Implications of dichlorofluorescein photoinstability for detection of UVA-induced oxidative stress in fibroblasts and keratinocyte cells
Received
15th October 2019
, Accepted 19th November 2019
First published on 21st November 2019
Abstract
Although the dichlorofluorescein (DCF) assay is widely used to detect the production of UVA-induced ROS, the photostability and phototoxicity of the probe after UVA irradiation remains controversial and the experimental conditions often vary across studies, making it difficult to compare results from different studies. This study aimed to evaluate the suitability of the DCF assay for detection of UVA-induced ROS in human cells after UVA irradiation. Human primary fibroblasts (HPF) and HaCaT cells were loaded with 2′,7′-dichlorodihydrofluorescein diacetate (DCFDA) (2, 10, and 50 μM) for 10 and 30 min, before and after exposure to UVA radiation (5–50 J cm−2). Fluorescence was recorded immediately or 30 min after irradiation using three different techniques: microplate reading, flow cytometry, and confocal scanning microscopy. Cell viability was assessed by flow cytometry before and after UVA exposure. A UVA-dose-dependent increase in ROS was observed at 5–50 μM DCFDA, and the magnitude of the fluorescent signal was affected by RPMI medium, as well as DCFDA loading concentration and incubation period. However, higher concentrations of DCFDA compromised the viability of both HaCaT and HPF cells after UVA irradiation. The most sensitive and reliable combination for the ROS assay was pre-incubation with 10 μM DCFDA for 30 min in PBS. Reading the fluorescence 30 min after UVA irradiation diminished the emission signal, as did the DCFDA post-incubation. In conclusion, this single-point DCF assay allowed reproducible and sensitive UVA-induced ROS detection in HaCaT and HPF cells without compromising the cell viability or morphology.
1. Introduction
UVA is thought to cause cell damage by inducing the production of reactive oxygen species (ROS), highly reactive molecules generated by the reduction of oxygen and possessing one or more unpaired electrons (e.g. singlet oxygen, the superoxide and hydroxyl radicals).1,2 These molecules can cause the oxidation of cell proteins, DNA, lipids, and other biomolecules, leading to aberrant cell signaling, dysfunctional redox control, apoptosis, and cell death, which can result in carcinogenesis and skin aging.2–4 Therefore, investigating the ROS production in cells is one of the first and fundamental steps in elucidating the genotoxic effects of solar radiation on human skin.5–9
Different techniques are available to assess the cellular oxidant levels and ROS, including electrochemical quantification, electro paramagnetic resonance (EPR) spectroscopy, and fluorescent signaling.10–12,35 But, 2′,7′-dichlorodihydrofluorescein diacetate (H2DCFDA or DCFDA) is the most widely used probe for measuring peroxyl, hydroxyl, and other ROS within cells. DCFDA is a cell-permeable molecule that is hydrolyzed to 2′,7′-dichlorodihydrofluorescein (H2DCF) carboxylate anion form in the cytosol by intracellular esterases, and then oxidized by intracellular ROS to form the fluorescent compound, dichlorofluorescein (DCF). Upon excitation at 495 nm, DCF emits fluorescence in the green range of the spectrum at 520 nm, at a magnitude proportional to the level of ROS production.10,12,13
Although the DCF probe has been extensively used in human cells to evaluate ROS production after UV radiation,6–9,14–16 its photo-oxidation upon exposure to UV radiation is the main concern about the DCF assay,17 especially as an indicator of oxidative stress. In addition, the possible effects of its photoinstability on cell viability and ROS production have not been explored in the literature,18,19 which may lead to misinterpretation of experimental data. It has been assumed that DCFDA does not affect cell metabolism or viability, but few studies have confirmed the absence of toxicity, especially in the context of keratinocytes and fibroblasts.10,20 To our knowledge, this is the first study to report DCF toxicity on both fibroblasts and keratinocytes after UVA radiation.10,12,20,21 Another difficulty is that the experimental conditions of DCFDA assay also vary across studies, making it difficult to compare results from different studies. For instance, the slope of the ROS dose–response curves differs greatly depending on the DCFDA concentration in the cell culture media, which can range from 5 to 50 μM, for 15 min to 1 h, before or after the cells are exposed to UV light.3,5–9,18
Considering that the DCF assay is very easy to use, inexpensive, and extremely sensitive to changes in the redox state of a cell,12,22 it is important to better understand how the systems behaves upon UVA radiation for proper interpretation of the data obtained using the DCF assay. Therefore, this study aimed to evaluate the suitability of the DCF assay for monitoring UVA-induced oxidative stress in human keratinocytes and fibroblasts using various assay techniques such as microplate reading, flow cytometry, and confocal microscopy. Some issues related to the DCF assay, such as incubation period, DCFDA concentration, and viability of cells post-UVA irradiation incubation in DCFDA, are also discussed.
2. Materials and methods
2.1. Chemicals, media, buffers, and reagents
Dulbecco's modified Eagle medium (DMEM), 0.5% trypsin-0.1 mM EDTA solution (trypsin-EDTA), penicillin/streptomycin, fetal bovine serum (FBS), non-essential amino acids solution (100×), and the H2DCFDA substrate were purchased from Life Technologies/Gibco (New York, USA). The other chemicals and reagents used in this study were purchased from Sigma Chemical Company (St Louis, MO), unless specified otherwise. Phosphate-buffered saline (PBS 0.1 M, pH 7.2) was prepared in deionized water containing 2.25 mM KCl, 100 mM NaCl, 0.75 mM Na2HPO4, and sterile-filtered using 0.22 μm pore membrane before use. A 100 mM DCFDA stock solution was prepared in dimethyl sulfoxide (DMSO), stored in frozen form at −80 °C, and diluted in buffers or medium immediately before use.
