Hematoporphyrin monomethyl ether-mediated photodynamic therapy inhibits the growth of keloid graft by promoting fibroblast apoptosis and reducing vessel formation
Received
7th September 2019
, Accepted 6th January 2020
First published on 8th January 2020
Abstract
Photodynamic therapy (PDT) has been shown to significantly inhibit fibroblast activity. However, the effect of PDT mediated by the photosensitizer hematoporphyrin monomethyl ether (HMME) on keloids is not known well. The aim of our study was to examine the efficacy of HMME-PDT in cellular and animal models of keloids. Keloid fibroblasts (KFbs) were isolated from human keloid specimens and the proliferation, invasion, and migration of KFbs after HMME-PDT treatment was examined in vitro. Apoptosis in cells was measured by flow cytometry. Cysteinyl aspartate specific proteinase 3 (Caspase3) expression was determined by immunofluorescence staining and western blot. HMME-PDT inhibited KFbs proliferation, invasion, migration, increased apoptosis rate and enhanced caspase3 and cleaved caspase3 expression. The keloid graft transplantation was performed by using nude mice. The growth of the graft was monitored every third day. Interleukin-6 (IL-6) and tumor necrosis factor-α (TNF-α) mRNA expression were detected by quantitative real time PCR. It was observed that HMME-PDT attenuated graft growth and reduced vessel density in the keloid grafts. However, HMME-PDT did not alter IL-6 and TNF-α mRNA expression in the keloid grafts. Moreover, HMME-PDT suppressed transforming growth-β1 (TGF-β1) and small phenotype and Drosophila Mothers Against Decapentaplegic 3 (Smad3) expression in both KFbs and keloid grafts. Collectively, the evidence suggests that HMME-PDT inhibits the growth of the keloid graft by promoting the apoptosis of fibroblasts and reducing vessel formation of the keloid graft.
1. Introduction
Keloids are characterized by excessive fibroblast growth and collagen production, a clinically challenging problem.1 These abnormal fibrous growths often cause itching and pain and can be disfiguring and reoccur frequently.2,3 Keloids usually induce physical and psychological stress on the patient due to their impaired appearance.4,5 Keloids are conventionally managed by comprehensive therapy including corticosteroid injection, surgical excision, radiation therapy, silicone gel sheeting and pressure therapy.6–8 However, none of these treatments provide satisfactory outcomes in the clinic. Thus, more novel technologies are being developed. Mesenchymal stem cells play a multifunctional role in the regulation of inflammation and have gained increasing attention as candidates for the prevention or treatment of excessive scars.9 Neodymium-doped yttrium aluminum garnet (Nd:YAG) laser treatment is also regarded as a safe and effective therapeutic modality for hypertrophic scars and keloids.10 Photodynamic therapy (PDT) is a relatively new approach and is considered to be useful for the treatment of ocular diseases, tumors, bacterial and fungal infections and scars.11–16 Hematoporphyrin monomethyl ether (HMME) was developed by The Second Military Medical University in China as a kind of potential photosensitizer for PDT which marketed as hemoportfin.17 On December 3, 2016, HMME was launched into the market and applied in clinic and China Food and Drug Administration approved of the application in treating PWS. Meanwhile, HMME has many advantages such as fast metabolism, shorter time to avoid light, single structure and stable chemical composition. The ability of selective binding was stronger and the clinical adverse reactions were smaller. To date, HMME-PDT has been tested in clinical trials as a treatment for capillary malformations via vascular targeting. Cai et al. showed that low- or high-level exposure to HMME-PDT may induce fibroblast apoptosis or necrosis respectively.18 Studies on tissue-engineered keratinocyte fibroblast co-culture systems, in which keratinocytes are layered on top of fibroblasts embedded in a collagen matrix, have additionally shown that PDT suppresses fibroblast viability, down-regulates collagen expression and reduces keloid mass after 5-aminolevulinic acid (5-ALA) incubation and laser irradiation at 635 nm.19 However, information regarding the effect of HMME-PDT on keloids is still unknown, particularly in animal models.
Therefore, the effects of HMME-PDT on keloid fibroblasts (KFbs) viability in vitro was initially investigated in this study. Further, the efficacy and mechanisms of HMME as a photosensitizer for vascular targeting was evaluated in a nude mouse model where triamcinolone acetonide (TA) served as a positive control. This investigation was beneficial in allowing the proposal of a novel therapeutic approach for the management of keloids.
2. Materials and methods
2.1. Patients
Keloid samples were obtained from six patients (20–37 years old, average age 25.3 years) undergoing keloid excision surgery. None of the patients had received prior treatment (Table 1). Human tissues were used in accordance to the principles outlined in the Declaration of Helsinki. The study protocol was approved by the ethics committee of Affiliated Hospital of Nantong University. Written informed consent was obtained prior to use of keloid tissues. Following informed consent, human skin samples were harvested by removing the epidermis and adipose tissue. Next, the tissues were cut into 3 mm × 8 mm × 10 mm blocks. Care was taken to avoid contamination of the tissues.
Table 1 The profile of each sample
|
Sex |
Age (years) |
Biopsy site |
Duration of the lesion |
Etiology |
KD 1 |
Male |
25 |
Chest, shoulder |
8 years |
Acne |
KD 2 |
Female |
22 |
Chest |
4 years |
Surgery |
KD 3 |
Male |
23 |
Shoulder |
5 years |
Unknown |
KD 4 |
Female |
37 |
Monsveneris |
13 years |
Unknown |
KD 5 |
Male |
25 |
Chest |
5 years |
Acne |
KD 6 |
Female |
20 |
Chest |
3 years |
Epifolliculitis |
2.2. Primary keloid fibroblast culture
Keloid tissues were washed with phosphate-buffered saline (PBS) and cut into small pieces (1 mm3) after careful removal of the epidermis and surrounding adipose. Next, KFbs were cultured in the flasks containing Dulbecco's modified Eagle's medium (DMEM) supplemented with 10% fetal bovine serum (FBS, Gibco), 100 μg ml−1 of streptomycin and 100 U ml−1 of penicillin. The fibroblasts grew out of the tissues after 10 to 12 days of culture. KFbs passaged between 3 to 6 were used for the experimental procedures.
