DOI:
10.1039/C9QI00681H
(Research Article)
Inorg. Chem. Front., 2020,
7, 204-211
A facile fabrication of 1D/2D nanohybrids composed of NiCo-hydroxide nanowires and reduced graphene oxide for high-performance asymmetric supercapacitors†
Received
10th June 2019
, Accepted 20th October 2019
First published on 21st October 2019
Abstract
A novel 1D/2D hybrid design is reported for synthesizing a high performance NiCo-hydroxide nanowires/reduced graphene oxide (NiCo-NWs/G) composite. The oxygen-containing groups on the graphene oxide surface can act as anchoring sites for uniformly adsorbing the metal seeds, leading to the dispersed formation of thin NiCo-NWs on the graphene surface. The existence of graphene in NiCo-NWs/G can provide a conductive network to boost the faradaic reactions. In addition, the bimetallic NiCo-NWs possess rich redox reaction sites and the thin nanowire structure ensures that electrons can move rapidly from the reaction sites to the current collectors. Owing to the synergic effects of 1D NiCo-NWs and 2D graphene, the developed NiCo-NWs/G electrode exhibits an excellent performance of 1449 F g−1 at a current density of 1 A g−1, a high rate capability of 61% at 20 A g−1, and a good cycling stability in 6 M KOH electrolyte. Moreover, the asymmetric supercapacitor with NiCo-NWs/G as a positive electrode and active carbon as a negative electrode delivers a high energy density of 42.1 W h kg−1 at a power density of 0.8 kW kg−1. This work may pave a promising new way for the development of 1D/2D composites based on pseudocapacitive hydroxides and carbon materials for high-performance asymmetric supercapacitors.
1. Introduction
The growing demand for electrical energy calls for clean and highly efficient electrochemical energy storage and conversion devices.1,2 Supercapacitors, as a new type of energy storage device, have attracted intensive attention due to their high power density, stable cycling ability, and excellent safety.3 Carbon materials including carbon nanotubes and graphene are the most common materials for supercapacitor electrodes because of their good electrical conductivity, large surface area and excellent stability.4,5 Although excellent power capability is noted for such supercapacitors, their energy density is much lower than that of batteries, which limits their widespread application.6,7 One of the promising approaches to addressing the issue is to use pseudocapacitive materials as positive electrodes and carbon materials as negative electrodes to construct asymmetric supercapacitors (ASCs) for broadening the cell voltage.8,9
It is believed that positive electrode materials play a very important role in the design of ASCs with excellent electrochemical performance. Various positive materials with a pseudocapacitive feature, such as transition metal hydroxides and metal oxides, have been developed for fabricating ASCs, which provide a faradaic type charge transfer process through the surface redox reactions of the electrode with the electrolyte.10–12 Engineering the microstructure of pseudocapacitive materials is also a vitally important strategy to increase the electrochemical performance of ASCs, such as designing one-dimensional (1D) or two-dimensional (2D) nanostructures to facilitate more efficient charge and mass transportations. For instance, Zhang et al.13 demonstrated a facile hydrothermal method to synthesize 1D NiCu(OH)2CO3 which showed a high specific capacitance and good electrochemical stability as a supercapacitor electrode. Pang et al.14 synthesized nickel-cobalt phosphate 2D nanosheets with a specific capacitance of 1132 F g−1 at a current density of 1 A g−1. Among them, 1D and 2D hydroxides, especially the transition bimetallic hydroxides, have received tremendous research interest as promising positive materials for ASCs because of rich redox reaction sites, low cost, and abundant resources.13,15,16 However, some shortcomings of bimetallic hydroxides, such as low electrical conductivity and poor cycling life, still prevent their use as high electrochemical performance electrode materials.