2.2. Cells and cell culture
This study was performed in strict accordance with ethical guidelines of the 1975 Declaration of Helsinki, revised in 2000, for the ethics of research involving human subjects and was approved by the Human Experimentation Committee of the FFCLRP at University of São Paulo (CAAE no. 76759717.7.0000.5407, Brazil). Human primary fibroblasts (HPF) were isolated from foreskins of 3–8 years old children after approval by the institution Ethics Committee (CAAE no. 76759717.7.0000.5407) and maintained in DMEM supplemented with 10% FBS, 100 U mL−1 penicillin, and 50 μg mL−1 streptomycin. HaCaT, a transformed epidermal human cell line,23 was cultured in DMEM supplemented with 10% FBS, 100 U mL−1 penicillin, 50 μg mL−1 streptomycin, and 0.1 mM non-essential amino acids. Cell cultures were maintained in a 5% CO2 atmosphere at 37 °C, and sub-cultured following trypsin-EDTA treatment after they had reached confluence.
2.3. UVA radiation source
Cells were irradiated at 5–50 J cm−2 using an improvised system equipped with two UVA fluorescent lamps (DULUX L BL UVA 36 W/78) with an emission spectrum between 320 and 460 nm, corresponding to 86.1% UVA and 13.9% visible light. The cells were irradiated at a distance of 17.5 cm from the source and received an irradiance of 7.39 ± 0.79 mW cm−2, measured with an Ocean Optics spectrophotometer (USB 2000 +UV–VIS–ES) guided by Ocean Optics optical fiber (QP50-2-UV–VIS).
2.4. DCF assay optimization for oxidative stress in HaCaT cells
In the present study, the DCF assay conditions were optimized and carried out using HaCaT cell culture under different experimental conditions. Under the first experimental condition, the cells were seeded in 24-well plates (Corning Costar, Corning, NY) at an initial density of 50
000 cells per well. After 24 h, the confluent cells were irradiated in RPMI medium (without phenol red), added with 10 μM DCFDA in RPMI medium, and incubated at 37 °C under a 5% CO2 atmosphere for 10 min. Under the second experimental condition, the confluent HaCaT cells were first washed twice and irradiated in pre-warmed PBS (containing 1 mM non-essential amino acids), and then added with 2, 10, or 50 μM DCFDA in PBS and incubated at 37 °C under a 5% CO2 atmosphere for 10 and 30 min. Under the third experimental condition, the confluent cells were first washed twice in pre-warmed PBS and pre-incubated at 37 °C with 2, 10, or 50 μM DCFDA in PBS under a 5% CO2 atmosphere for 30 min. At the end of the incubation period, the cells were again washed twice with PBS and then irradiated. Control and irradiated samples were subjected to the same experimental conditions and non-irradiated cells were kept at room temperature within the laminar flow during UVA exposure time.
The Relative Fluorescent Unit (RFU) was recorded with the cells in PBS, immediately and 30 min after irradiation, using a Cytation 5 fluorescence plate reader (Biotek Instruments Inc., Vermont, EUA) at excitation and emission wavelengths of 485 and 528 nm, respectively. To minimize the possible photo-oxidation of the probe and/or the photo-reduction of DCF, the plates were covered with aluminum foil to shield the probe from ambient light during incubation.
The background levels of DCFH oxidation in RPMI and PBS were determined by diluting DCFDA to the final concentration of 10 μM or 50 μM in RPMI and PBS, and monitoring the change in fluorescence after 30 min of incubation.
After assay optimization, five independent experiments were performed in order to evaluate the linear range of the method. Cells were exposed to 5, 15, 30, 40 and 50 J cm−2 and control (non-irradiated cells) were kept at room temperature within the laminar flow during this time.
2.5. Phototoxicity evaluation
The DCFDA cytotoxicity was evaluated in HaCaT and HPF cells before and after UVA exposure. After 30 min of incubation in 10 and 50 μM DCFDA in PBS, cells were exposed to UVA doses of 10 and 30 J cm−2.14,18 Non-irradiated cells were used as controls. Immediately after irradiation, the cells were harvested by trypsinization and centrifuged at 106g at 4 °C for 5 min. The cell pellet was resuspended in the ViaCount® reagent (Guava ViaCount® Assay Kit, Merck Millipore, UK) and incubated in the dark for 20 min at room temperature (∼23 °C). After staining, viability data were acquired using the Guava 8HT (GuavaTechnologies, Hayward, CA, USA) flow cytometer in the yellow channel with 5000 events per sample.22 Experiments were conducted in triplicate and data were analyzed using the in-built GuavaSoft™ ViaCount 4.2.1 software.
Cell morphology was examined using an inverted light microscope (Axiovert 40 CFL, Carl Zeiss, Göttingen, Germany) and an AxioVision LE 40 v 4.8.2.0 (Carl Zeiss, Göttingen, Germany) system. Three digital images of each well were acquired with a 20× (0.40 php) objective using an image acquisition system, Axiocam MRC (Carl Zeiss, Göttingen, Germany).