2.3. Device for HMME-PDT
The laser equipment used in the experiment was provided by Wuhan Lingyun Photoelectric Technology Co., Ltd, and the product model is FD532-5-A. The wavelength of the laser device is 532 nm(±5 nm) which was chosen for its wavelength is close to the absorption peak of oxyhemoglobin 542 nm.
2.4. HMME-PDT in cells
Collecting enough cells, the cells were inoculated into culture plate and different doses of the drug were added into the medium (equal volume between groups) to produce different concentrations. Next, cells were cultured in darkness for avoiding light for 4 h. A 532 nm wavelength of green light was then irradiated vertically onto the culture plate. Tinfoil was used to cover the non-light groups of cells residing on the same culture plate and steriled gauze was inserted into the gaps of the culture plate to help minimize the interference of light between groups. When the light treatment was terminated, the medium was immediately aspirated and cells were washed with PBS. Finally, DMEM culture medium containing 10% fetal bovine serum was added.
2.5. HMME-PDT in transplantation
Half a month after the keloids were implanted in the nude mice, HMME was injected into nude mice through the tail vein at a dose of 0.4 μg g−1, and then waitting for 10 minutes patiently. The keloid graft was exposed to 532 nm semiconductor laser for 10 minutes. After the irradiation, the nude mice were kept for a week and then irradiated again. We will evaluate the graft in nude mice at 14 days after first exposure.
2.6. Cell proliferation assay
Cell proliferation was assessed by Cell Counting Kit-8 assay (CCK-8; Dojindo Molecular Technologies, Gaithersburg, MD, USA) according to the manufacturer's instruction. Briefly, KFbs were seeded in 96-well plates at a density of 5 × 103 cells per well. KFbs were treated by 100 mW cm−2 laser (532 nm laser 10 min), HMME-PDT (100 mW cm−2 laser + 5 μg ml−1 HMME, 100 mW cm−2 laser + 15 μg ml−1 HMME, 100 mW cm−2 laser + 30 μg ml−1 HMME, 100 mW cm−2 laser + 50 μg ml−1 HMME, 200 mW cm−2 laser + 5 μg ml−1 HMME, 200 mW laser + 15 μg ml−1 HMME, through the tail vein, 532 nm laser 10 min), TA (1 mg ml−1) and DMEM, which was serviced as the control group. Next, the CCK-8 was employed to quantitatively evaluate cell viability. DMEM (100 μl), FBS (10%) and 10 μl CCK-8 (C0038, Beyotime Institute) was added to each pre-cultured film and incubated at 37 °C. The absorbance was determined at a wavelength of 450 nm with a microplate reader (Multi-skan MK33, Thermo Electron Corporation, China) at 0 h, 24 h, 48 h, 72 h and 96 h.
2.7. Scratch migration assay
Fibroblasts were seeded into a 6-well plates, then take diverse treatments, and scratched by 100 μl pipette tips. The detached cells were removed twice by PBS wash. The scratched cells were incubated in medium at 37 °C in a humidified 5% CO2 incubator. Wound closures were analyzed at 0 h, 24 h and 48 h and the cell wound-healing rate was calculated.
2.8. Matrigel invasion assay
Cell invasion assays were conducted by 24-well Transwell chamber with 8 mm-pores (Costar, New York, NY). Inserts were coated with 30 ml of Matrigel. After different treatments, fibroblasts (5 × 103) were seeded into the upper Matrigel chamber containing 100 ml of serum-free medium and incubated for 24 h. Subsequently, 10% DMEM was added into the lower chamber as the chemoattractant. Cells were fixed and stained with 0.1% crystal violet containing formaldehyde and the numbers of invading cells were imaged and quantified across five randomly selected fields (×100) under a microscope.
2.9. Flow cytometry
Keloid fibroblasts of logarithmic growth stage were inoculated in six-well plates and intervention was performed in the next day. After 24 h, the cell suspension was digested and prepared and the cell concentration was adjusted to 1 × 106 ml−1. Cells were centrifuge for 5 minutes, 1000 rpm, 4 °C. Discarding the supernatant, the cells were resuspended in 195 μl buffer and 5 μl Annexin V-FITC, blending gently and incubating at room temperature in dark for 10 minutes. Cells were centrifuge for 5 minutes, 1000 rpm, 4 °C. Discarding the supernatant, cells were resuspended in 190 μl Annexin V-FITC and 10 μl propidium iodide, and the flow cytometry was proceeded.
2.10. Transplantation of keloid grafts and experimental treatments
All animal studies were performed using protocols approved by the department of Animal Center, Medical College of Nantong University. Four to six week old (18 g ± 2 g) male Bagg albino laboratory bred (BALB)/c-nu/nu nude mice were maintained in a Specefic Pathogen Free environment. All nude mice were acclimated for 2 weeks (20 g ± 2 g) prior to transplantation. An 8 mm length incision was made using eye scissors for the transplantation of keloid tissues. The incision was sutured after the keloid graft filled the lacuna between skin and subcutaneous tissue. Nude mice were subsequently treated by HMME-PDT (100 mW cm−2 laser + 8 μg HMME per mouse, through the tail vein, 532 nm laser 10 min), TA (0.1 mg per mouse with no light) and normal saline on day 7 and day 14. The injection dose in volume of TA group and NS group was 0.1 ml. The control group was maintained with no light and no injection.
