DOI:
10.1039/C9PY01694E
(Review Article)
Polym. Chem., 2020,
11, 1410-1423
Dynamic covalent bonds in self-healing, shape memory, and controllable stiffness hydrogels
Received
8th November 2019
, Accepted 24th January 2020
First published on 3rd February 2020
Abstract
Emerging technologies are increasingly reliant on materials that deliver multiple functionality. For example, hydrogels using dynamic covalent crosslinks have proven well-suited for the preparation of functional systems with properties including self-healing, shape memory, and stimuli-induced stiffness changes. These covalent bonds are capable of exchanging, dissociating or switching in response to various stimuli. In this review stimuli responsive dynamic covalent hydrogels are discussed on the basis of their chemistry and how the gels are increasingly used in novel applications. Challenges and potential future developments of dynamic covalent hydrogels are also discussed.
 M. Mario Perera | Mario Perera received his B.S from Institute of Chemistry, Ceylon, Sri Lanka where he worked on the modifying the formulations for natural rubber latex-based household gloves. He joined the group in January 2016. He is focused on preparing stimuli responsive hydrogels for biomaterials applications. |
 Neil Ayres | Dr. Neil Ayres received his Ph. D. training under Prof. David Haddleton at The University of Warwick, where his thesis was focused on surface-initiated atom transfer radical polymerization. After graduating in 2003 he worked as a post-doc for Prof. Charles McCormick at the University of Southern Mississippi, then Prof. William Brittain at the University of Akron, and also Prof. David Grainger at the University of Utah. He became an Assistant Professor in the Department of Chemistry at The University of Cincinnati in 2008, and was promoted to Associate Professor with Tenure in 2014. |
Introduction
Hydrogels are three-dimensional networks synthesized by crosslinking hydrophilic (macro)molecules which can absorb and retain a large amount of water.1 The term ‘hydrogel’ was first used in scientific literature in the 1890s when it was used to describe a colloidal gel of inorganic salts.2 In 1960 Wichterle and Lim were the first to define hydrogels as three dimensional, hydrophilic, crosslinked polymer structures in their article on poly(2-hydroxyethyl methacrylate) (PHEMA) gels for use as soft contact lenses.3 Hydrogels are now used in many different areas including biomaterials (for drug delivery,4,5 cell culture,6,7 biomedical implants8,9 and regenerative medicine,10,11 contact lenses,12,13 pharmaceuticals,14,15 cosmetics,16 adhesives,17 adsorbents,18,19 and sensors.20,21
Both synthetic and natural polymers have been used to prepare hydrogels,22,23 and the polymer chains can be crosslinked by a variety of chemical bonds or physical interactions.24,25 Naturally occurring polymers including polysaccharides, such as hyaluronic acid,26 alginate,27 and heparin,28 and polypeptides, such as gelatin29 and collagen,30 are often used in hydrogels. These hydrogels are typically formed as physical gels. Physical gels are defined as networks held together by molecular entanglements, ionic interactions, hydrogen-bonding, hydrophobic interactions, and host–guest interactions.31,32 All these interactions are reversible, and can be disrupted by changes in physical properties such as pH, temperature, stress, or addition of a specific chemical stimuli.33 Synthetic hydrogels use various chemistries to crosslink synthetic hydrophilic molecules,34 and crosslinking results in hydrogels with relatively high mechanical strength.35 The most widely used crosslinking reactions include free radical polymerization, condensation reactions, or orthogonal coupling reactions such as thiol–ene/yne reactions or alkyne–azide reactions.36 Covalently crosslinked hydrogels are called chemical hydrogels, and cannot be dissolved in solvents unless covalent bonds are cleaved.31
Some hydrogels are prepared using dynamic bonds that can undergo structural changes in response to a given stimulus by reversibly forming and breaking under one set of conditions and under different conditions acting as permanent covalent bonds.37 These hydrogels are commonly described as “dynamic hydrogels”. For example, hydrogels with disulfide crosslinks can undergo reversible exchange reaction in response to chemical or biological stimuli resulting in changes in matrix stiffness.38–40 Hydrazones are stable under neutral and basic conditions but readily hydrolyze under acidic conditions and exchange in the presence of hydrazides, aldehydes or ketones.41,42 Imines, the less stable homologs of hydrazones, are obtained from amines and aldehydes or ketones. Boronic esters are less stable, they hydrolyze quite easily and exchange in the presence of vicinal diols and catechols.43 Collectively, these chemistries are attaining increasing attention to prepare dynamic hydrogels and the ability to change the physical properties of these gels have made dynamic hydrogels attractive candidates for a variety of applications.44 Dynamic hydrogels including neutral34 and ionic polymers,45 degradable polymers,46 proteins,47 and nanoparticles48 have been prepared that are responsive to stimuli such as temperature,49 pH,50 light,51 ligands, or biomolecules.52 Considering this, we have limited the of scope this review to dynamic hydrogels crosslinked via dynamic covalent bonds (Fig. 1).
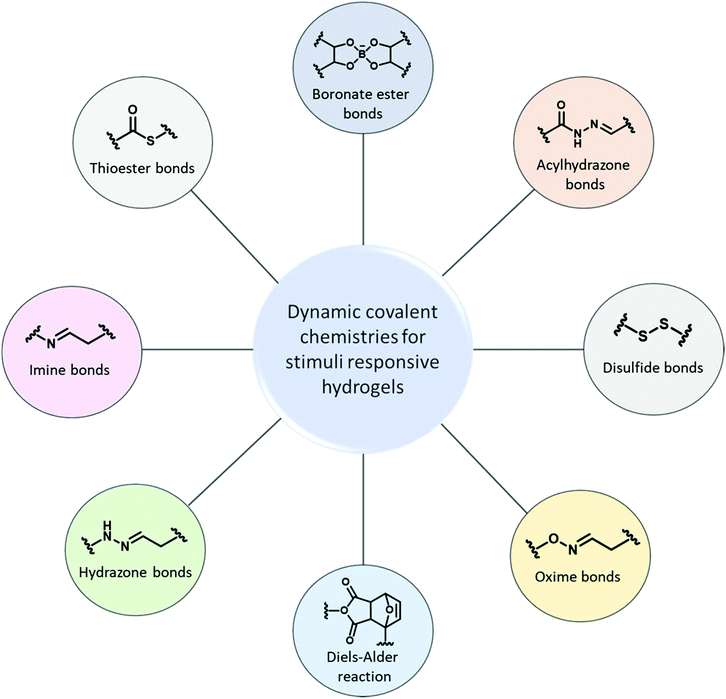 |
| Fig. 1 Dynamic covalent bonds used for preparation of stimuli responsive hydrogels. | |
We discuss how using the exchange reactions of these bonds under different stimuli give rise properties such as stimuli responsiveness,53,54 shape memory,55 and self-healing,56,57 and how these properties are increasingly used in novel applications.