2.6. DCF assay in keratinocyte and fibroblast cell cultures
For the routine DCF assay, after optimization of the method, HaCaT and HPF cells were seeded in 24-well plates (Corning Costar, Corning, NY) at a density of 50
000 cells per well. After 24 h, the cells were washed twice with pre-warmed PBS and then incubated at 37 °C for 30 min with 10 μM DCFDA in PBS under a 5% CO2 atmosphere in the dark. At the end of the incubation period, the cells were again washed twice with PBS and irradiated at 10, 30, and 50 J cm−2, while they were in 300 μL of PBS per well. Immediately after the radiation exposure, the plates were read with a Cytation 5 fluorescence plate reader (Biotek Instruments Inc., Vermont, EUA) using the following settings: reading mode: bottom, multiple reads per well type: FilledSquare, multiple reads per well size: 5 × 5, excitation: 485 nm, and emission: 528 nm.
A control (DCFDA-loaded and non-irradiated cells), negative control (non-DCFDA-loaded and non-irradiated cells), and a positive control (DCFDA-loaded and non-irradiated cells, treated with 100 μM H2O2) were subjected to the same procedures. The positive control was quantified after incubation with H2O2 (100 μM in PBS) for 30 min at 37 °C and 5% CO2 atmosphere in the dark.
2.7. DCF assay by flow cytometry
Alternatively, immediately after reading of the plates, the cells irradiated at 10 and 50 J cm−2 UVA were collected by trypsinization and centrifuged at 106g at 4 °C for 5 min. The pellets were washed and resuspended in PBS for analysis by flow cytometry. The DCF fluorescence was measured in the green channel in a Guava EasyCyte 8HT (GuavaTechnologies, Hayward, CA, USA) flow cytometer. The data were collected and analyzed using the Guava CytoSoft 4.2.1 Software Environment (GuavaTechnologies, Hayward, CA, USA).24
2.8. DCF assay by confocal microscopy
For the simultaneous visualization of the oxidative stress induced by UVA radiation, HPF and HaCaT cells were seeded in glass bottom plates at a density of 20
000 cells per plate. After reaching confluence, the cells were treated and irradiated at 10 and 50 J cm−2 UVA doses following the routine DCF assay protocol described above. Immediately after radiation exposure, the fluorescence of irradiated cells and negative controls was visualized using a confocal laser scanning microscope (Leica Platform TCS SP8, Leica Microsystems, Wetzlar, Germany).16,25
2.9. Statistical analysis
Linear regression analyses were used to determine the relationship between the UVA dose and the fluorescent signal in the dose–response curve. The mean RFU value at each UVA dose was compared with that of the control (non-irradiated) using a mixed-model analysis of variance (ANOVA). Bonferroni, Holm-Sidak, and Tukey post-hoc adjustments for multiple comparisons were used to investigate statistical significance. All statistical analyses were performed using Prism version 7.0a statistical software (GraphPad Software, San Diego, CA). All experiments were performed with three independent replicates, and all data are expressed as mean ± standard deviation.
3. Results
3.1. DCF assay optimization for oxidative stress in HaCaT cells
When HaCaT cells were irradiated at 10 and 30 J cm−2 and then incubated with 10 μM DCFDA in RPMI (experimental condition 1), no significant difference was observed in their fluorescence emission level compared with that in the sham-irradiated cells (Fig. 1). Replacing the RPMI medium with PBS during incubation and irradiation of cells (experimental condition 2) significantly reduced the fluorescent signal of both treated and untreated cells, but it improved the sensitivity of the method (Fig. 2). A high background signal was observed for 10 μM DCFDA in RPMI after 30 min of incubation in a cell-free system (16
681 ± 1165 RFU). While the background signal in PBS was minimal and the mean RFU values were 477 ± 11 and 674 ± 25 for 10 μM and 50 μM DCFDA, respectively. In addition, the RFU signal was dependent on the loading concentration and DCFDA incubation period. Incubation for 30 min and DCFDA concentration of at least 10 μM was needed to obtain a significant difference in ROS detection between irradiated and non-irradiated cells (Fig. 2).