2.11. Western blot
All nude mice were anesthetized with chloral hydrate (10% solution) and the neck subcutaneous keloid blocks were surgically removed and flash-frozen at −80 °C 21 days after transplantation. Frozen keloid tissues were thawed and homogenized in lysis buffer followed by centrifugation at 12
000 rpm at 4 °C for 20 min for the collection of the supernatant. Cell cultures for immunoblot were lysed with sodium lauryl sulfate loading buffer and stored at −80 °C until use. The supernatant was subjected to SDS-polyacrylamide gel electrophoresis after determining the protein concentration with the Bradford assay. After the separated proteins were transferred to polyvinylidene difluoride filter membranes, the membranes were blocked with 1% (w/v) bovine serum albumin (BSA) for 2 h and incubated with the following primary antibodies: transforming growth factor-β1 (TGF-β1) (1
:
1000, Sigma-Aldrich), Sma and Mad homologue 3 (Smad3) (1
:
1000, Sigma-Aldrich), leukocyte differentiation antigen 34 (CD34) (1
:
1000, Cell Signaling Technology), cysteinyl aspartate specific proteinase 3 (caspase3) (1
:
1000; Cell Signaling Technology) and glyceraldehyde-3-phosphate dehydrogenase (GAPDH) (1
:
1000, Cell Signaling Technology) at 4 °C overnight. After 3 round of 5 min PBS wash, the membranes were incubated with secondary antibodies for 2 h at 4 °C overnight. Bound proteins were visualized by Chemi Doc XRS system (Hercules, CA).
2.12. Immunofluorescent staining
Fibroblasts were seeded onto coverslips in 24-well plate formation and cultured overnight after treatment. All cells were fixed in 4% para-formaldehyde for 30 min, permeabilized in 0.1% Triton X-100 for 30 min, blocked with 10% normal serum blocking solution containing 3% bovine serum albumin (BSA), and 0.05% Tween-20 for 2 h at room temperature to avoid non-specific staining. The fixed cells or frozen keloid sections were incubated in primary antibodies raised against vimentin (vim) (1
:
1000; Sigma), caspase3 (1
:
1000; Cell Signaling Technology) and CD34 (1
:
1000; Cell Signaling Technology) at 4 °C overnight. After 3, 5 min PBS washes, the fibroblasts were incubated in a mixture of FITC-and TRITC-conjugated secondary antibodies for 2 h at 4 °C overnight. In order to detect the morphology of apoptotic cells, cells were incubated in 4′,6-diamidino-2-phenylindole (DAPI) (0.1 mg ml−1 in PBS; Sigma) for 10 minutes at room temperature. The cells were washed in PBS a final time and coverslipped. Cells and tissues were examined by Leica microscope (Leica, DM 5000B; Leica CTR 5000; Germany).
2.13. Quantitative real-time PCR
Total RNA was obtained from keloid tissue via TRIZOL reagent (Invitrogen, USA) and RNA concentration was quantified by the Thermo Scientific NanodROP 2000 Spectrophotometer (Thermo Scientific, USA). Then the cDNA was reverse transcribed (Takara, Japan) and the qRT-PCR reaction was prepared according to the manufacturer's protocol including SYBRGreen qRT-PCR Master Mix (Takara, Japan). Reactions were cycled in Pikoreal 96 real-time PCR system (Thermo Scientific, USA) in order to detect gene mRNA expression level. The primers used were as follows: Interleukin-6 (IL-6) (forward: 5′-AATCATCACTGGTCTTTTGGAG-3′; reverse: 5′-GCATTGTTGGTTGGGTCA-3′), tumor necrosis factor-α (TNF-α) (forward: 5′-TTCTGAAGCCCCTCCCAGTTCT′; 5′-GTTGGGGACACACAAGCATCAA-3′; 5′-CCCTTCATTGACCTCAACTACA-3′; 5′-TGGAAGATGGTGATGGGATT-3′). The mRNA levels of GAPDH served as an internal control and final mRNA quantitation were calculated using the 2−ΔΔCt method.
2.14. Statistical analysis
All data in this paper was analyzed using Stata 13.0 statistical software (Systat Software Inc., San Jose, CA, USA) and were expressed as mean ± SEM. Differences between groups were determined by one-way analysis of variance followed by Tukey's post-hoc multiple comparison tests. P < 0.05 was considered statistically significant.
3. Results
3.1. HMME-PDT inhibited keloid fibroblast viability
To explore the potential of HMME-PDT as a keloid treatment, we first determined the effects of various doses of HMME-PDT on the viability of KFbs in culture, using microscopic imaging and the CCK-8 cell viability assay. Primary KFbs were isolated from keloids as previously mentioned, cultured for 10 days, and examined by immunofluorescent staining. KFbs displayed elongated or spindle shaped form with abundant cytoplasm. Some KFbs exhibited two or three ridges extending outward, which were typical fibroblasts morphologies. Primary KFbs were stained positive for vim, a marker of mesenchymal origin (Fig. 1A). In addition, a combination of HMME and PDT, but not PDT alone, altered the morphology of cultured KFbs (Fig. 1B). A CCK-8 assay further showed that incubation of KFbs with HMME-PDT (100 mW cm−2 laser + 5, 15, 30, 50 μg ml−1 HMME; 200 mW cm−2 laser + 5, 15 μg ml−1 HMME) diminished the cell numbers compared with the control group (Fig. 1C and D). Moreover, HMME at the concentration of 100 mW cm−2 laser + 5 μg ml−1 HMME and 100 mW cm−2 laser + 15 μg ml−1 HMME inhibited proliferation without nuclear condensation and cell fragmentation. As such, 100 mW cm−2 laser + 5 μg ml−1 HMME and 100 mW cm−2 laser + 15 μg ml−1 HMME were chosen for further experiments. These results indicated that HMME-PDT treatment effectively inhibited keloid fibroblast viability.