Self-healing hydrogels
Synthetic materials capable of autonomous healing upon damage, defined as intrinsic and automatic healing,58,59 are being developed for potential applications including coatings,60 sealants,61 tissue adhesives,62 soft robotics,63 tissue engineering,64 and drug delivery.65 In the case of hydrogels, the most common types of damage are mechanical damage upon scratching, cracking, puncture, and delamination.66 Healing of a damaged site in a hydrogel can be achieved by number of different strategies including reversible dynamic covalent bonds,67,68 non-covalent interactions such as hydrogen bonding,69–71 supra-molecular host–guest interactions,72–74 electrostatic interactions,75,76 metal co-ordination,69,77 π–π stacking,78,79 hydrophobic arrangements,80–82 and molecular recognition.83 Specific functional groups are required in all these mechanisms to mediate self-healing in the damaged region.84
In this section we review hydrogels using dynamic covalent crosslinking chemistry to impart self-healing properties. Boronate complexation,85,86 disulfide bonds, Diels–Alder chemistry,87–89 oxime bonds,90 imine bonds,91 hydrazone bonds,92 and alkoxyamine bonds93 have all been used to prepare self-healing chemical hydrogels with or without various types of external stimuli (e.g. light, pH, or temperature).94 For example, reversible boronic ester bonds have been used as complexation between boronic acids and 1,2- or 1,3-diols in aqueous solutions results in reversible covalent bonds (Scheme 1). Importantly, the stability of the bond depends on the pH of the solution.95,96
 |
| Scheme 1 Formation of boronic esters from boronic acid and a 1,2-diol (top) and formation of anionic boronate monoester from boronate anion and 1,2-diol (bottom). | |
Sumerlin and coworkers85 demonstrated this by crosslinking polymer-bound phenylboronic acid (PBA) with either poly(vinyl alcohol) or a catechol-functionalized copolymer (Fig. 2a). The hydrogels demonstrated self-healing at neutral and acidic pH, where the cross-links reconstituted rapidly after removing an applied strain, restoring the moduli of the original hydrogel.
 |
| Fig. 2 (a) Synthesis of self-healing hydrogel using crosslinking polymer-bound phenylboronic acid (PBA) with either poly(vinyl alcohol) or a catechol-functionalized copolymer. Reprinted with permission from ref. 73. Copyright 2015, American Chemical Society. (b) Self-healing mechanism and macroscopic self-healing of hydrogels prepared by Kloxin and coworkers. Reprinted with permission from ref. 52. Copyright 2018, American Chemical Society. | |
Kloxin, Sumerlin, and coworkers57 similarly introduced self-healing properties into hydrogels under physiological conditions by crosslinking statistical copolymers of N,N-dimethylacrylamide and a pinacol protected ester of 2-acrylamidophenylboronic acid with poly(vinyl alcohol) (Fig. 2b). The self-healing property of these gels were demonstrated in relevant cell culture media and could be used in dynamic co-cultures of breast cancer cells and lung fibroblasts. Hydrogel ‘blocks’ loaded with the different cell types were self-healed to prepare the co-culture hydrogels. These co-culture hydrogels will enable study of cellular processes such as migration, mechanotransduction, and cell–cell signaling. Similarly, Cooper-White and coworkers97 demonstrated the preparation of a cytocompatible and viscoelastic hydrogel with reversible boronate ester cross-links to probe timescale dependent mechanotransduction of fibroblasts. The viscoelastic properties (storage and loss moduli) of the gels were tuned using the equilibrium kinetics of the dynamic covalent crosslinks.
Imine bonds (Schiff bases)98 undergo reversible imine–amine exchange and this reaction (Scheme 2a) can result in self-healing properties in imine crosslinked hydrogels.
 |
| Scheme 2 (a) Reaction between aldehyde and amines to form imine bonds and (b) reaction between a ketone or aldehyde and hydrazone to form acylhydrazone bond. | |
For example, Maynard and coworkers43 developed imine-crosslinked poly(ethylene glycol) (PEG) self-healing hydrogels that demonstrated self-healing in less than 10 min. The hydrogels also demonstrated degradability when treated with pH 5.6 cell culture media because of the pH sensitivity of the imine crosslinks, and were used to encapsulate murine mesenchymal stromal cells (mMSCs) cells to evaluate the controlled release of cells. Similarly, naturally-sourced chitosan has been used in preparing dynamic self-healing hydrogels using imine bonds derived from amino-groups along the polymer backbone.99,100 In work from Zhang and coworkers,101 the amine groups in chitosan were cross-linked with benzaldehyde terminated PEG to form a hydrogel with imine crosslinks. The mechanical properties of a self-healed hydrogel were similar to those of the original gel, and a crack on the hydrogel surface completely disappeared after 2 h indicating high self-healing efficiency (Fig. 3). These gels were used for encapsulation and controlled release of proteins such as lysozyme. Other biomacromolecules such as gelatin and collagen which contain amine groups have also been used to develop imine crosslinked self-healing hydrogels.102,103
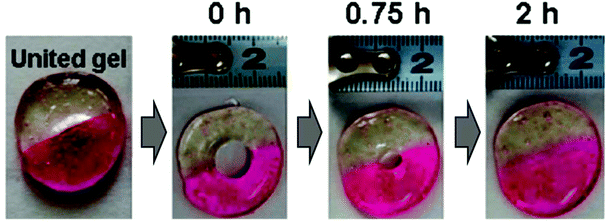 |
| Fig. 3 Self-healing with time of two imine-containing hydrogels dyed with different colors. Reprinted with permission from ref. 87. Copyright 2011, American Chemical Society. | |
Baker and coworkers104 demonstrated the reversible self-healing ability of oxidized alginate-based hydrogels crosslinked by imine-type dynamic covalent chemistries (oxime, semicarbazone, and hydrazone). The different crosslinks in the hydrogel contributed properties including self-healing, shear thinning, injectability, and printability. Interestingly, only hydrazone and semi carboazone gels could undergo self-healing, whereas all three crosslink types enabled gels to be printable and injectable (shear thinning). These hydrogels may be used to prepare bio-inks for printing extra cellular matrix (ECM) mimicking biomaterials.
The reaction between an aldehyde and a hydrazine forms an acylhydrazone bond capable of participating in reversible exchange reactions, and acylhydrazone crosslinks have been used to impart self-healing in hydrogels (Scheme 2b).105 In one example, Wang and coworkers106 prepared an acylhydrazone containing hydrogel with self-healing properties by crosslinking poly(N-isopropylacrylamide-co-hydrazone) with oxidized sodium alginate under ambient conditions (Fig. 4). The hydrogel was proposed to have potential use in controlled drug delivery and biosensing. In a similar study, Xia and coworkers107 prepared a hyaluronic acid-based hydrogel through dynamic covalent hydrazone cross-linking in the presence of a biocompatible benzimidazole-based catalyst. The use of a catalyst accelerated the formation and exchange of hydrazone bonds resulting in injectable gels. This chemistry has been proposed as being applicable for a broad range of hydrazone-based hydrogel systems for cell delivery, cell culture, and 3D printing cell scaffolds.
 |
| Fig. 4 (a) Synthesis of self-healing hydrogels using poly(N-isopropylacrylamide)-based copolymers crosslinked with oxidized sodium alginate. Adapted from, ‘Self-healing hydrogels with stimuli responsiveness based on acylhydrazone bonds’, vol. 160, 2019, pp. 246–253. Copyright 2019, with permission from Elsevier. (b) Schematic representation preparation of injectable hydrogel using diffusible organocatalyst. (c) Chemical structures of hydrazine and aldehyde-modified hyaluronic acid polymers (left) and catalysts used to accelerate hydrazone exchange (right). Adapted from, ‘Dynamic Hyaluronan Hydrogels with Temporally Modulated High Injectability and Stability Using a Biocompatible Catalyst’, vol. 30, 2018, p. 1705215. Copyright 2018, with permission from John Wiley and Sons. | |
Disulfide bonds are dynamic covalent bonds resulting from oxidation of thiols (Scheme 3).108 The self-healing of disulfide bonds can either be through an exchange reaction or a metathesis reaction, although the former is used in hydrogels because the metathesis mechanism often requires high temperatures.38
 |
| Scheme 3 Formation of disulfide bonds via oxidation of thiols (top) and thiol–disulfide exchange reaction. | |
Chen and coworkers105 developed a self-healing hydrogel combining disulfide bonds and acylhydrazone bonds, and under basic environments the hydrogels underwent self-healing by disulfide exchange reactions. The self-healing process was reversible for multiple cycles and triggered at room temperature without external stimuli. The acylhydrazone crosslinks used in these gels also triggered another self-healing mechanism under acidic conditions owing the dynamic nature of the acylhydrazone bonds. In this way, the disulfide and acylhydrazone bonds enabled reversible sol–gel transitions in the gels in response to both pH and chemicals such as dithioerythritol or hydrogen peroxide. The authors of this study suggested that the self-healing, hydrogels are potential candidates for organ repair or stimuli responsive drug delivery.