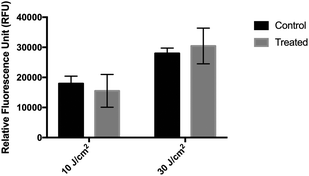 |
| Fig. 1 ROS generation by HaCaT cells loaded with 10 μm 2′,7′-dichlorofluorescein diacetate (DCFDA) in RPMI medium after irradiation at different UVA doses (10 and 30 J cm−2). Means ± SD from three independent experiments are shown. Data were subjected to two-way ANOVA with Bonferroni's post-hoc test. Control: non-irradiated cells. | |
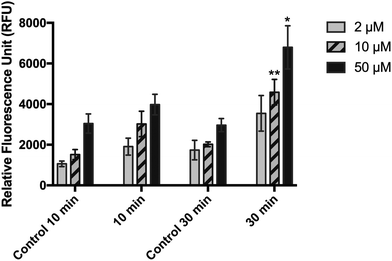 |
| Fig. 2 Effects of 2′,7′-dichloroflurescin diacetate (DCFDA) loading (2, 10, and 50 μm) and incubation period (10 or 30 min) on the detection of UVA-induced ROS in HaCaT cells. DCFDA was incubated in PBS after irradiation at 30 J cm−2 UVA. Means ± SD from three independent experiments are shown. Data were subjected to two-way ANOVA with Bonferroni's post-hoc test. *p ≤ 0.05; **p ≤ 0.01 compared with the respective control. Control: non-irradiated cells. | |
When cells were pre-incubated with DCFDA for 30 min (experimental condition 3), followed by washing, irradiation, and immediate measurement of fluorescence signal, the sensitivity of the method was significantly improved compared with that of the post-incubation method (Fig. 3A). Similarly, a significant difference in RFU values between irradiated and non-irradiated cells could be obtained only at 10 and 50 μM DCFDA. At these concentrations, the RFU was dose-dependent and showed a linear behavior (R2 = 0.969 and R2 = 0.961, respectively) against the UVA dose (5–50 J cm−2), ranging from 2736 ± 459 and 6776 ± 20 to 81
280 ± 5466 and 145
276 ± 5348 (Fig. 3B). We could not observe this behavior at 2 μM DCFDA. Importantly, when the RFU was recorded 30 min after the end of irradiation, there was a reduction in the RFU values compared with the case when RFU was recorded immediately after irradiation, although this was not statistically significant (data not shown).
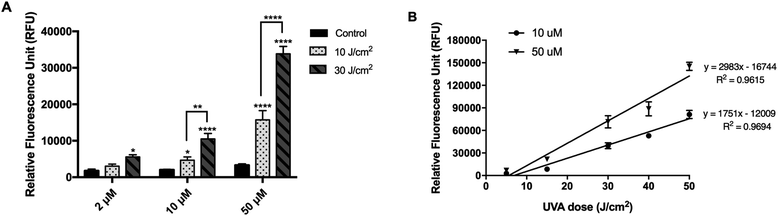 |
| Fig. 3 Effects of 2′,7′-dichloroflurescin diacetate (DCFDA) pre-loading (2, 10, and 50 μm) on the detection of UVA-induced ROS in HaCaT cells (a) and dose–response curve at 10 and 50 μm DCFDA (b). DCFDA was incubated in PBS for 30 min before UVA irradiation. Means ± SD from three (a) or five (b) independent experiments are shown. Data were subjected to two-way ANOVA with Bonferroni's post-hoc test. *p ≤ 0.05; **p ≤ 0.01; ****p ≤ 0.0001 compared with the control. Control: non-irradiated cells. | |
3.2. Phototoxicity assay
When the cells were loaded with 10 or 50 μM DCFDA, there was no significant effect on cell viability or morphology (Fig. 4 and 5). However, exposing the cells to the combination of 50 μM DCFDA and sub-lethal UVA doses (10 and 30 J cm−2) seemed to prove more toxic to cells, and the cell viability showed a significant reduction compared with the control (non-irradiated cells). This behavior was observed for both HaCaT and HPF cell lines.
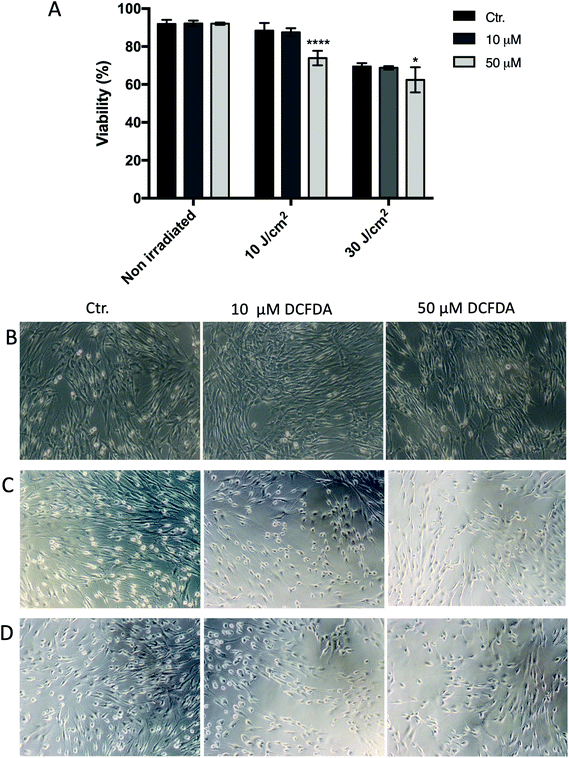 |
| Fig. 4 Effect of DCFDA on HPF cell viability and morphology. Cells were loaded with 10 or 50 μm 2′,7′-dichlorofluorescein diacetate (DCFDA) for 30 min, and then washed and exposed to 10 and 30 J cm−2 UVA. Cell viability was assessed by flow cytometry (a). Cell morphology was examined using an inverted microscope; digital images were taken with Axiovert 40 CFL, Carl Zeiss acquisition system for (b) non-irradiated cells, (c) UVA 10 J cm−2 treatment group, and (d) UVA 30 J cm−2 treatment group. Means ± SD from three independent experiments are shown. Cell viability data were subjected to two-way ANOVA with Tukey's post-hoc test. *p ≤ 0.05; ****p ≤ 0.00005. HPF: human primary fibroblasts; control (Ctr.): non-DCFDA-loaded cells. | |