 |
| Fig. 1 HMME-PDT inhibits viability of KFbs. (A) Cultured KFbs were stained with vim (green) and DAPI (blue). (B) KFbs were treated by 100 mW cm−2 laser (532 nm laser 10 min), HMME-PDT (100 mW cm−2 laser + 5 μg ml−1 HMME, 100 mW cm−2 laser + 15 μg ml−1 HMME, 100 mW cm−2 laser + 30 μg ml−1 HMME, 100 mW cm−2 laser + 50 μg ml−1 HMME, 200 mW cm−2 laser + 5 μg ml−1 HMME, 200 mW cm−2 laser + 15 μg ml−1 HMME, through the tail vein, 532 nm laser 10 min), TA (1 mg ml−1) and DMEM, which was serviced as the control group. The changes of KFbs morphology were observed 24 h after treatment. (C and D) The cell viability of KFbs was determined by OD value following CCK-8 assay. Data are presented as mean ± SEM, *P < 0.05, compared with control group (n = 6). | |
3.2. HMME-PDT suppressed KFb invasion and migration
We next analyzed the influence of HMME-PDT on the invasive and migratory capacity of KFb in a scratch wound healing assay and a matrigel invasion assay. The scratch migration assay demonstrated that cells in the 100 mW cm−2 laser + 15 μg ml−1 HMME group and TA group migrated significantly shorter distances than the control group (Fig. 2C and D). In the invastion assay, the number of keloid-derived cells reaching the lower surface of the filter was decreased in the 100 mW cm−2 laser + 15 μg ml−1 HMME and TA groups compared to the control group (Fig. 2A and B). Collectively, these results demonstrated that HMME-PDT significantly inhibited KFb invasion and migration.
 |
| Fig. 2 HMME-PDT suppresses cell invasion and migration in cultured KFbs. (A and B) After treatment with HMME-PDT (100 mW cm−2 + 5 μg ml−1, 100 mW cm−2 + 15 μg ml−1) or TA (1 mg ml−1), the numbers of KFbs invading into the lower surface of the filter were measured within a transwell chamber assay. (C and D) The scratch migration assay was performed across all groups. (D) The cell wound-healing rate after 24 h and 48 h was subsequently measured. Data are presented as mean ± SEM, *P < 0.05, compared with control group (n = 6). | |
3.3. HMME-PDT promoted KFb apoptosis
To explore the effect of HMME-PDT on KFb survival, KFb apoptosis was evaluated by flow cytometry. The apoptosis rate in the 100 mW cm−2 laser + 15 μg ml−1 group and TA group were significantly higher than the control group (Fig. 3A and B). Further, immunofluorescence staining showed that the caspase 3 positive cell rate was higher in the 100 mW cm−2 laser + 15 μg ml−1 and TA groups compared to the control group (Fig. 3C and D). Moreover, the expression of cleaved caspase 3 in cells treated with 100 mW cm−2 laser + 15 μg ml−1 group and TA was higher than control levels (Fig. 3E). These results indicated that HMME-PDT treatment promoted KFb apoptosis.
 |
| Fig. 3 HMME-PDT promotes KFb apoptosis. (A and B) After treatment with HMME-PDT (100 mW cm−2 + 5 μg ml−1, 100 mW cm−2 + 15 μg ml−1) or TA (1 mg ml−1), apoptosis of KFbs was analyzed by flow cytometry. (C) Immunofluorescence staining revealed the apoptosis conditions of KFbs across different treatment groups. Cells were co-labeled via staining with vim (green), caspase3 (red) and DAPI (blue) respectively. (D) Caspase3 positive cells were calculated as a percentage of the total cell number across all treatment groups. (E) The protein level of caspase3 and cleaved caspase3 were additionally detected by western blot. GAPDH was served as a landing control. Data were presented as mean ± SEM, *P < 0.05, compared with control group (n = 6). | |
3.4. HMME-PDT inhibited keloid graft growth
To examine the effect of HMME-PDT on keloid growth in vivo, human-derived keloid tissues were transplanted into nude mice (Fig. 4A). Nude mice were treated by HMME-PDT on day 7 and day 14 (through the tail vein, 100 mW cm−2 laser + 8 μg HMME per mouse, 532 nm laser 10 min), TA group (0.1 mg) and normal saline (0.1 ml). The control group was without any treatment. The grafts were monitored regularly and finally excised and processed for hematoxylin–eosin staining to detect vascular remodeling on day 14 (before the second HMME-PDT treatment) and day 21. Microscopic examination revealed that there were fewer vessels surrounding the grafts and collagen becomes orderly and slender after HMME-PDT treatment (Fig. 4B). The graft volume was measured by calipers every three days and over the course of 21 days showed that graft volumes were reduced by HMME-PDT treatment (Fig. 4C). HMME-PDT increased the rate of volume reduction on day 14 and day 21 (Fig. 4D). These findings demonstrated that HMME-PDT effectively inhibited keloid graft growth in vivo.