The Diels–Alder [4 + 2] cycloaddition between a diene and a dienophile is subjected to kinetic and thermodynamic control. The forward reaction. The forward reaction (Scheme 4) occurs when ΔH > TΔS and the retro reaction occurs when ΔH < TΔS.109,110 This reversibility has been used to make self-healing hydrogels.88,111,112
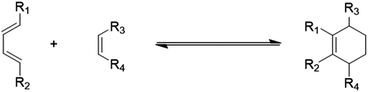 |
| Scheme 4 Bond formation resulting from the Diels–Alder reaction and retro-Diels–Alder reaction. | |
In work by Yang and coworkers,113 furyl-modified carbon nanotubes were crosslinked with maleimide end-functionalized PEG using a Diels–Alder reaction to prepare self-healing nanocomposite hydrogels. The self-healing hydrogels were able to heal when incubated at 90 °C. The mechanical properties of the self-healed nanocomposite hydrogels were assessed by tensile testing, and the stress where the hydrogel failed was up to 78% of that experienced by the original gels (Fig. 5a and b).
 |
| Fig. 5 (a) Macroscopic self-healing of carbon nanotube-containing hydrogels cut in half and then re-cured at 90 °C. (b) Stress–strain curves of the original and self-healed hydrogel after various healing times. Reprinted (adapted) with permission from ref. 95. Copyright 2017, American Chemical Society. | |
Dextran self-healing hydrogels using a Diels–Alder reaction between fulvene-modified dextran and dichloromaleic acid-modified poly(ethylene glycol) were reported to be cytocompatible by Chen and coworkers.88 The hydrogels demonstrated excellent self-healing at physiological conditions (pH 7.4 and 37 °C) within 7 h (Fig. 6). The amount of healing in the hydrogels was defined as the ratio of the depth of the scratch after different times to the depth of the scratch initially, and values of close to 100% healing were reported. The authors suggest these gels can potentially be used in biomedical applications including cell encapsulation.
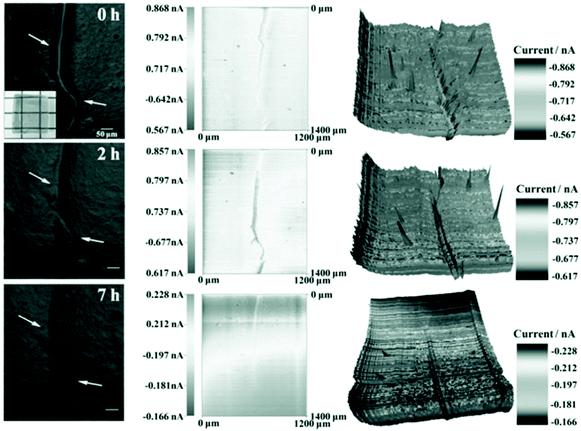 |
| Fig. 6 Optical microscopy images (left) and corresponding scanning electron microscopy (middle and right) images of self-healing of a scratch on the surface of a fulvene-modified dextran and dichloromaleic acid-modified poly(ethylene glycol) hydrogel after different times. Reprinted with permission from ref. 76. Copyright 2013, John Wiley and Sons. | |
The self-healing ability of hydrogels prepared using Diels–Alder crosslinks has also been studied by Heilshorn and coworkers.89 Hydrogels prepared using a Diels–Alder reaction between fulvenes and maleimides demonstrated improved gelation kinetics and hydrolytic stability compared to other commonly used DA reaction pairs such as furan–maleimide gels that can take hours to cross-link under physiological conditions.114
Self-healing multifunctional hydrogels are a promising class of hydrogels prepared using dynamic covalent chemistries.115 These hydrogels demonstrate self-healing properties along with another function, such as electrical conductivity, magnetism, or luminescence, in a single gel. For example, hydrogels used in artificial skin applications,116–118 soft actuators,119,120 or for artificial muscles120,121 have been reported. However, many self-healing hydrogels possess poor mechanical properties. Several efforts to develop mechanically tough self-healing hydrogels have been reported, for example preparing composite hydrogels with materials such as graphene oxide122 or using hydrophobic interactions80 to enhance the mechanical properties (toughness) of the hydrogels. High strength networks have been obtained by preparing double network hydrogels,123,124 topological hydrogels125 and tough and stretchable hydrogels.126,127 Despite these methods, there is still often a gap between the mechanical properties of self-healing gels and tough gels that can sustain MPa stress and exhibit fracture energy of the order of 1000 J m−2.84
Shape memory hydrogels
Shape memory hydrogels can be made to change shape under a specific set of conditions, such as temperature and stress, into a “fixed” temporary shape and then later relax to the original macroscopic shape under new stimuli.128,129 Physical interactions such as host–guest interactions,130–132 metal ion co-ordination,133,134 and hydrogen-bonding135,136 are often used with shape memory hydrogels. Dynamic covalent bonds can also be used for incorporating shape recovery properties into hydrogels, for example with phenylboronic acid-diol exchange reactions,137 or imine chemistry.138
In a representative example, Chen and coworkers137 developed a pH- and sugar-responsive shape memory hydrogel prepared by crosslinking phenylboronic acid modified sodium alginate (Alg-PBA) and polyvinyl alcohol (PVA). Phenyl boronic acid–diol ester bonds acted as dynamic crosslinks, while Ca2+-alginate ionic bonds behaved as permanent crosslinks in the hydrogels. The permanent shape could be recovered in a slightly acidic solution (pH = 6), or in a 0.2 M glucose solution as glucose breaks the original PBA–diol interactions (Fig. 7).
 |
| Fig. 7 Description of pH and sugar-induced shape memory effects in a reversible phenylboronic acid–diol ester hydrogel. Reprinted with permission from ref. 115. Copyright 2015, John Wiley and Sons. | |
A hydrogel with triple shape memory properties combining Schiff-base bonding with metal coordination interactions was prepared by free radical polymerization of polyacrylamide with chitosan and oxidized dextran.138 Conventional shape memory hydrogels can only memorize one temporary shape in each shape memory cycle. Triple shape memory refers to the ability of a shape memory hydrogel to memorize two temporary shapes in a single shape memory cycle.139 Reversible Schiff base bonds formed between the amino groups of chitosan and aldehyde groups on oxidized dextran enabled shape memory behavior. In alkaline media the temporary shape was fixed within 5 min due to the rapid formation of Schiff base bonds. Subsequently, the imine bonds were broken when exposed to a buffer solution at pH = 3, and the hydrogel recovered the original shape (Fig. 8). The shape recovery ratios in these hydrogels reached almost 100%, and these types of triple shape memory hydrogels have the potential to be applied in new research areas such as actuators, defect recoverable coatings, and smart adhesives.
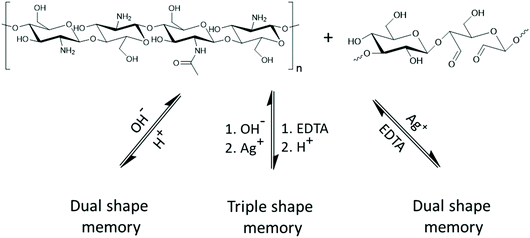 |
| Fig. 8 Schiff base bonds in the triple shape memory gel hydrogel reported by Chen and co-workers respond to pH and Ag+ ions.138 | |
Hydrogels with stimuli-induced stiffness changes
Hydrogels with material properties that can undergo temporal changes (i.e. changes with time) are gaining increasing attention in regenerative medicine140,141 and drug delivery.142 Bond formation or bond breaking in response to external stimuli changes the crosslink density in dynamic hydrogels, and hence the stiffness of the gel changes.143,144 Different strategies have been proposed to prepare temporally dynamic gels, including forming crosslinks through host–guest interactions,145 ionic interactions,146cis/trans azobenzene isomerization,147 and reversible covalent bonds.148 Free radical polymerization,149,150 photocleavage of crosslinks,144,151 supramolecular guest–host interactions,152 ionic interactions,153 and metal ion co-ordination154 have also been used to cause temporal changes in hydrogel properties, however their discussion falls outside the scope of our review.