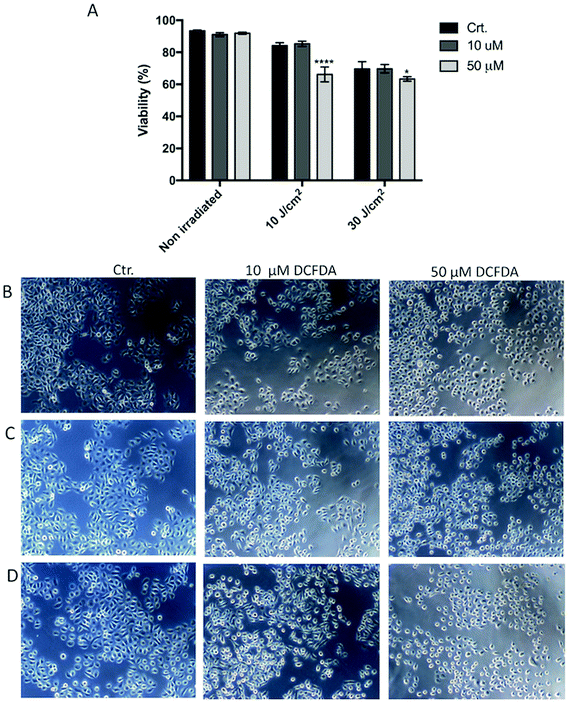 |
| Fig. 5 Effect of DCFDA on HaCaT cell viability and morphology. Cells were loaded with 10 or 50 μm 2′,7′-dichlorofluorescein diacetate (DCFDA) for 30 min, and then washed and exposed to 10 or 30 J cm−2 UVA. Cell viability was accessed by flow cytometry (a). Cell morphology was examined using an inverted microscope; digital images were taken with Axiovert 40 CFL, Carl Zeiss acquisition system for (b) non-irradiated cells, (c) UVA 10 J cm−2 treatment group, and (d) UVA 30 J cm−2 treatment group. Means ± SD from three independent experiments are shown. Cell viability data were subjected to two-way ANOVA with Tukey's post-hoc test. *p ≤ 0.05; ****p ≤ 0.00005. Control (Ctr.): non-DCFDA-loaded cells. | |
3.3. DCF assay in HaCaT and HPF cell cultures
The optimized assay was tested for measuring the oxidative stress induced by UVA radiation in HaCaT and HPF cell lines, and the results are presented in Fig. 6. Treatment with 100 μM H2O2 for 30 min was also performed after DCFDA loading and used as a positive control. As observed, the oxidative stress was UVA-dose dependent for both cell lines, as reported above, but its nature depended on the cell line. At the same UVA dose, HaCaT cells showed a higher fluorescence signal than HPF cells (Fig. 6A). These results were confirmed by the flow cytometry and confocal microscopy data. A clear redox imbalance was observed after analysis by flow cytometry in both HaCaT and HPF cells at UVA doses of 10 and 50 J cm−2 (Fig. 6B and C). This dose-dependent behavior could also be visualized by confocal microscopy, and it was obtained similar results to flow cytometer. In summary, the standardized method showed a good sensitivity, indicating that it can be used for the evaluation of oxidative stress in HaCaT and HPF cell lines induced by different doses of UVA radiation.
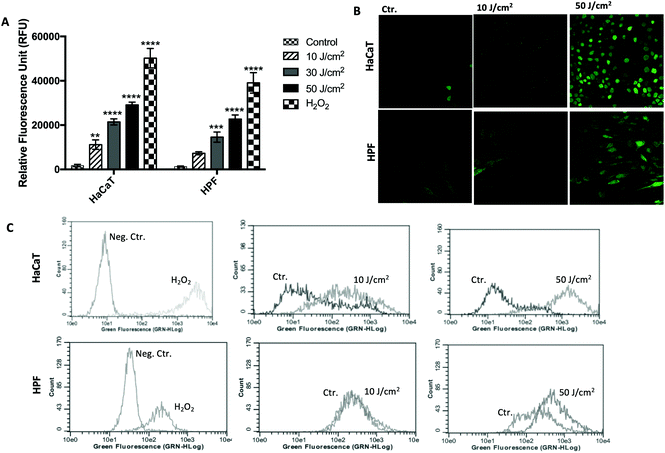 |
| Fig. 6 ROS production in HaCaT and HPF cells after pre-incubation with 10 μm DCFDA in PBS for 30 min and exposed to different UVA doses. The fluorescent signal was measured immediately after cell irradiation by microplate reader (a), confocal scanning microscopy (b) and flow cytometer (c). Means ± SD of 3 independent experiments shown. Data were subjected to one-way ANOVA with Holm-Sidak's post-hoc test. *p ≤ 0.05; **p ≤ 0.01; ***p ≤ 0.001; ****p ≤ 0.0001 compared with control. Ct: control (non-irradiated cells); nc: negative control; pc: positive control (100 μm H2O2); HPF: human primary fibroblasts. | |
4. Discussion
The aim of this study was to evaluate the suitability of DCF assay and discus the different parameters that can influence the results, in order to adopt it for measuring the oxidative stress induced by UVA radiation in cultured human cells. Considering the DCFDA photo-oxidation upon UV radiation, which is the main drawback of the assay, this study also discusses its effect on cell viability, morphology and ROS generation, in addition to establish the optimal assay conditions for routine assay in HaCaT and HPF cells.
