zhu, S. Guo, Z. L. Wang and S. Sun, Core/Shell Au/CuPt Nanoparticles and Their Dual Electrocatalysis for Both Reduction and Oxidation Reactions, J. Am. Chem. Soc., 2014, 136, 5745–5749 CrossRef CAS PubMed.
- Q. Zhang, W. Li, L.-P. Wen, J. Chen and Y. Xia, Facile Synthesis of Ag Nanocubes of 30 to 70 nm in Edge Length with CF3COOAg as a Precursor, Chem. – Eur. J., 2010, 16, 10234–10239 CrossRef CAS PubMed.
- J. Du, J. J. Cullen and G. R. Buettner, Ascorbic acid: Chemistry, biology and the treatment of cancer, Biochim. Biophys. Acta, Rev. Cancer, 2012, 1826, 443–457 CrossRef CAS PubMed.
- X. Sun, J. Kim, K. D. Gilroy, J. Liu, T. A. F. König and D. Qin, Gold-Based Cubic Nanoboxes with Well-Defined Openings at the Corners and Ultrathin Walls Less Than Two Nanometers Thick, ACS Nano, 2016, 10, 8019–8025 CrossRef CAS PubMed.
- Y. Yang, J. Liu, Z.-W. Fu and D. Qin, Galvanic Replacement-Free Deposition of Au on Ag for Core–Shell Nanocubes with Enhanced Chemical Stability and SERS Activity, J. Am. Chem. Soc., 2014, 136, 8153–8156 CrossRef CAS PubMed.
- X. Xia, S. Xie, M. Liu, H.-C. Peng, N. Lu, J. Wang, M. J. Kim and Y. Xia, On the role of surface diffusion in determining the shape or morphology of noble-metal nanocrystals, Proc. Natl. Acad. Sci. U. S. A., 2013, 110, 6669–6673 CrossRef CAS PubMed.
- H. L. Johnston, F. Cuta and A. B. Garrett, The Solubility of Silver Oxide in Water, in Alkali and in Alkaline Salt Solutions. The Amphoteric Character of Silver Hydroxide, J. Am. Chem. Soc., 1933, 55, 2311–2325 CrossRef CAS.
- X. Li, J. J. Lenhart and H. W. Walker, Aggregation Kinetics and Dissolution of Coated Silver Nanoparticles, Langmuir, 2012, 28, 1095–1104 CrossRef CAS PubMed.
- S. Zhou, J. Li, K. D. Gilroy, J. Tao, C. Zhu, X. Yang, X. Sun and Y. Xia, Facile Synthesis of Silver Nanocubes with Sharp Corners and Edges in an Aqueous Solution, ACS Nano, 2016, 10, 9861–9870 CrossRef CAS PubMed.
- J. Ahn, D. Wang, Y. Ding, J. Zhang and D. Qin, Site-Selective Carvings and Co-Deposition: Transformation of Ag Nanocubes into Concave Nanocrystals Encased by Au-Ag Alloy Frames, ACS Nano, 2018, 12, 298–307 CrossRef CAS PubMed.
- J. Du, Z. Chen, C. Chen and T. J. Meyer, A Half-Reaction Alternative to Water Oxidation: Chloride Oxidation to Chlorine Catalyzed by Silver Ion, J. Am. Chem. Soc., 2015, 137, 3193–3196 CrossRef CAS PubMed.
- A. M. Schwartzberg, T. Y. Olson, C. E. Talley and J. Z. Zhang, Synthesis, Characterization, and Tunable Optical Properties of Hollow Gold Nanospheres, J. Phys. Chem. B, 2006, 110, 19935–19944 CrossRef CAS PubMed.
- N. Pradhan, A. Pal and T. Pal, Catalytic Reduction of Aromatic Nitro Compounds by Coinage Metal Nanoparticles, Langmuir, 2001, 17, 1800–1802 CrossRef CAS.
- P. Xu, L. Kang, N. H. Mack, K. S. Schanze, X. Han and H.-L. Wang, Mechanistic understanding of surface plasmon assisted catalysis on a single particle: cyclic redox of 4-aminothiophenol, Sci. Rep., 2013, 3, 2997 CrossRef PubMed.
- J. Li, J. Liu, Y. Yang and D. Qin, Bifunctional Ag@Pd-Ag Nanocubes for Highly Sensitive Monitoring of Catalytic Reactions by Surface-Enhanced Raman Spectroscopy, J. Am. Chem. Soc., 2015, 137, 7039–7042 CrossRef CAS PubMed.
- P. Zhao, X. Feng, D. Huang, G. Yang and D. Astruc, Basic Concepts and Recent Advances in Nitrophenol Reduction by Gold- and Other Transition Metal Nanoparticles, Coord. Chem. Rev., 2015, 287, 114–136 CrossRef CAS.
- Y. Shin, A. Dohnalkova and Y. Lin, Preparation of Homogeneous Gold-Silver Alloy Nanoparticles Using the Apoferritin Cavity as a Nanoreactor, J. Phys. Chem. C, 2010, 114, 5985–5989 CrossRef CAS.
- L. Sun, P. Lv, H. Li, F. Wang, W. Su and L. Zhang, One-Step Synthesis of Au-Ag Alloy Nanoparticles Using Soluble Starch and Their Photocatalytic Performance for 4-Nitrophenol Degradation, J. Mater. Sci., 2018, 53, 15895–15906 CrossRef CAS.
- L. Sun, Y. Yin, F. Wang, W. Su and L. Zhang, Facile One-Pot Green Synthesis of Au-Ag Alloy Nanoparticles Using Sucrose and Their Composition-Dependent Photocatalytic Activity for The Reduction of 4-Nitrophenol, Dalton Trans., 2018, 47, 4315–4324 RSC.
- E. Menumerov, R. A. Hughes and S. Neretina, One-Step Catalytic Reduction of 4-Nitrophenol through the Direct Injection of Metal Salts into Oxygen-Depleted Reactants, Catal. Sci. Technol., 2017, 7, 1460–1464 RSC.
- E. Menumerov, R. A. Hughes, S. D. Golze, R. D. Neal, T. B. Demille, J. C. Campanaro, K. C. Kotesky, S. Rouvimov and S. Neretina, Identifying the True Catalyst in the Reduction of 4-Nitrophenol: A Case Study Showing the Effect of Leaching and Oxidative Etching Using Ag Catalysts, ACS Catal., 2018, 8, 8879–8888 CrossRef CAS.
Footnotes |
† Electronic supplementary information (ESI) available. See DOI: 10.1039/c9nr08420g |
‡ These authors contributed equally to this work. |
|
This journal is © The Royal Society of Chemistry 2020 |
Click here to see how this site uses Cookies. View our privacy policy here.