Disulfide exchange reactions have been used in dynamic hydrogels.155,156 As we described for self-healing hydrogels, disulfide crosslinks can be cleaved or formed via physiologically relevant reduction/oxidation reactions or thiol–disulfide exchange reactions.157,158 A recent example of thiol–disulfide exchange reactions to form and subsequently dissolve a hydrogel used a zwitterionic carboxybetaine disulfide crosslinker (Fig. 9).158 Hydrogels using the zwitterionic carboxybetaine disulfide crosslinker underwent mass loss in less than 10 min, this is a stark contrast to the hydrolytically-degraded bonds typically used, such as esters and carbonates, which exhibit degradation kinetics from days to months.159,160 The disulfide crosslinker could be split upon exposure to redox stimuli such as dithiothreitol, and the hydrogels were used to encapsulate fluorescein isothiocyanate-labeled bovine serum albumin and then release the protein in a controlled manner using different concentrations of dithiothreitol.
 |
| Fig. 9 Chemical structure of the carboxybetaine disulfide cross-linker used by Jiang and co-workers.158 | |
Disulfide crosslinks have also been used in combination with static crosslinks to soften hydrogels without dissolving the gel. An example of this was described in work from our lab,157 where gelatin-based hydrogels were prepared using both alkyl sulfide and disulfide crosslinks. Norbornene modified gelatin was crosslinked with a thiol containing crosslinking polymer maintaining an excess concentration of thiols to enable disulfide formation (Fig. 10a). The thiol–disulfide exchange reactions were performed using 2-mercaptoethanol to soften the hydrogels by 15–25% of their original mechanical properties (Fig. 10b).
 |
| Fig. 10 (a) Structures of crosslinks in the thiol norbornene hydrogels. (b) Softening of the hydrogels via thiol–disulfide exchange reactions. | |
Conversely, Peyton and coworkers161 demonstrated that disulfide bonding could stiffen hydrogels under shear by using disulfide reduction and formation chemistry in PEG-based hydrogels using a “latent crosslinking” approach with a disulfide containing monomer. Interestingly, these gels could be cycled through several oxidation/reduction cycles with the thiols, leading to reversible changes in the moduli of the gels.
Thiol–thioester bonds undergo reversible exchange reactions in aqueous solution (Scheme 5).162–164 In the thiol–thioester exchange reaction, a thiolate anion reacts with a thioester to form new thiolate and thioester products. Hupe and Jencks experimentally showed that the rate of the thioester exchange is a function of the pKa of the conjugate acid of the incoming thiol and the leaving thiolate anion.165
 |
| Scheme 5 Generic reaction scheme of thiol–thioester exchange reaction. | |
In work by Grinstaff and coworkers,166 thioester exchange was used to induce degradation in hydrogel wound sealants in the presence of L-cysteine methyl ester. The hydrogels consisted of lysine-based dendrons and thioester-containing crosslinkers (Fig. 11). The crosslinker was end capped with N-hydroxy succinimide ester, which reacted with the amines in the dendron to form the network. The thioester containing hydrogels dissolved upon exposure to L-cysteine methyl ester within 30 min, but no changes in stiffness were observed when lysine methyl ester was used, or if L-cysteine methyl ester was used on a hydrogel without thioester linkages.
 |
| Fig. 11 Reaction between an amine-functionalized dendron and a thioether containing crosslinker forms hydrogels with “on-demand” hydrogel degradation using thiol–thioester exchange reaction with an L-cysteine methyl ester solution. Reprinted with permission from ref. 144. Copyright 2016, John Wiley and Sons. | |
Anseth and coworkers167 have prepared adaptable tissue engineering scaffolds for regenerative biology using this thiol–thioester exchange chemistry. In their study, hydrogels containing an 8-arm thiolated polyethylene glycol were crosslinked with a thioester-containing divinyl crosslinker using a photoinitiated thiol–ene reaction (Fig. 12).
 |
| Fig. 12 Star-PEG macromonomers that are end-functionalized with thiols were crosslinked using thioester di(vinyl ether) (TEDVE) with and without thioesters to prepare adaptable and static hydrogels respectively. Reprinted with permission from ref. 145. Copyright 2018, John Wiley and Sons. | |
The thioester-containing hydrogels could be completely dissolved by L-cysteine at physiological pH, as the pKa of the conjugate base of L-cysteine is lower than that of the thioester formed after the exchange reaction. The breakdown of the hydrogel was solely due to the presence of thioester linkages in the network, this was confirmed by a control experiment where a similar hydrogel was prepared using a poly(ethylene glycol) diene crosslinker instead of TEDVE (Fig. 12) resulting in permanent covalent crosslinks. The hydrogels were prepared so that they possessed similar initial material properties regardless of the type of crosslinker used. While the biological relevance and high efficiency of the exchange reaction is useful for biomedical applications, the reaction efficiency in organic solvents and compatibility with a wide pH range also makes this chemistry suitable for non-biological applications.168,169
Summary
Hydrogels have become far more complex than a network of hydrophilic polymers with a large volume of imbibed water and are increasingly being used in a variety of application areas. In this review we have highlighted the main dynamic covalent chemistries used to prepare functional hydrogels with dynamic covalent bonds. While the function can change, e.g. self-healing, temporal responsiveness, etc. it is clear that a “toolbox” of dynamic covalent bonding is typically used. It is not surprising that these dynamic bonds are compatible with different functional groups and tolerant to different reaction conditions, making them ideal for materials development. Through this mini-review we have also touched on some persistent challenges for dynamic hydrogels, for example a lack of toughness, and highlighted some emerging and creative approaches to mitigating these challenges such as double networks and composite gels. As this field continues to evolve it will be interesting to see how dynamic bonding will be combined with existing and other nascent strategies, for example tyrosine dimers,170 to give value added properties in advanced applications, including drug delivery, biomaterials, and non-biomedical applications such as sensing,171–173 chemical separation,174 physical and chemical absorption agents175 and adhesive agents.176
Conflicts of interest
There are no conflicts of interest to declare.
References
- T. Canal and N. A. Peppas, J. Biomed. Mater. Res., 1989, 23(10), 1183–1193 CrossRef CAS PubMed.
- E. A. Schneider, Z. Anorg. Chem., 1894, 7(1), 386–391 CrossRef.
- O. Wichterle and D. Lím, Nature, 1960, 185(4706), 117–118 CrossRef.
- Y. Qiu and K. Park, Adv. Drug Delivery Rev., 2001, 53(3), 321–339 CrossRef CAS PubMed.
- P. Ghasemiyeh and S. Mohammadi-Samani, Trends Pharm. Sci., 2019, 5(1), 7–24 CAS.
- M. M. Perera, D. M. Fischesser, J. D. Molkentin and N. Ayres, Polym. Chem., 2019, 10(46), 6360–6367 RSC.
- M. C. Cushing and K. S. Anseth, Science, 2007, 316(5828), 1133 CrossRef CAS PubMed.
- R. Edri, I. Gal, N. Noor, T. Harel, S. Fleischer, N. Adadi, O. Green, D. Shabat, L. Heller, A. Shapira, I. Gat-Viks, D. Peer and T. Dvir, Adv. Mater., 2019, 31(1), 1803895 CrossRef PubMed.
- M. M. Islam, R. Ravichandran, D. Olsen, M. K. Ljunggren, P. Fagerholm, C. J. Lee, M. Griffith and J. Phopase, RSC Adv., 2016, 6(61), 55745–55749 RSC.
- M. Liu, X. Zeng, C. Ma, H. Yi, Z. Ali, X. Mou, S. Li, Y. Deng and N. He, Bone Res., 2017, 5(1), 17014 CrossRef CAS PubMed.
- J. Leijten, J. Seo, K. Yue, G. Trujillo-de Santiago, A. Tamayol, G. U. Ruiz-Esparza, S. R. Shin, R. Sharifi, I. Noshadi, M. M. Álvarez, Y. S. Zhang and A. Khademhosseini, Mater. Sci. Eng., R, 2017, 119, 1–35 CrossRef PubMed.