The main difficulty in evaluating the UVA-induced ROS is that the ROS are present in low concentrations in the intracellular environmental, and most of the reactive oxygen species have a short lifespan.20,26,27 Although some previous studies have indicated that there is little to no difference between loading DCFDA into cells before rather than after UVA exposure,15,28 in this study, we obtained significant higher levels of RFU and a dose-dependent behavior from 5 to 50 J cm−2 when DCFDA was pre-incubated (Fig. 2 and 3), which improved the sensitivity of the method. In addition, real-time monitoring during the first 30 min after irradiation reduced the DCF fluorescent signal, suggesting that the UVA-induced DCFH oxidation was instantaneous, and it must be read immediately after the end of irradiation. In the post-incubation method, during the incubation period (10–30 min) needed to load DCFDA into cells, the UVA-induced ROS production may have exhibited an initial burst, which could not be adequately quantified. In addition, DCFDA needs to be converted to DCF by cellular esterases before the occurrence of ROS-induced DCF oxidation, as previously discussed. Similar results were found by Wan et al. (2003) and Alzal et al. (2003). It is reported that the increase in ROS begins during radiation exposure and continues for approximately 2–5 min before returning to baseline levels after irradiation.
This study demonstrates that the sensitivity and specificity of the DCF assay was also dependent on the concentration and incubation period of DCFDA. While this can help maximize the assay sensitivity and ROS detection, attention should be paid to the possibility of dye-induced cytotoxicity. Almost all previous studies have reported DCFDA incubation at concentrations ranging from 5 to 50 μM, for 15 min to 1 h.7,9,10,16,22,29 Although DCFDA is typically considered non-toxic to cells, previous studies reported the absence of cytotoxicity only in HaCaT cells until μM 20 DCFDA.18–20 In this study, 30 min of incubation was needed for significant detection of UVA-induced ROS, and the higher the DCFDA concentration (2–50 μM), the greater was the fluorescence signal, resulting in a precise ROS assay. As observed in Fig. 5 and 6, these concentrations had no effect on the viability of non-irradiated cells, which confirms the previous results with HaCaT cells. Interestingly, the medium for DCFDA loading may also affect the ROS detection. A high background signal was observed when RPMI was used for DCFDA incubation for both cell-containing and cell-free system (Fig. 1), suggesting a non-specific H2DCF oxidation when the probe is incubated in RPMI. RPMI and other commonly used culture media and buffers contain tyrosine, among other amino acids, that can oxidize H2DCF in a non-specific manner, which can be incorrectly interpreted as cellular ROS generation.5,20,30 In contrast, the background signal was reduced when PBS was used during DCFDA incubation, indicating that the non-specific H2DCF oxidation was minimal under this condition. The simple composition and lack of any metal ions such as Mg+2, Ca+2, and CO+2 in PBS help maintain a ‘neutral’ environment for the incubation of such probes, thereby alleviating the possibility of any exacerbation in fluorescence, as observed in RPMI due to the presence of such components.5,12,20,30
Although the DCF assay has been widely used to detect ROS induction by UV radiation, the controversy regarding the DCF assay is mainly around the photostability of DCFDA in UV-light-exposed systems.10,17,31 Some authors have reported that photochemical reactions may occur in cells containing DCF and/or H2DCF when they are irradiated, or that UV light may itself exhibit an effect on DCFDA that is independent of cellular ROS production.18,19 Considering that the pre-incubation of DCFDA generated greater quantification of ROS in this study, a further experiment was performed to account for any UVA–DCFDA interaction in exposed cells. This involved flow-cytometric analysis of cells double-labelled with DCFDA and the ViaCount® reagent to indicate any effects on cell death or lack of cell viability upon exposure to sub-lethal UVA doses, once cellular injury due to oxygen radicals may be mediated by the peroxidation of membrane lipids with consequent loss of cellular viability.13,22 As observed, no significant change in cell viability or morphology were observed at these concentrations in non-irradiated cells, but when the cells were irradiated to sub-lethal UVA doses, the viability decreased significantly in cells previously loaded with 50 μM DCFDA, compared with control (non-DCFDA-loaded cells), as shown in Fig. 4 and 5. These results suggest that there was an interaction between DCFDA and/or DCF and UVA light, which decreased cell viability after irradiation, once it was not impaired prior to UVA irradiation. This hypothesis was supported when a cell-free system containing 10 and 50 μM DCFDA in PBS was irradiated at 10 J cm−2 and a high fluorescent signal was recorded (7485 ± 838 and 128
057 ± 7202, respectively), thus, providing evidence that UVA increases the conversion of DCFDA to DCF in a cellular-ROS-independent manner.18,19,31
In this context, optimal assay conditions were achieved when cells were pre-loaded with 10 μM DCFDA and washed prior to UVA irradiation (at least twice with pre-warmed PBS), which yielded a significant and UVA dose-dependent increase in fluorescence signal in all three independent experiments, without a significant decrease in cell viability. Therefore, we strongly recommend washing of the cells prior to UVA irradiation, once the excess DCFDA probe is removed from the medium, thereby diminishing its photo-oxidation and preventing non-specific ROS generation.5 Therefore, if the cells are loaded before UV irradiation, adequate control conditions and sufficient care must be taken to account for the potential confounding effects reported in this study.