In order to solve these problems, the use of carbon materials to hybridize metallic hydroxides has been regarded as an effective method for improving electrochemical performance.17,18 As an excellent candidate, graphene has received considerable attention in recent years due to its high surface area, excellent electrical conductivity, and tunable chemical properties.19 Owing to its unique 2D structure, graphene can provide an ideal flexible platform with highly accessible functional groups to anchor metal precursors for the growth of metallic compounds. Moreover, the outstanding electronic conductivity of graphene can act as an efficient current-collecting component to facilitate electron transfer.20,21 For instance, Yang et al.10 fabricated NiCo-hydroxide nanosheets/graphene as a supercapacitor electrode showing a specific capacitance of 1489 F g−1 at a current density of 1 A g−1. Graphene was used as the conducting substrate and the surface-confined template for the fabrication of ultrathin NiCo-hydroxide nanosheets. However, the aggregation of graphene sheets due to van der Waals interactions may result in poor electrochemical performance because of the severely decreased specific surface area. It has been reported that the linkage of 1D materials to graphene sheets can increase the basal plane spacing between the graphene layers.20,22 These features make the graphene-based 1D/2D hybrids promising candidates for supercapacitors, electrocatalysis and lithium-based batteries.10,23–25 Therefore, it is an efficient strategy to integrate 1D bimetallic hydroxides and graphene into a heterostructure to combine the advantages of both for achieving high performance ASCs.
Herein, we report a 1D/2D hybrid design to synthesize a novel, high performance NiCo-hydroxide nanowires/reduced graphene oxide (NiCo-NWs/G) composite for ASCs. The bimetallic NiCo-hydroxides possess rich redox reaction sites and their thin nanowire structure ensures that electrons can move rapidly from the reaction sites to the current collectors. Moreover, NiCo-NWs can be used as a “spacer” to mitigate the restacking of graphene for facilitating charge and mass transportations. In addition, the use of graphene oxide (GO) in composite fabrication is also crucial because its surface oxygen-containing functional groups can act as anchoring sites to induce the nucleation and uniform growth of NiCo-NWs on the GO surface. Furthermore, the existence of graphene in NiCo-NWs/G can provide a conductive network to boost the faradaic reaction. Owing to the synergic effects of 1D NiCo-NWs and 2D graphene, the developed NiCo-NWs/G electrode exhibits an excellent performance of 1449 F g−1 at a current density of 1 A g−1, and the asymmetric supercapacitor employing NiCo-NWs/G as a positive electrode and activated carbon as a negative electrode also achieves a high specific capacitance and a stable cycling performance.
2. Experimental
2.1. Preparation of NiCo-NWs/G and NiCo-NWs
Graphene oxide (GO) was first synthesized by the modified Hummers’ method as described in other reports.26,27 1.5 mmol NiCl2·6H2O, 3 mmol CoCl2·6H2O, and 10 mmol urea were dissolved into a 100 mL of GO solution (0.6 mg mL−1 in water) at 100 °C under vigorous stirring for 16 h. The precipitate was filtered with deionized water and freeze-dried to obtain the NiCo-NWs/G. For comparison, NiCo-NWs and graphene were prepared by the same method except for adding GO and metal precursors, respectively.
2.2. Material characterization
The morphologies and structures of the samples were investigated by using a field-emission scanning electron microscope (FESEM, FEI, Nova NanoSEM 450) and a transmission electron microscope (TEM) and scanning TEM (JEM-F200). Nitrogen adsorption–desorption isotherms were measured on a physical sorption instrument (Autosorb-iQ-C). Crystalline properties were examined by using a Shimadzu X-ray diffractometer with Cu Kα radiation. Raman spectra were recorded at 532 nm on a Renishaw inVia Raman Microscope. The Ni and Co contents in the composites were determined by using Inductively Coupled Plasma Spectrometry (ICP, Varian 820). The graphene content was obtained by thermogravimetric analysis (TGA, Mettler-Toledo). The surface chemistry properties of the samples were investigated using the high resolution X-ray photoelectron spectroscopy (XPS, ESCALAB250Xi).
2.3. Electrochemical measurements
The electrochemical performances of the samples were evaluated by using a CHI 760E electrochemistry workstation. The as-prepared samples, carbon black, and binder poly (tetrafluoroethylene) with the mass ratio of 8
:
1
:
1 were mixed together by adding a small amount of ethanol, and after drying at 80 °C, the mixture was rolled into a carbon film. The mass loading of the electrode is about 1.5–2 mg cm−2. For the three-electrode test in 6 M KOH electrolyte, the prepared mixture was pressed onto nickel foam to make the working electrodes; Pt foil was used as the counter electrode, and Hg/HgO electrode was used as the reference electrode. The gravimetric capacitance for a single electrode C (F g−1) was calculated according to galvanostatic charge–discharge profiles.
where I is the discharging current (A), Δt is the discharge time (s), and m is the mass of active material in a single electrode (g).