- S.-H. Hyon, W.-I. Cha, Y. Ikada, M. Kita, Y. Ogura and Y. Honda, J. Biomater. Sci., Polym. Ed., 1994, 5(5), 397–406 CrossRef CAS PubMed.
- L. Xinming, C. Yingde, A. W. Lloyd, S. V. Mikhalovsky, S. R. Sandeman, C. A. Howel and L. Liewen, Cont. Lens Anterior Eye, 2008, 31(2), 57–64 CrossRef PubMed.
- N. A. Peppas, P. Bures, W. Leobandung and H. Ichikawa, Eur. J. Pharm. Biopharm., 2000, 50(1), 27–46 CrossRef CAS PubMed.
- F. Ahmadi, Z. Oveisi, S. M. Samani and Z. Amoozgar, Res. Pharm. Sci., 2015, 10(1), 1–16 CAS.
- M. E. Parente, A. Ochoa Andrade, G. Ares, F. Russo and Á. Jiménez-Kairuz, Int. J. Cosmet. Sci., 2015, 37(5), 511–518 CrossRef CAS PubMed.
- C. Ghobril and M. W. Grinstaff, Chem. Soc. Rev., 2015, 44(7), 1820–1835 RSC.
- C. Jeon and W. H. Höll, Water Res., 2003, 37(19), 4770–4780 CrossRef CAS PubMed.
- E. Ramírez, S. G. Burillo, C. Barrera-Díaz, G. Roa and B. Bilyeu, J. Hazard. Mater., 2011, 192(2), 432–439 CrossRef PubMed.
- D. Buenger, F. Topuz and J. Groll, Prog. Polym. Sci., 2012, 37(12), 1678–1719 CrossRef CAS.
- G. Gerlach, M. Guenther, J. Sorber, G. Suchaneck, K.-F. Arndt and A. Richter, Sens. Actuators, B, 2005, 111–112, 555–561 CrossRef CAS.
- S. R. Shin, C. Zihlmann, M. Akbari, P. Assawes, L. Cheung, K. Zhang, V. Manoharan, Y. S. Zhang, M. Yüksekkaya, K.-t. Wan, M. Nikkhah, M. R. Dokmeci, X. Tang and A. Khademhosseini, Small, 2016, 12(27), 3677–3689 CrossRef CAS PubMed.
- N. Annabi, S. R. Shin, A. Tamayol, M. Miscuglio, M. A. Bakooshli, A. Assmann, P. Mostafalu, J.-Y. Sun, S. Mithieux, L. Cheung, X. Tang, A. S. Weiss and A. Khademhosseini, Adv. Mater., 2016, 28(1), 40–49 CrossRef CAS PubMed.
- A. Khademhosseini and R. Langer, Biomaterials, 2007, 28(34), 5087–5092 CrossRef CAS PubMed.
- N. A. Peppas, J. Z. Hilt, A. Khademhosseini and R. Langer, Adv. Mater., 2006, 18(11), 1345–1360 CrossRef CAS.
- C. B. Highley, G. D. Prestwich and J. A. Burdick, Curr. Opin. Biotechnol., 2016, 40, 35–40 CrossRef CAS PubMed.
- J. A. Rowley, G. Madlambayan and D. J. Mooney, Biomaterials, 1999, 20(1), 45–53 CrossRef CAS PubMed.
- A. Watarai, L. Schirmer, S. Thönes, U. Freudenberg, C. Werner, J. C. Simon and U. Anderegg, Acta Biomater., 2015, 25, 65–75 CrossRef CAS PubMed.
- D. Loessner, C. Meinert, E. Kaemmerer, L. C. Martine, K. Yue, P. A. Levett, T. J. Klein, F. P. W. Melchels, A. Khademhosseini and D. W. Hutmacher, Nat. Protoc., 2016, 11, 727–746 CrossRef CAS PubMed.
- J. Lou, R. Stowers, S. Nam, Y. Xia and O. Chaudhuri, Biomaterials, 2018, 154, 213–222 CrossRef CAS PubMed.
- W. E. Hennink and C. F. van Nostrum, Adv. Drug Delivery Rev., 2002, 54(1), 13–36 CrossRef CAS.
- E. Hui, K. I. Gimeno, G. Guan and S. R. Caliari, Biomacromolecules, 2019, 4126–4134 CrossRef CAS PubMed.
- S. J. de Jong, B. van Eerdenbrugh, C. F. van Nostrum, J. J. Kettenes-van den Bosch and W. E. Hennink, J. Controlled Release, 2001, 71(3), 261–275 CrossRef CAS PubMed.
- N. A. Peppas, H. J. Moynihan and L. M. Lucht, J. Biomed. Mater. Res., 1985, 19(4), 397–411 CrossRef CAS PubMed.
- K. Y. Lee, K. H. Bouhadir and D. J. Mooney, Biomaterials, 2004, 25(13), 2461–2466 CrossRef CAS PubMed.
- F. Ullah, M. B. H. Othman, F. Javed, Z. Ahmad and H. M. Akil, Mater. Sci. Eng., C, 2015, 57, 414–433 CrossRef CAS PubMed.
- Y. Jin, C. Yu, R. J. Denman and W. Zhang, Chem. Soc. Rev., 2013, 42(16), 6634–6654 RSC.
- X. Z. Shu, Y. Liu, Y. Luo, M. C. Roberts and G. D. Prestwich, Biomacromolecules, 2002, 3(6), 1304–1311 CrossRef CAS PubMed.
- L. Wang, L. Li, X. Wang, D. Huang, F. Yang, H. Shen, Z. Li and D. Wu, Polym. Chem., 2016, 7(7), 1429–1438 RSC.
- M. E. Bracchi, G. Dura and D. A. Fulton, Polym. Chem., 2019, 10(10), 1258–1267 RSC.
- B. M. Richardson, D. G. Wilcox, M. A. Randolph and K. S. Anseth, Acta Biomater., 2019, 83, 71–82 CrossRef CAS PubMed.
- L. L. Wang, C. B. Highley, Y.-C. Yeh, J. H. Galarraga, S. Uman and J. A. Burdick, J. Biomed. Mater. Res., Part A, 2018, 106(4), 865–875 CrossRef CAS PubMed.
- N. Boehnke, C. Cam, E. Bat, T. Segura and H. D. Maynard, Biomacromolecules, 2015, 16(7), 2101–2108 CrossRef CAS PubMed.
-
M. W. Toepke and W. L. Murphy, 1.31 Dynamic Hydrogels, in Comprehensive Biomaterials II, ed. P. Ducheyne, Elsevier, Oxford, 2017, pp. 705–724 Search PubMed.
- L. Zhang, Z. Cao, T. Bai, L. Carr, J.-R. Ella-Menye, C. Irvin, B. D. Ratner and S. Jiang, Nat. Biotechnol., 2013, 31, 553 CrossRef CAS PubMed.
- G. D. Nicodemus and S. J. Bryant, Tissue Eng., Part B, 2008, 14(2), 149–165 CrossRef CAS PubMed.
- W. Shen, K. Zhang, J. A. Kornfield and D. A. Tirrell, Nat. Mater., 2006, 5, 153 CrossRef CAS PubMed.
- M. Hamidi, A. Azadi and P. Rafiei, Adv. Drug Delivery Rev., 2008, 60(15), 1638–1649 CrossRef CAS PubMed.
- L. Klouda and A. G. Mikos, Eur. J. Pharm. Biopharm., 2008, 68(1), 34–45 CrossRef CAS PubMed.
- P. Gupta, K. Vermani and S. Garg, Drug Discovery Today, 2002, 7(10), 569–579 CrossRef CAS PubMed.
- I. Tomatsu, K. Peng and A. Kros, Adv. Drug Delivery Rev., 2011, 63(14), 1257–1266 CrossRef CAS PubMed.
- R. V. Ulijn, N. Bibi, V. Jayawarna, P. D. Thornton, S. J. Todd, R. J. Mart, A. M. Smith and J. E. Gough, Mater. Today, 2007, 10(4), 40–48 CrossRef CAS.