 |
| Fig. 4 HMME-PDT inhibited keloid graft growth. (A) The nude mice model was completed as follows: (a) The keloid tissue was transplanted into the back of the animal model. (b and c) The stitches fell off about 7 days after surgery. Nude mice were treated by HMME-PDT (through the tail vein, 100 mW cm−2 laser + 8 μg HMME per mouse, 532 nm laser 10 min), TA group (0.1 mg per mouse) and normal saline (0.1 ml per mouse). The control group was without any treatment. (d) The grafts were excised from the animal on the 21st day. Tissue sections were stained with hematoxylin–eosin to detect vascular remodeling on day 7 and day 14. (B) Volume of grafts was measured by calipers every three days. (C) The rate of volume reduction was calculated on day 14 and day 21. Data were presented as mean ± SEM, *P < 0.05, compared with control group (n = 6). | |
3.5. HMME-PDT inhibited vessel density in keloid grafts
As the hematopoietic progenitor cell antigen 34 (CD34) is highly-expressed in vasculature, immunofluorescence staining and western blot analysis of CD34 protein was evaluated across the four groups. The number of positive cells in the four groups were respectively 12.1, 5.9, 11.8 and 12.2. Total number of cells was 80 per field. HMME-PDT inhibited CD34 expression in the keloid grafts, suggesting that the density of vessels was attenuated by HMME-PDT (Fig. 5). These results also indicated that HMME-PDT may inhibit the formation of vessels surrounding the transplanted keloid grafts.
 |
| Fig. 5 HMME-PDT inhibited vessel density in keloid grafts. (A) The grafts were stained with the immunofluorescent labels CD34 (red) and DAPI (blue). (B) Density of vessels was represented as CD34 positive cells was analyzed in different groups. The number of positive cells in the four groups were respectively 12.1, 5.9, 11.8 and 12.2. Total number of cells was 80 per field. (C) The protein level of CD34 in the keloid grafts was additionally detected by western blot. GAPDH served as a landing control. Data were presented as mean ± SEM, *P < 0.05, compared with control group (n = 6). | |
3.6. HMME-PDT reduced TGF-β1 and Smad3 expression in KFbs and keloid grafts
Mounting evidence suggests that the TGF-β family and the Smad pathway may play an important role in the pathogenesis of keloids. Accordingly, the protein level of TGF-β1 and Smad3 in KFbs (Fig. 6A) and keloid grafts (Fig. 6B) were examined by western blot. The observations demonstrated that HMME-PDT treatment (in vitro and in vivo) inhibited the expression of TGF-β1 and Smad3.
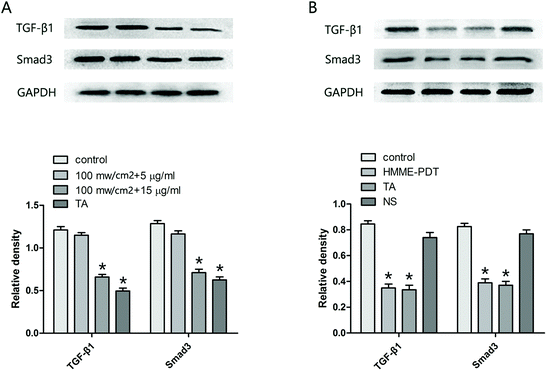 |
| Fig. 6 HMME-PDT reduced TGF-β1 and Smad3 expression in KFbs and keloid grafts. (A) After treatment with HMME-PDT (through the tail vein, 100 mW cm−2 laser + 8 μg HMME per mouse, 532 nm laser 10 min) or TA (0.1 mg per mouse), TGF-β1 and Smad3 protein in the KFbs were detected by western blot. (B) The grafts were excised on the 21st day, and TGF-β1 and Smad3 protein in the keloid grafts were once again detected by western blot. GAPDH was served as a landing control. Data were presented as mean ± SEM, *P < 0.05, compared with control group (n = 6). | |
3.7. HMME-PDT did not alter IL-6 and TNF-α mRNA expression in keloid grafts
PDT has indicated a dual role in the stimulation and inhibition of the immune response. To explore the inflammatory response of HMME-PDT in the keloid system, IL-6 and TNF-α, two main inflammatory factors, were detected by qRT-PCR. The results demonstrated that the treatment posed no significant difference in the expression of IL-6 and TNF-α in the experimental groups except for the positive control TA group, suggesting that this PDT modality was not sufficient to activate detectable changes in the inflammatory microenvironment of the keloid (Fig. 7).
 |
| Fig. 7 HMME-PDT did not alter IL-6 and TNF-α mRNA expression in keloid grafts. The grafts were excised after 21 days and the expression of IL-6 and TNF-α in the keloid grafts was detected by qRT-PCR. Data were presented as mean ± SEM, *P < 0.05, compared with control group (n = 6). | |
4. Discussion
Keloids are regarded as benign fibroproliferative growths resulting from the derangement of the normal wound healing process in susceptible individuals. Several studies have demonstrated the value of photodynamic therapy in conjunction with first generation photosensitizing agents such as 5-aminolevulinic acid (5-ALA). Either photosensitizer or light alone has demonstrated efficacy in the treatment of keloids, Golding et al. found that a combination of targeted energy, glycolysis inhibitors 2-deoxyglucose and lonidamine, and the photosensitizing agent 5-ALA exhibited elevated cytotoxicity towards the breast cancer cell line MCF-7.20 This potentiation of PDT cytotoxicity occurred only if the glycolysis inhibitors were added after ALA incubation. This suggested that the intracellular accumulation of the photosensitizer and inhibitors could derail the compound action of the PDT-photosensitizer reaction.20 Giovannini et al. similarly found that PDT combined with systemic steroid treatment provided a better outcome for the control of juxta/subfoveal idiopathic choroidal neovascularization compared with systemic steroids alone.21 Chang et al.22 investigated the molecular mechanisms involved in 5-ALA-based PDT and concluded that p53-related apoptosis and TGF-β1-mediated signaling may be crucial factors associated with the prediction and evaluation of treatment outcomes for hypertrophic scars. In our study, the photosensitizing agent HMME and PDT were examined at variable concentrations and light power in vitro.
Previous in vitro studies and clinical evaluations support the use of HMME as a promising PDT photosensitizer for the treatment of capillary malformations. Our study found that HMME-PDT treatment elevated the rate of apoptosis of keloid fibroblasts. In addition, keloid graft volume and vessel density were decreased by HMME-PDT treatment, suggesting that the inhibitory effect on vessel formation might be the vital mechanism for the attenuation of keloid growth by HMME-PDT.