When the optimized method was used to compare the UVA-induced ROS between two cell lines (keratinocytes and fibroblasts) at different UVA doses, it was capable of detecting differences in ROS generation at low doses (10 J cm−2), with a very low background noise. It was also demonstrated that HaCaT cells generate more ROS after UVA irradiation than HPF cells regardless of the quantification method (microplate reader, flow-cytometry and confocal microscopy).24,32,33 As observed in Fig. 6(A and B), a clear redox imbalance was observed immediately after irradiation in both HaCaT and HPF cells at 10 and 50 J cm−2, along with a higher redox imbalance in HaCaT was also confirmed.34 Yohn et al. had previously demonstrated that human fibroblasts have higher antioxidant enzyme activity than keratinocytes, and therefore, are more resistant to oxidative stress, which supports these results and confirms the high sensitivity of the method. Simultaneously, we also used confocal laser microscopy for the comparative visualization of fluorescence from HaCaT and HPF cells treated with DCFDA. The results suggest higher oxidative stress at higher UVA doses, but the reproducibility was not as good as in the case of plate readings and flow cytometry. Importantly, when a confocal laser scanning microscope is used to quantify DCF fluorescence, the intensity of the excitation light needs to be as low as possible to minimize photo-oxidation of the DCFDA.17,22 In addition, it is advisable to use it in combination with another technique, such as cytometry, especially for comparative studies in order to avoid misinterpretation of data. In contrast, flow cytometry is expected to be much more precise than cell imaging and automated fluorescence reading, because of the large number of cells analyzed (5000 events), and allowing researchers to overcome a major disadvantage of the plate reader method (no consideration of cell number).18,22,24,35
In summary, the data presented here indicate that despite DCF's photo-instability, it can be suitable for the DCF assay in UV-exposed systems. Pre-incubation with 10 μM DCFDA for 30 min in PBS was sufficient to generate a sensitive and reproducible standard curve for detection of UVA-induced ROS in HaCaT and HPF cells, with no effects on cell viability or morphology. The finding that the DCF fluorescent signal must be measured immediately after UVA irradiation and that cells must be washed at least twice prior to UVA exposure should help obtain precise estimations and potentially increase the utility of the DCF assay for future research applications of UV-exposed cells. Finally, our data emphasize the suitability of DCFH as a probe to detect the oxidizing species in UVA-exposed cells, despite its photo-instability upon UVA radiation, but we highlight that adequate control conditions must be considered to overcome the potential limitations discussed in this study and proper interpretation of data.
Conflicts of interest
The authors report no conflicts of interest.
Acknowledgements
A. C. T. thanks the Brazilian Federal Agency for Support and Evaluation of Graduate Education (CAPES), State of São Paulo Research Foundation (FAPESP) (#2013/50181-1), FINEP (#01.10.0758.01) and the National Council for Scientific and Technological Development (CNPq) (PRONON-SIPAR project #25000.077093/2015-86). C. S. thanks FAPESP for her postdoctoral fellowship (#2017/01272-5). D. A. M. thanks CNPq for her Masters scholarship (#130731/2019-6). We also thank the National Institute of Science and Technology (INCT) for financial support through Nanobiotechnology project 573880/2008-5.
References
- P. Karran and R. Brem, DNA Repair, 2016, 44, 178–185 CrossRef CAS PubMed.
- J. Cadet, T. Douki and J.-L. Ravanat, Photochem. Photobiol., 2015, 91, 140–155 CrossRef CAS PubMed.
- R. Brem, M. Guven and P. Karran, Free Radicals Biol. Med., 2017, 107, 101–109 CrossRef CAS PubMed.
- C. Souza and P. M. B. G. M. Campos, Eur. J. Pharm. Sci., 2017, 104, 52–64 CrossRef CAS PubMed.
- X. S. Wan, Z. Zhou and A. R. Kennedy, Radiat. Res., 2003, 160, 622–630 CrossRef CAS PubMed.
- E. Metral, W. Rachidi, O. Damour, F. Demarne and N. Bechetoille, Photochem. Photobiol., 2018, 94, 343–350 CrossRef CAS PubMed.
- A. K. Srivastav, S. F. Mujtaba, A. Dwivedi, S. K. Amar, S. Goyal, A. Verma, H. N. Kushwaha, R. K. Chaturvedi and R. S. Ray, J. Photochem. Photobiol., B, 2016, 156, 87–99 CrossRef CAS PubMed.
- J.-K. Lee, S.-H. Ko, S.-K. Ye and M.-H. Chung, J. Dermatol. Sci., 2013, 70, 49–57 CrossRef CAS PubMed.
- B. Cortat, C. C. M. Garcia, A. Quinet, A. P. Schuch, K. M. de Lima-Bessa and C. F. M. Menck, Photochem. Photobiol. Sci., 2013, 12, 1483–1495 RSC.
- B. Kalyanaraman, V. Darley-Usmar, K. J. A. Davies, P. A. Dennery, H. J. Forman, M. B. Grisham, G. E. Mann, K. Moore, L. J. Roberts and H. Ischiropoulos, Free Radicals Biol. Med., 2012, 52, 1–6 CrossRef CAS PubMed.