- T. Sun, C. Zhu and J. Xu, Soft Matter, 2018, 14(6), 921–926 RSC.
- E. A. Appel, X. J. Loh, S. T. Jones, F. Biedermann, C. A. Dreiss and O. A. Scherman, J. Am. Chem. Soc., 2012, 134(28), 11767–11773 CrossRef CAS PubMed.
- Y. Osada and A. Matsuda, Nature, 1995, 376(6537), 219–219 CrossRef CAS PubMed.
- A. Phadke, C. Zhang, B. Arman, C.-C. Hsu, R. A. Mashelkar, A. K. Lele, M. J. Tauber, G. Arya and S. Varghese, Proc. Natl. Acad. Sci. U. S. A., 2012, 109(12), 4383 CrossRef CAS PubMed.
- M. E. Smithmyer, C. C. Deng, S. E. Cassel, P. J. LeValley, B. S. Sumerlin and A. M. Kloxin, ACS Macro Lett., 2018, 7(9), 1105–1110 CrossRef CAS.
- D. L. Taylor and M. in het Panhuis, Adv. Mater., 2016, 28(41), 9060–9093 CrossRef CAS PubMed.
- C. Kim, H. Ejima and N. Yoshie, J. Mater. Chem. A, 2018, 6(40), 19643–19652 RSC.
- W. J. Yang, X. Tao, T. Zhao, L. Weng, E.-T. Kang and L. Wang, Polym. Chem., 2015, 6(39), 7027–7035 RSC.
- Y. Liu, H. Meng, Z. Qian, N. Fan, W. Choi, F. Zhao and B. P. Lee, Angew. Chem., Int. Ed., 2017, 56(15), 4224–4228 CrossRef CAS PubMed.
- J. Qu, X. Zhao, Y. Liang, T. Zhang, P. X. Ma and B. Guo, Biomaterials, 2018, 183, 185–199 CrossRef CAS PubMed.
- R. A. Bilodeau and R. K. Kramer, Front. Robot. AI, 2017, 4(48) DOI:10.3389/frobt.2017.00048.
- B. Guo, J. Qu, X. Zhao and M. Zhang, Acta Biomater., 2019, 84, 180–193 CrossRef CAS PubMed.
- L. Yang, Y. Li, Y. Gou, X. Wang, X. Zhao and L. Tao, Polym. Chem., 2017, 8(34), 5071–5076 RSC.
- J. Dahlke, S. Zechel, M. D. Hager and U. S. Schubert, Adv. Mater. Interfaces, 2018, 5(17), 1800051 CrossRef.
- H. Liu, X. Sui, H. Xu, L. Zhang, Y. Zhong and Z. Mao, Macromol. Mater. Eng., 2016, 301(6), 725–732 CrossRef CAS.
- Z. Wei, J. H. Yang, Z. Q. Liu, F. Xu, J. X. Zhou, M. Zrínyi, Y. Osada and Y. M. Chen, Adv. Funct. Mater., 2015, 25(9), 1352–1359 CrossRef CAS.
- G. Zhang, L. Lv, Y. Deng and C. Wang, Macromol. Rapid Commun., 2017, 38(12), 1700018 CrossRef PubMed.
- L. Teng, Y. Chen, M. Jin, Y. Jia, Y. Wang and L. Ren, Biomacromolecules, 2018, 19(6), 1939–1949 CrossRef CAS PubMed.
- M. M. C. Bastings, S. Koudstaal, R. E. Kieltyka, Y. Nakano, A. C. H. Pape, D. A. M. Feyen, F. J. van Slochteren, P. A. Doevendans, J. P. G. Sluijter, E. W. Meijer, S. A. J. Chamuleau and P. Y. W. Dankers, Adv. Healthcare Mater., 2013, 3(1), 70–78 CrossRef PubMed.
- L. Peng, H. Zhang, A. Feng, M. Huo, Z. Wang, J. Hu, W. Gao and J. Yuan, Polym. Chem., 2015, 6(19), 3652–3659 RSC.
- M. Zhang, D. Xu, X. Yan, J. Chen, S. Dong, B. Zheng and F. Huang, Angew. Chem., 2012, 124(28), 7117–7121 CrossRef.
- M. Nakahata, Y. Takashima, H. Yamaguchi and A. Harada, Nat. Commun., 2011, 2, 511–515 CrossRef PubMed.
- J.-Y. Sun, X. Zhao, W. R. K. Illeperuma, O. Chaudhuri, K. H. Oh, D. J. Mooney, J. J. Vlassak and Z. Suo, Nature, 2012, 489, 133–136 CrossRef CAS PubMed.
- A. B. South and L. A. Lyon, Angew. Chem., Int. Ed., 2010, 49(4), 767–771 CrossRef CAS PubMed.
- H. Liang, Z. Zhang, Q. Yuan and J. Liu, Chem. Commun., 2015, 51(82), 15196–15199 RSC.
- J. Cao, L. Meng, S. Zheng, Z. Li, J. Jiang and X. Lv, Int. J. Polym. Mater. Polym. Biomater., 2016, 65(10), 537–542 CrossRef CAS.
- Y. Xu, Q. Wu, Y. Sun, H. Bai and G. Shi, ACS Nano, 2010, 4(12), 7358–7362 CrossRef CAS PubMed.
- D. C. Tuncaboylu, M. Sari, W. Oppermann and O. Okay, Macromolecules, 2011, 44(12), 4997–5005 CrossRef CAS.
- T. Kakuta, Y. Takashima, M. Nakahata, M. Otsubo, H. Yamaguchi and A. Harada, Adv. Mater., 2013, 25(20), 2849–2853 CrossRef CAS PubMed.
- D. C. Tuncaboylu, A. Argun, M. Sahin, M. Sari and O. Okay, Polymer, 2012, 53(24), 5513–5522 CrossRef CAS.
- C. T. S. Wong Po Foo, J. S. Lee, W. Mulyasasmita, A. Parisi-Amon and S. C. Heilshorn, Proc. Natl. Acad. Sci. U. S. A., 2009, 106(52), 22067–22072 CrossRef PubMed.
- W. Wang, R. Narain and H. Zeng, Front. Chem., 2018, 6, 497–497 CrossRef CAS PubMed.
- C. C. Deng, W. L. A. Brooks, K. A. Abboud and B. S. Sumerlin, ACS Macro Lett., 2015, 4(2), 220–224 CrossRef CAS.
- J. Xu, D. Yang, W. Li, Y. Gao, H. Chen and H. Li, Polymer, 2011, 52(19), 4268–4276 CrossRef CAS.
- Y.-L. Liu and T.-W. Chuo, Polym. Chem., 2013, 4(7), 2194–2205 RSC.
- Z. Wei, J. H. Yang, X. J. Du, F. Xu, M. Zrinyi, Y. Osada, F. Li and Y. M. Chen, Macromol. Rapid Commun., 2013, 34(18), 1464–1470 CrossRef CAS.
- C. M. Madl and S. C. Heilshorn, Chem. Mater., 2019, 31(19), 8035–8043 CrossRef CAS.
- S. Mukherjee, M. R. Hill and B. S. Sumerlin, Soft Matter, 2015, 11(30), 6152–6161 RSC.
- Y. Xu, Y. Li, Q. Chen, L. Fu, L. Tao and Y. Wei, Int. J. Mol. Sci., 2018, 19(8), 2198 CrossRef PubMed.
- H. Ying, Y. Zhang and J. Cheng, Nat. Commun., 2014, 5, 3218–3227 CrossRef PubMed.
- C. E. Yuan, M. Z. Rong, M. Q. Zhang, Z. P. Zhang and Y. C. Yuan, Chem. Mater., 2011, 23(22), 5076–5081 CrossRef CAS.
- Y. Yang and M. W. Urban, Chem. Soc. Rev., 2013, 42(17), 7446–7467 RSC.
- S. D. Bull, M. G. Davidson, J. M. H. van den Elsen, J. S. Fossey, A. T. A. Jenkins, Y.-B. Jiang, Y. Kubo, F. Marken, K. Sakurai, J. Zhao and T. D. James, Acc. Chem. Res., 2013, 46(2), 312–326 CrossRef CAS PubMed.