2.4. Fabrication of the ASC
The ASC was assembled by using NiCo-NWs/G as the positive electrode, home-made AC as the negative electrode and 6 M KOH as the electrolyte. The mass loading of the two electrodes was optimized by the charge balancing equation (q+ = q−). The cyclic voltammetry curves and galvanostatic charge/discharge voltage profiles were recorded in a potential range of 0–1.6 V using a bio-logic electrochemical workstation. The cycling performance was conducted on a LAND CT2001A battery test system. The energy density (E, W h kg−1) and power density (P, W kg−1) were calculated on the basis of the total mass of active materials of the two electrodes according to the equations:
where C is the specific capacitance of the ASC, I is the response current (A), t is the discharge time (s), V is the cell voltage, and m is the weight of total active materials on both electrodes (g).
3. Results and discussion
3.1. Synthesis and characterization of NiCo-NWs/G
The fabrication processes of NiCo-NWs and NiCo-NWs/G are illustrated in Fig. 1. In the absence of GO, the free nucleation and growth of Ni and Co seeds would result in the aggregated structures consisting of thick NiCo-NWs. Nevertheless, when GO is introduced, the oxygen-containing groups on the GO surface can act as anchoring sites for uniformly adsorbing the Ni and Co seeds, leading to the dispersed formation of thin NiCo-NWs on the graphene surface. The inductively coupled plasma spectrometry measured that the Co/Ni molar ratio of the NiCo-NWs and NiCo-NWs/G is 1.86 and 2.01, respectively, which corresponds with the theory ratio of 2.0. Based on thermogravimetric analysis (TGA), the content of NiCo-NWs on the graphene surface is about 94.0 wt% (Fig. S1†), indicating that graphene with a 2D structure is the ideal supporter for the high loading of 1D NiCo-NWs.
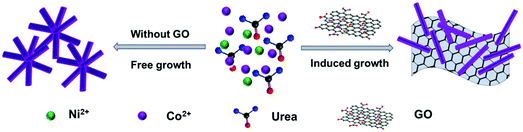 |
| Fig. 1 Schematic illustration of the fabrication routes of NiCo-NWs and NiCo-NWs/G. | |
The field-emission scanning electron microscopy (FESEM) images in Fig. 2a and Fig. S2a, b† show that in the absence of GO, NiCo-NWs aggregate into bulk structures with a diameter of around 50 nm. By contrast, dispersed thin NiCo-NWs (red arrows) supported on the graphene (blue arrows) surfaces can be found when the GO is present as shown in Fig. 2b and Fig. S2c, d.† This indicates that the GO plays an important role where the oxygen-containing groups can effectively adsorb Ni and Co ions uniformly and further tune the formation of NiCo-NWs on the graphene surfaces.
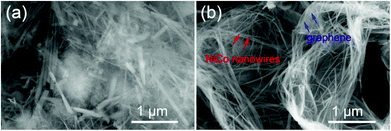 |
| Fig. 2 SEM images of (a) NiCo-NWs; (b) NiCo-NWs/G. | |
The typical X-ray diffraction (XRD) patterns of the NiCo-NWs and NiCo-NWs/G are shown in Fig. 3. The diffraction peaks of the NiCo-NWs at 11.8°, 23.9°, 34.0°, 38.8° and 59.2° correspond to (003), (006), (012), (015), and (110) plane reflections of two-dimensional hydrotalcite-like materials (JCPDS card no.54-1030).28 After introducing GO, the crystal structure of the NiCo-NWs on graphene is almost unchanged. It is also noted that the diffraction peak at 10.7° corresponding to GO (Fig. S3†) in the NiCo-NWs/G disappears completely after the refluxing reaction, indicating that GO is reduced in the presence of urea and the generated graphene is in the amorphous state.29 Raman spectra of graphene reduced from GO by urea without the metal salts and NiCo-NWs/G in Fig. S4† show two typical peaks of carbon materials at ca. 1345 cm−1 (D band) and 1590 cm−1 (G band). Compared with the reduced graphene sheets, the ID/IG ratio of the NiCo-NWs/G is increased from 1.03 to 1.18, indicating that there are more structural defects after the anchoring of NiCo-NWs on the graphene surface.