In addition, several studies in vivo have suggested that HMME might be useful as both an ocular PDT and an anti-tumor PDT.17,23 HMME-PDT was found to effectively promote the death of HepG2 cells in vitro by stimulating apoptosis as demonstrated by the exponential relationship between the photodynamic parameter B (B = optical output power × irradiation time × HMME concentration) and the viability/apoptosis rate of HepG2 cells.24 Within a specific range, if the concentration was higher, the fluorescence of the apoptotic marker became stronger until the intracellular photosensitizer reached saturation at 40 μg ml−1 in a PDT paradigm applied to SKOV3 cells.25 A previous study26 compared the sensitivity of Epstein–Barr virus (EBV)-positive and -negative human nasopharyngeal carcinoma cells and concluded that EBV-CNE2 cells were significantly more sensitive than EBV + C666-1 cells to HMME-PDT. In this study the intracellular uptake of HMME was shown to play a key role in the determination of PDT sensitivity. Another study demonstrated that the photodynamic activity of HMME was mainly mediated through a type II reaction, where 1O2 was the predominant active substance in vitro and HMME was selectively accumulated in macrophages. This led to the hypothesis that HMME-PDT may induce apoptosis of macrophages via activation of caspase 9 and TNF-α pathways.4 Other studies have shown that HMME-mediated PDT an effective promoter of mitochondrial dysfunction in cancer cells and may be a promising treatment for canine breast cancer. The underlying mechanisms may be attributable to HMME-PDT's direct impact on reactive oxygen species (ROS) production as well as its indirect effect on the inhibition of mitochondrial reductase activities. The accumulation of ROS in mitochondria can lead to high levels of structural damage and compromised mitochondrial functions.27 Our present study additionally identifies that HMME-PDT has notable effects on cell invasion and migration. In conjunction with the aforementioned biological effects of the treatment, our study suggests that HMME-PDT might be an ideal strategy for the treatment of keloids. The laser wavelength was chosen based on both the deoxyghemoglobin absorption and the excitation spectra of HMME. The absorption peaks of oxyhemoglobin in the visible spectrum consists of 418 nm, 542 nm and 577 nm. 532 nm laser was chosen for its wavelength is close to the absorption peak of oxyhemoglobin 542 nm. In addition, the excitation spectra of HMME peaks contain 395 nm, 497 nm, 531 nm, 567 nm and 620 nm. For the shallow penetration in the skin, the highest peak of 395 nm was removed from the selection. The 531 nm is the optimal penetration depth which was close to wavelength of 532 nm laser. 532 nm laser were used in some experimental and clinical researches aimed to study the efficacy of hemoporfin in photodynamic therapy.28–31 Shi J et al. selected 532 nm laser + 100 mw cm−2 to deal with the tumor regions in vivo study.29 Zhao Y et al. used 532 nm continuous wave laser with a power density of 80–100 mw cm−2 for a total of 20 minutes for the treament of port-wine stain.30
Increasing evidence suggests the vital role of TGF-β family and Smad pathway in the pathogenesis of keloids.32 In addition, TGF-β/Smads also stimulate the extracellular signal regulated kinase ERK, the c-jun N-terminal, stress activated protein kinase JNK and p38 mitogen-activated protein kinase.33 Sato et al. previously showed that TGF-β induces activation of β-catenin-mediated transcription in human dermal fibroblasts via the Smad3 and p38 MAPK pathways.34 The present study adds that HMME-PDT is capable of downregulating TGF-β1 and Smad3 expression, suggesting that the dysregulation of TGF-β/Smad signaling in keloid pathogenesis can be restored by HMME-PDT.
Inflammation is one of the vital components of the therapeutic mechanisms of PDT in wound healing. The basic physiological process for cutaneous healing after PDT treatment is dynamic and complex, including complex of cellular, molecular and biochemical events that culminate in the reconstruction of damaged tissue. Recent studies have also indicated that PDT can induce a localized, acute inflammatory response.35 Immediately following PDT, myeloid cells, monocytes, macrophages and mast cells are accumulated, resulting in the activation of CD8+ T cells which subsequently contribute to the clearance of damaged cells and tissues.35,36 Other studies also have shown complementary evidence that PDT has a significant impact on neutrophil activation, likely contributing to the increase of pro-inflammatory cytokines after PDT.35,37,38 Parallel to the acute phase of the inflammation and tissue restoration regulated by PDT, lipid mediators are produced including the anti-inflammatory and immune-modulating responses. This is accompanied by leukocyte chemotaxis inhibition, TNF-α and IL-6 blockage, and simultaneous elevation of IL-10 expression.39 Two human studies have additionally highlighted that the inflammatory cytokine profile (especially the interleukins IL-1, IL-8 and TNF-α) is inhibited after PDT.35 Animal studies have similarly reported the down-regulation of pro-inflammatory cytokines after PDT application.40 Therefore, PDT has demonstrated a dynamic role in the regulation of the immune response. In the present study, IL-6 and TNF-α evaluation suggested that HMME-PDT did not affect the inflammatory microenvironment of the keloid. Despite this, the inhibitory effect on keloid fibroblast proliferation in lieu of inflammation stimulation by PDT was ultimately beneficial for the treatment of the keloid.
Collectively, the evidence suggests that HMME-PDT inhibits the growth of keloid grafts through the promotion of fibroblast apoptosis and the suppression of adjacent vessel formation. Though the molecular signals of HMME-PDT require further study, the therapeutic potential of HMME-PDT was demonstrated in this study via both in vitro and in vivo disease models of keloid formation.
Conflicts of interest
There are no conflicts of interest to declare.