- C. Souza, P. Maia Campos, S. Schanzer, S. Albrecht, S. B. Lohan, J. Lademann, M. E. Darvin and M. C. Meinke, Skin Pharmacol. Physiol., 2017, 30, 81–89 CrossRef CAS PubMed.
- X. Chen, Z. Zhong, Z. Xu, L. Chen and Y. Wang, Free Radical Res., 2010, 44, 587–604 CrossRef CAS PubMed.
- J. A. Scott, C. J. Homcy, B.-A. Khaw and C. A. Rabito, Free Radicals Biol. Med., 1988, 4, 79–83 CrossRef CAS PubMed.
- R. W. Redmond, A. Rajadurai, D. Udayakumar, E. V. Sviderskaya and H. Tsao, J. Invest. Dermatol., 2014, 134, 1083–1090 CrossRef CAS PubMed.
- H. J. Jee, H.-J. Kim, A. J. Kim, Y.-S. Bae, S. S. Bae and J. Yun, Biochem. Biophys. Res. Commun., 2009, 383, 358–362 CrossRef CAS PubMed.
- S. F. Mujtaba, A. Dwivedi, N. Yadav, R. S. Ray and G. Singh, J. Hazard. Mater., 2013, 252–253, 258–271 CrossRef CAS PubMed.
- M. Afzal, S. Matsugo, M. Sasai, B. Xu, K. Aoyama and T. Takeuchi, Biochem. Biophys. Res. Commun., 2003, 304, 619–624 CrossRef CAS PubMed.
- S. Boulton, A. Anderson, H. Swalwell, J. R. Henderson, P. Manning and M. A. Birch-Machin, Free Radical Res., 2011, 45, 115–122 CrossRef CAS PubMed.
- C. F. Chignell and R. H. Sik, Free Radicals Biol. Med., 2003, 34, 1029–1034 CrossRef CAS PubMed.
- D. Figueroa, M. Asaduzzaman and F. Young, J. Pharmacol. Toxicol. Methods, 2018, 94, 26–33 CrossRef CAS PubMed.
- C. C. Winterbourn, Biochim. Biophys. Acta, 2014, 1840, 730–738 CrossRef CAS PubMed.
- O. G. Lyublinskaya, J. S. Ivanova, N. A. Pugovkina, I. V. Kozhukharova, Z. V. Kovaleva, A. N. Shatrova, N. D. Aksenov, V. V. Zenin, Y. A. Kaulin, I. A. Gamaley and N. N. Nikolsky, Redox Biol., 2017, 12, 758–769 CrossRef CAS PubMed.
- N. E. Fusenig, D. Breitkreutz, R. T. Dzarlieva, P. Boukamp, A. Bohnert and W. Tilgen, J. Invest. Dermatol., 1983, 81, S168–S175 CrossRef PubMed.
-
E. Eruslanov and S. Kusmartsev, in Advanced Protocols in Oxidative Stress II, ed. D. Armstrong, Humana Press, Totowa, NJ, 2010, pp. 57–72 Search PubMed.
-
A. Wojtala, M. Bonora, D. Malinska, P. Pinton, J. Duszynski and M. R. Wieckowski, in Methods in Enzymology, ed. L. Galluzzi and G. Kroemer, Academic Press, 2014, vol. 542, pp. 243–262 Search PubMed.
- R. W. Redmond and I. E. Kochevar, Photobiology, 2006, 82, 1178–1187 CrossRef CAS PubMed.
- W. J. H. Koopman, L. G. J. Nijtmans, C. E. J. Dieteren, P. Roestenberg, F. Valsecchi, J. A. M. Smeitink and P. H. G. M. Willems, Antioxid. Redox Signaling, 2010, 12, 1431–1470 CrossRef CAS PubMed.
- F. Vanlente, Anal. Chem., 1991, 63, R199–R202 Search PubMed.
- C. Casale, G. Imparato, F. Urciuolo, F. Rescigno, S. Scamardella, M. Escolino and P. A. Netti, J. Tissue Eng. Regener. Med., 2018, 12, 1658–1669 CrossRef CAS PubMed.
- T. Ohashi, A. Mizutani, A. Murakami, S. Kojo, T. Ishii and S. Taketani, FEBS Lett., 2002, 511, 21–27 CrossRef CAS PubMed.
- P. Bilski, A. Belanger and C. Chignell, Free Radicals Biol. Med., 2002, 33, 938–946 CrossRef CAS PubMed.
- M. Oparka, J. Walczak, D. Malinska, L. M. P. E. van Oppen, J. Szczepanowska, W. J. H. Koopman and M. R. Wieckowski, Methods, 2016, 109, 3–11 CrossRef CAS PubMed.
- G. Rothe and G. Valet, J. Leukocyte Biol., 1990, 47, 440–448 CrossRef CAS PubMed.
- J. J. Yohn, D. A. Norris, D. G. Yrastorza, I. J. Buno, J. A. Leff, S. S. Hake and J. E. Repine, J. Invest. Dermatol., 1991, 97, 405–409 CrossRef CAS.
- S. Ahlberg, F. Rancan, M. Epple, K. Loza, D. Höppe, J. Lademann, A. Vogt, B. Kleuser, C. Gerecke and M. C. Meinke, Methods, 2016, 109, 55–63 CrossRef CAS.
|
This journal is © The Royal Society of Chemistry and Owner Societies 2020 |
Click here to see how this site uses Cookies. View our privacy policy here.