- S. Spoljaric, A. Salminen, N. D. Luong and J. Seppälä, Eur. Polym. J., 2014, 56, 105–117 CrossRef CAS.
- I. A. Marozas, K. S. Anseth and J. J. Cooper-White, Biomaterials, 2019, 223, 119430 CrossRef CAS PubMed.
- Q. Li, C. Liu, J. Wen, Y. Wu, Y. Shan and J. Liao, Chin. Chem. Lett., 2017, 28(9), 1857–1874 CrossRef CAS.
- M. N. V. Ravi Kumar, React. Funct. Polym., 2000, 46(1), 1–27 CrossRef.
- Y. Xu, P. A. Patsis, S. Hauser, D. Voigt, R. Rothe, M. Günther, M. Cui, X. Yang, R. Wieduwild, K. Eckert, C. Neinhuis, T. F. Akbar, I. R. Minev, J. Pietzsch and Y. Zhang, Adv. Sci., 2019, 6(15), 1802077 CrossRef PubMed.
- Y. Zhang, L. Tao, S. Li and Y. Wei, Biomacromolecules, 2011, 12(8), 2894–2901 CrossRef CAS PubMed.
- M. Rafat, F. Li, P. Fagerholm, N. S. Lagali, M. A. Watsky, R. Munger, T. Matsuura and M. Griffith, Biomaterials, 2008, 29(29), 3960–3972 CrossRef CAS PubMed.
- J. Zhang, Y. Yang, Y. Chen, X. Liu, S. Guo, L. Zhu and Y. Wang, J. Mater. Chem. B, 2016, 4(5), 973–981 RSC.
- S. Hafeez, W. H. Ooi, L. C. F. Morgan, C. Mota, M. Dettin, C. Van Blitterswijk, L. Moroni and B. M. Baker, Gels, 2018, 4(4), 85 CrossRef CAS PubMed.
- G. Deng, F. Li, H. Yu, F. Liu, C. Liu, W. Sun, H. Jiang and Y. Chen, ACS Macro Lett., 2012, 1(2), 275–279 CrossRef CAS.
- C. Sun, H. Jia, K. Lei, D. Zhu, Y. Gao, Z. Zheng and X. Wang, Polymer, 2019, 160, 246–253 CrossRef CAS.
- J. Lou, F. Liu, C. D. Lindsay, O. Chaudhuri, S. C. Heilshorn and Y. Xia, Adv. Mater., 2018, 30(22), 1705215 CrossRef.
- M. Pepels, I. Filot, B. Klumperman and H. Goossens, Polym. Chem., 2013, 4(18), 4955–4965 RSC.
- K. F. Wong and C. A. Eckert, Trans. Faraday Soc., 1970, 66, 2313–2319 RSC.
- C. M. Nimmo, S. C. Owen and M. S. Shoichet, Biomacromolecules, 2011, 12(3), 824–830 CrossRef CAS PubMed.
- S. Kirchhof, A. Strasser, H.-J. Wittmann, V. Messmann, N. Hammer, A. M. Goepferich and F. P. Brandl, J. Mater. Chem. B, 2015, 3(3), 449–457 RSC.
- L. J. Smith, S. M. Taimoory, R. Y. Tam, A. E. G. Baker, N. Binth Mohammad, J. F. Trant and M. S. Shoichet, Biomacromolecules, 2018, 19(3), 926–935 CrossRef CAS PubMed.
- C. Shao, M. Wang, H. Chang, F. Xu and J. Yang, ACS Sustainable Chem. Eng., 2017, 5(7), 6167–6174 CrossRef CAS.
- S. Kirchhof, M. Gregoritza, V. Messmann, N. Hammer, A. M. Goepferich and F. P. Brandl, Eur. J. Pharm. Biopharm., 2015, 96, 217–225 CrossRef CAS PubMed.
- X. Zhang, J. Liu, Y. Gao, J. Hao, J. Hu and Y. Ju, Soft Matter, 2019, 15(23), 4662–4668 RSC.
- C. Hou, T. Huang, H. Wang, H. Yu, Q. Zhang and Y. Li, Sci. Rep., 2013, 3, 3138 CrossRef PubMed.
- Z. Lei, Q. Wang, S. Sun, W. Zhu and P. Wu, Adv. Mater., 2017, 29(22), 1700321 CrossRef PubMed.
- M. A. Darabi, A. Khosrozadeh, R. Mbeleck, Y. Liu, Q. Chang, J. Jiang, J. Cai, Q. Wang, G. Luo and M. Xing, Adv. Mater., 2017, 29(31), 1700533 CrossRef PubMed.
- E. Acome, S. K. Mitchell, T. G. Morrissey, M. B. Emmett, C. Benjamin, M. King, M. Radakovitz and C. Keplinger, Science, 2018, 359(6371), 61 CrossRef CAS PubMed.
- H. Qin, T. Zhang, N. Li, H.-P. Cong and S.-H. Yu, Nat. Commun., 2019, 10(1), 2202 CrossRef PubMed.
- B. C. K. Tee, C. Wang, R. Allen and Z. Bao, Nat. Nanotechnol., 2012, 7, 825–832 CrossRef CAS PubMed.
- J. Liu, G. Song, C. He and H. Wang, Macromol. Rapid Commun., 2013, 34(12), 1002–1007 CrossRef CAS PubMed.
- H. J. Zhang, T. L. Sun, A. K. Zhang, Y. Ikura, T. Nakajima, T. Nonoyama, T. Kurokawa, O. Ito, H. Ishitobi and J. P. Gong, Adv. Mater., 2016, 28(24), 4884–4890 CrossRef CAS PubMed.
- T. Nonoyama, S. Wada, R. Kiyama, N. Kitamura, M. T. I. Mredha, X. Zhang, T. Kurokawa, T. Nakajima, Y. Takagi, K. Yasuda and J. P. Gong, Adv. Mater., 2016, 28(31), 6740–6745 CrossRef CAS PubMed.
- K. Ito, Polym. J., 2007, 39(6), 489–499 CrossRef CAS.
- J.-Y. Sun, X. Zhao, W. R. K. Illeperuma, O. Chaudhuri, K. H. Oh, D. J. Mooney, J. J. Vlassak and Z. Suo, Nature, 2012, 489(7414), 133–136 CrossRef CAS PubMed.
- X. P. Morelle, W. R. Illeperuma, K. Tian, R. Bai, Z. Suo and J. J. Vlassak, Adv. Mater., 2018, 30(35), 1801541 CrossRef PubMed.
- A. Lendlein and R. Langer, Science, 2002, 296(5573), 1673–1676 CrossRef PubMed.
- C. Löwenberg, M. Balk, C. Wischke, M. Behl and A. Lendlein, Acc. Chem. Res., 2017, 50(4), 723–732 CrossRef PubMed.
- K. Miyamae, M. Nakahata, Y. Takashima and A. Harada, Angew. Chem., Int. Ed., 2015, 54(31), 8984–8987 CrossRef CAS PubMed.
- W. Lu, X. Le, J. Zhang, Y. Huang and T. Chen, Chem. Soc. Rev., 2017, 46(5), 1284–1294 RSC.
- A. Yasin, W. Zhou, H. Yang, H. Li, Y. Chen and X. Zhang, Macromol. Rapid Commun., 2015, 36(9), 845–851 CrossRef CAS PubMed.
- S. Y. Zheng, H. Ding, J. Qian, J. Yin, Z. L. Wu, Y. Song and Q. Zheng, Macromolecules, 2016, 49(24), 9637–9646 CrossRef CAS.
- A. Yasin, H. Li, Z. Lu, S. U. Rehman, M. Siddiq and H. Yang, Soft Matter, 2014, 10(7), 972–977 RSC.
- Y. Zhang, Y. Li and W. Liu, Adv. Funct. Mater., 2015, 25(3), 471–480 CrossRef CAS.