Acknowledgements
We thank Anne M. O'Rourke, PhD, from Liwen Bianji, Edanz Group China for English-language editing of this manuscript and Shanghai Fudan-Zhangjiang Bio-Pharmaceutical Co., Ltd for drug delivery and technical support.
References
- G. M. Bran, U. R. Goessler, K. Hormann, F. Riedel and H. Sadick, Keloids: current concepts of pathogenesis (review), Int. J. Mol. Med., 2009, 24, 283–293 CrossRef CAS PubMed.
- O. J. Smith and D. A. McGrouther, The natural history and spontaneous resolution of keloid scars, J. Plast. Reconstr. Aesthet. Surg., 2014, 67, 87–92 CrossRef PubMed.
- D. Wolfram, A. Tzankov, P. Pulzl and H. Piza-Katzer, Hypertrophic scars and keloids-a review of their pathophysiology, risk factors, and therapeutic management, Dermatol. Surg., 2009, 35, 171–181 CrossRef CAS PubMed.
- J. P. Celli, B. Q. Spring, I. Rizvi, C. L. Evans, K. S. Samkoe, S. Verma and B. W. Pogue, Imaging and photodynamic therapy: mechanisms, monitoring, and optimization, Chem. Rev., 2010, 110, 2795–2838 CrossRef CAS PubMed.
- G. G. Gauglitz, H. C. Korting, T. Pavicic, T. Ruzicka and M. G. Jeschke, Hypertrophic scarring and keloids: pathomechanisms and current and emerging treatment strategies, Mol. Med., 2011, 17, 113–125 CAS.
- L. Y. Kerwin, A. K. El Tal, M. A. Stiff and T. M. Fakhouri, Scar prevention and remodeling: a review of the medical, surgical, topical and light treatment approaches, Int. J. Dermatol., 2014, 53, 922–936 CrossRef PubMed.
- S. Monstrey, E. Middelkoop, J. J. Vranckx, F. Bassetto, U. E. Ziegler, S. Meaume and L. Teot, Updated scar management practical guidelines: non-invasive and invasive measures, J. Plast. Reconstr. Aesthet. Surg., 2014, 67, 1017–1025 CrossRef.
- F. B. Rabello, C. D. Souza and J. A. Farina Junior, Update on hypertrophic scar treatment, Clinics, 2014, 69, 565–573 CrossRef.
- B. F. Seo and S. N. Jung, The immunomodulatory effects of mesenchymal stem cells in prevention or treatment of excessive scars, Stem Cells Int., 2016, 2016, 6937976 Search PubMed.
- S. Al-Mohamady Ael, S. M. Ibrahim and M. M. Muhammad, Pulsed dye laser versus long-pulsed Nd:YAG laser in the treatment of hypertrophic scars and keloid: A comparative randomized split-scar trial, J. Cosmet. Laser Ther., 2016, 18, 208–212 CrossRef PubMed.
- J. Akimoto, Photodynamic therapy for glioblastoma, Gan To Kagaku Ryoho, 2015, 42, 678–682 Search PubMed.
- E. Christensen, T. Warloe, S. Kroon, J. Funk, P. Helsing, A. M. Soler and H. J. Stang, Guidelines for practical use of MAL-PDT in non-melanoma skin cance, J. Eur. Acad. Dermatol. Venereol., 2010, 24, 505–512 CrossRef CAS PubMed.
- D. H. Ghodasra and H. Demirci, Photodynamic therapy for choroidal metastasis, Am. J. Ophthalmol., 2016, 161, 104–109 CrossRef PubMed.
- R. Jayadevappa and S. Chhatre, Survival and cost among photodynamic therapy patients with non-small cell lung cancer, Value Health, 2015, 18, A437 CrossRef.
- C. Lavogiez, L. Mortier and S. Mordon, Antimicrobial photodynamic therapy in dermatology. Part I: Bacterial and fungal infections, Ann. Dermatol. Venereol., 2015, 142, 782–788 CrossRef CAS PubMed.
- M. Ramirez, S. Groff and C. Kowalewski, Eruptive keratoacanthomas after photodynamic therapy, Dermatol. Surg., 2015, 41, 1426–1429 CrossRef CAS PubMed.
- T. C. Lei, G. F. Glazner, M. Duffy, L. Scherrer, S. Pendyala, B. Li and X. Wang, Optical properties of hematoporphyrin monomethyl ether (HMME), a PDT photosensitizer, Photodiagn. Photodyn. Ther., 2012, 9, 232–242 CrossRef CAS PubMed.
- H. Cai, Y. Gu, Q. Sun, J. Zeng, N. Dong and G. Zhao, Effect of hematoporphyrin monomethyl ether-mediated photodynamic therapy on hypertrophic scar fibroblasts, Photodermatol., Photoimmunol. Photomed., 2011, 27, 90–96 CrossRef CAS PubMed.
- L. L. Chiu, C. H. Sun, A. T. Yeh, B. Torkian, A. Karamzadeh, B. Tromberg and B. J. Wong, Photodynamic therapy on keloid fibroblasts in tissue-engineered keratinocyte-fibroblast co-culture, Lasers Surg. Med., 2005, 37, 231–244 CrossRef PubMed.
- J. P. Golding, T. Wardhaugh, L. Patrick, M. Turner, J. B. Phillips, J. I. Bruce and S. G. Kimani, Targeting tumour energy metabolism potentiates the cytotoxicity of 5-aminolevulinic acid photodynamic therapy, Br. J. Cancer, 2013, 109, 976–982 CrossRef CAS.
- A. Giovannini, P. Neri, L. Mercanti and C. Brue, Photodynamic treatment versus photodynamic treatment associated with systemic steroids for idiopathic choroidal neovascularisation, Br. J. Ophthalmol., 2007, 91, 620–623 CrossRef PubMed.
- M. Chang, X. Ma, T. Ouyang, J. Lin, J. Liu, Y. Xiao and H. Chen, Potential molecular mechanisms involved in 5-aminolevulinic acid-based photodynamic therapy against human hypertrophic scars, Plast. Reconstr. Surg., 2015, 136, 715–727 CrossRef CAS PubMed.
- X. Lai, F. Ning, X. Xia, D. Wang, L. Tang, J. Hu and J. Wu, HMME combined with green light-emitting diode irradiation results in efficient apoptosis on human tongue squamous cell carcinoma, Lasers Med. Sci., 2015, 30, 1941–1948 CrossRef.
- L. Liu, Y. Song, L. Ma, L. Zang, L. Tao, Z. Zhang and J. Han, Growth inhibition effect of HMME-mediated PDT on hepatocellular carcinoma HepG2 cells, Lasers Med. Sci., 2014, 29, 1715–1722 CrossRef.
- K. Song, J. Li, L. Li, P. Zhang, F. Geng, R. Dong and Q. Yang, Intracellular metabolism, subcellular localization and phototoxicity of HMME/HB in ovarian cancer cells, Anticancer Res., 2011, 31, 3229–3235 CAS.
- B. Li, Z. Chen, L. Liu, Z. Huang, Z. Huang and S. Xie, Differences in sensitivity to HMME-mediated photodynamic therapy between EBV+C666-1 and EBV-CNE2 cells, Photodiagn. Photodyn. Ther., 2010, 7, 204–209 CrossRef PubMed.
- H. T. Li, X. Y. Song, C. Yang, Q. Li, D. Tang, W. R. Tian and Y. Liu, Effect of hematoporphyrin monomethyl ether-mediated PDT on the mitochondria of canine breast cancer cells, Photodiagn. Photodyn. Ther., 2013, 10, 414–421 CrossRef CAS.
- J. Cheng, H. Liang, Q. Li and W. Cao, Hematoporphyrin monomethyl ether-mediated photodynamic effects on THP-1 cell-derived macrophages, J. Photochem. Photobiol., B, 2010, 101, 9–15 CrossRef CAS.
- J. Shi, R. Ma, L. Wang and Z. Zhang, The application of hyaluronic acid-derivatized carbon nanotubes in hematoporphyrin monomethyl ether-based photodynamic therapy for in vivo and in vitro cancer treatment, Int. J. Nanomed., 2013, 8, 2361–2373 CrossRef.
- Y. Zhao, Z. Zhou, G. Zhou, P. Tu, Q. Zheng, J. Tao, Y. Gu and X. Zhu, Efficacy and safety of hemoporfin in photodynamic therapy for port-wine stain: a multicenter and open-labeled phase IIa study, Photodermatol., Photoimmunol. Photomed., 2011, 27, 17–23 CrossRef CAS.
- Y. Zhao, P. Tu, G. Zhou, Z. Zhou, X. Lin, H. Yang, Z. Lu, T. Gao, Y. Tu, H. Xie, Q. Zheng, Y. Gu, J. Tao and X. Zhu, Hemoporfin Photodynamic Therapy for Port-Wine Stain: A RandomizedControlled Trial, PLoS One, 2016, 11, e0156219 CrossRef.
- J. Jagadeesan and A. Bayat, Transforming growth factor beta (TGF beta) and keloid disease, Int. J. Surg., 2007, 5, 278–285 CrossRef PubMed.
- R. Derynck and Y. E. Zhang, Smad-dependent and Smad-independent pathways in TGF-beta family signalling, Nature, 2003, 425, 577–584 CrossRef CAS.
- M. Sato, Upregulation of the Wnt/beta-catenin pathway induced by transforming growth factor-beta in
hypertrophic scars and keloids, Acta Derm.-Venereol., 2006, 86, 300–307 CrossRef CAS.
- V. Nesi-Reis, D. S. S. L. Lera-Nonose, J. Oyama, M. P. P. Silva-Lalucci, I. G. Demarchi, S. M. A. Aristides, J. J. V. Teixeira, T. G. V. Silveira and M. V. C. Lonardoni, Contribution of photodynamic therapy in wound healing: A systematic review, Photodiagn. Photodyn. Ther., 2017, 21, 294–305 CrossRef.
- P. Agostinis, K. Berg, K. A. Cengel, T. H. Foster, A. W. Girotti, S. O. Gollnick, S. M. Hahn, M. R. Hamblin, A. Juzeniene, D. Kessel, M. Koberlik, J. Moan, P. Mroz, D. Nowis, J. Piette, B. C. Wilson and J. Golab, Photodynamic Therapy of cancer: an update, Ca-Cancer J. Clin., 2011, 61, 250–281 CrossRef PubMed.
- C. M. Brackett and S. O. Gollnick, Photodynamic therapy enhancement of anti-tumor immunity, Photochem. Photobiol. Sci., 2011, 10, 649–652 RSC.
- S. O. Gollnick, Photodynamic therapy and antitumor immunity, J. Natl. Compr. Cancer Network, 2012, 10, S40–S43 CAS.
- B. Wang, X. Gong, J. Y. Wan, L. Zhang, Z. Zhang and H. Z. Li, Resolvin D1 protects mice from LPS-induced acute lung injury, Pulm. Pharmacol. Ther., 2011, 24, 434–441 CrossRef CAS PubMed.
- J. Mendoza-Garcia, A. Sebastian, T. Alonso-Rasgado and A. Bayat, Ex vivo evaluation of the effect of photodynamic therapy on skin scars and striae distensae, Photodermatol., Photoimmunol. Photomed., 2015, 31, 239–251 CrossRef CAS PubMed.
Footnote |
† These authors contributed equally to this work. |
|
This journal is © The Royal Society of Chemistry and Owner Societies 2020 |
Click here to see how this site uses Cookies. View our privacy policy here.