- B. Xu, Y. Zhang and W. Liu, Macromol. Rapid Commun., 2015, 36(17), 1585–1591 CrossRef CAS PubMed.
- H. Meng, J. Zheng, X. Wen, Z. Cai, J. Zhang and T. Chen, Macromol. Rapid Commun., 2015, 36(6), 533–537 CrossRef CAS PubMed.
- H. Xiao, W. Lu, X. Le, C. Ma, Z. Li, J. Zheng, J. Zhang, Y. Huang and T. Chen, Chem. Commun., 2016, 52(90), 13292–13295 RSC.
- T. Xie, Nature, 2010, 464(7286), 267–270 CrossRef CAS PubMed.
- J.-H. Lee and H.-W. Kim, J. Tissue Eng., 2018, 9 Search PubMed , 2041731418768285.
- A. M. Kloxin, A. M. Kasko, C. N. Salinas and K. S. Anseth, Science, 2009, 324(5923), 59–63 CrossRef CAS PubMed.
- A. Lueckgen, D. S. Garske, A. Ellinghaus, R. M. Desai, A. G. Stafford, D. J. Mooney, G. N. Duda and A. Cipitria, Biomaterials, 2018, 181, 189–198 CrossRef CAS PubMed.
- M. D. Konieczynska and M. W. Grinstaff, Acc. Chem. Res., 2017, 50(2), 151–160 CrossRef CAS PubMed.
- A. M. Rosales and K. S. Anseth, Nat. Rev. Mater., 2016, 1, 15012 CrossRef CAS PubMed.
- Z. Zheng, J. Hu, H. Wang, J. Huang, Y. Yu, Q. Zhang and Y. Cheng, ACS Appl. Mater. Interfaces, 2017, 9(29), 24511–24517 CrossRef CAS PubMed.
- R. S. Stowers, S. C. Allen and L. J. Suggs, Proc. Natl. Acad. Sci. U. S. A., 2015, 112(7), 1953 CrossRef CAS PubMed.
- A. M. Rosales, K. M. Mabry, E. M. Nehls and K. S. Anseth, Biomacromolecules, 2015, 16(3), 798–806 CrossRef CAS PubMed.
- H. Wang and S. C. Heilshorn, Adv. Mater., 2015, 27(25), 3717–3736 CrossRef CAS PubMed.
- M. Guvendiren and J. A. Burdick, Nat. Commun., 2012, 3, 792–800 CrossRef PubMed.
- S. R. Caliari, M. Perepelyuk, B. D. Cosgrove, S. J. Tsai, G. Y. Lee, R. L. Mauck, R. G. Wells and J. A. Burdick, Sci. Rep., 2016, 6, 21387–21396 CrossRef CAS PubMed.
- B. A. Badeau, M. P. Comerford, C. K. Arakawa, J. A. Shadish and C. A. DeForest, Nat. Chem., 2018, 10, 251–258 CrossRef CAS PubMed.
- T. Xiao, L. Xu, L. Zhou, X.-Q. Sun, C. Lin and L. Wang, J. Mater. Chem. B, 2019, 7(10), 1526–1540 RSC.
- M. Zhong, Y.-T. Liu and X.-M. Xie, J. Mater. Chem. B, 2015, 3(19), 4001–4008 RSC.
- S. C. Grindy and N. Holten-Andersen, Soft Matter, 2017, 13(22), 4057–4065 RSC.
- S. P. Black, J. K. M. Sanders and A. R. Stefankiewicz, Chem. Soc. Rev., 2014, 43(6), 1861–1872 RSC.
- G. A. Barcan, X. Zhang and R. M. Waymouth, J. Am. Chem. Soc., 2015, 137(17), 5650–5653 CrossRef CAS PubMed.
- M. M. Perera and N. Ayres, Polym. Chem., 2017, 8(44), 6741–6749 RSC.
- P. Jain, H.-C. Hung, B. Li, J. Ma, D. Dong, X. Lin, A. Sinclair, P. Zhang, M. B. O'Kelly, L. Niu and S. Jiang, Langmuir, 2019, 35(5), 1864–1871 CrossRef CAS PubMed.
- Y. S. Jo, J. Gantz, J. A. Hubbell and M. P. Lutolf, Soft Matter, 2009, 5(2), 440–446 RSC.
- A. T. Metters, K. S. Anseth and C. N. Bowman, Polymer, 2000, 41(11), 3993–4004 CrossRef CAS.
- Y. H. Tran, M. J. Rasmuson, T. Emrick, J. Klier and S. R. Peyton, Soft Matter, 2017, 13(47), 9007–9014 RSC.
- P. T. Corbett, J. Leclaire, L. Vial, K. R. West, J.-L. Wietor, J. K. M. Sanders and S. Otto, Chem. Rev., 2006, 106(9), 3652–3711 CrossRef CAS PubMed.
- P. J. Bracher, P. W. Snyder, B. R. Bohall and G. M. Whitesides, Origins Life Evol. Biospheres, 2011, 41(5), 399–412 CrossRef CAS PubMed.
- C. Ghobril, K. Charoen, E. K. Rodriguez, A. Nazarian and M. W. Grinstaff, Angew. Chem., Int. Ed., 2013, 52(52), 14070–14074 CrossRef CAS PubMed.
- D. J. Hupe and W. P. Jencks, J. Am. Chem. Soc., 1977, 99(2), 451–464 CrossRef CAS.
- M. D. Konieczynska, J. C. Villa-Camacho, C. Ghobril, M. Perez-Viloria, K. M. Tevis, W. A. Blessing, A. Nazarian, E. K. Rodriguez and M. W. Grinstaff, Angew. Chem., Int. Ed., 2016, 55(34), 9984–9987 CrossRef CAS PubMed.
- T. E. Brown, B. J. Carberry, B. T. Worrell, O. Y. Dudaryeva, M. K. McBride, C. N. Bowman and K. S. Anseth, Biomaterials, 2018, 178, 496–503 CrossRef CAS PubMed.
- B. T. Worrell, S. Mavila, C. Wang, T. M. Kontour, C.-H. Lim, M. K. McBride, C. B. Musgrave, R. Shoemaker and C. N. Bowman, Polym. Chem., 2018, 9(36), 4523–4534 RSC.
- B. T. Worrell, M. K. McBride, G. B. Lyon, L. M. Cox, C. Wang, S. Mavila, C.-H. Lim, H. M. Coley, C. B. Musgrave, Y. Ding and C. N. Bowman, Nat. Commun., 2018, 9(1), 2804 CrossRef PubMed.
- H.-Y. Liu, H. D. Nguyen and C.-C. Lin, Adv. Healthcare Mater., 2018, 7(22), 1800954 CrossRef PubMed.
- Z. Ma, W. Shi, K. Yan, L. Pan and G. Yu, Chem. Sci., 2019, 10(25), 6232–6244 RSC.
- Y.-Z. Zhang, K. H. Lee, D. H. Anjum, R. Sougrat, Q. Jiang, H. Kim and H. N. Alshareef, Sci. Adv., 2018, 4(6), eaat0098 CrossRef PubMed.
- Y. Zhang, Y. Qi, S. Ulrich, M. Barboiu and O. Ramström, Mater. Chem. Front., 2020 10.1039/c9qm00598f.
- Z. Xue, S. Wang, L. Lin, L. Chen, M. Liu, L. Feng and L. Jiang, Adv. Mater., 2011, 23(37), 4270–4273 CrossRef CAS PubMed.
- Y. Gao, J. Song, S. Li, C. Elowsky, Y. Zhou, S. Ducharme, Y. M. Chen, Q. Zhou and L. Tan, Nat.
Commun., 2016, 7, 12316–12316 CrossRef CAS PubMed.
- W. Li, R. Feng, R. Wang, D. Li, W. Jiang, H. Liu, Z. Guo, M. J. Serpe and L. Hu, J. Mater. Chem. B, 2018, 6(29), 4799–4807 RSC.
|
This journal is © The Royal Society of Chemistry 2020 |
Click here to see how this site uses Cookies. View our privacy policy here.