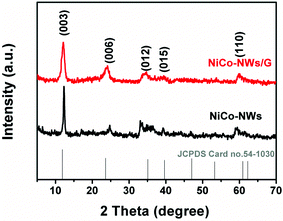 |
| Fig. 3 XRD patterns of NiCo-NWs and NiCo-NWs/G. | |
Transmission electron microscopy (TEM) and scanning TEM (STEM) were used to further investigate the morphology and structure of NiCo-NWs/G, the images of which are shown in Fig. 4. It is found in Fig. 4a that the NiCo-NWs (red arrows) are dispersed on the graphene (blue arrows) surface with a diameter range of ca. 15–30 nm, which is consistent with the SEM images. The lattice fringes of a typical nanowire with a spacing of ca. 0.26 nm can be distinctly observed in a high-resolution TEM image (Fig. 4b), which can be indexed to the (012) plane of the NiCo-NWs phase. The STEM and corresponding elemental mapping images (Fig. 4c) further demonstrate the uniform distribution of NiCo-NWs on the graphene surface, confirming the strong coupling interaction between the NiCo-NWs and the graphene sheets.
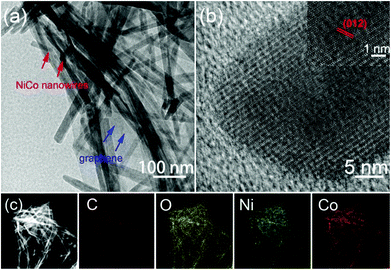 |
| Fig. 4 TEM images of (a, b) NiCo-NWs/G (inset: the lattice image of a single NiCo-NW). (c) STEM image of NiCo-NWs/G, and the corresponding elemental mapping of C; O; Ni; Co. | |
X-ray photoelectron spectroscopy (XPS) was performed to obtain information on the components and the surface electronic states of NiCo-NWs and NiCo-NWs/G. It can be clearly seen that the C, O, Co, and Ni elements are identified in Fig. S5,† which are the main components of NiCo-NWs and NiCo-NWs/G. The Ni 2p and Co 2p spectra are well fitted with two spin–orbit doublets and two shakeup satellites, as shown in Fig. 5. For NiCo-NWs, the peaks (Fig. 5a) for Co 2p1/2 and Co 2p3/2 located at 797.4 eV and 781.5 eV with a spin-energy separation of 15.9 eV indicate the presence of Co2+.30 Similarly, the peaks (Fig. 5b) at 873.8 eV for Ni 2p1/2 and 856.2 eV for Ni 2p3/2 indicate the existence of Ni2+.31 It is interesting to find that all the Co 2p and Ni 2p peaks of NiCo-NWs/G shift to a lower binding energy in comparison with the peaks of NiCo-NWs (ca. 0.2 eV for Co 2p and ca. 0.4 eV for Ni 2p). The negative shift of the metal peaks implies the strong interactions between NiCo-NWs and graphene.28 These results show that the 1D NiCo-NWs are bridged onto 2D graphene via a strong coupling interactions, which is beneficial for charge transfer.
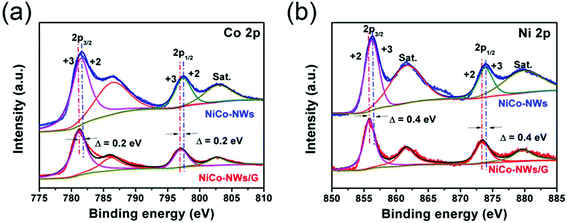 |
| Fig. 5 High-resolution XPS spectra (a) Co 2p and (b) Ni 2p of NiCo-NWs and NiCo-NWs/G. | |
The pore structures of the NiCo-NWs and NiCo-NWs/G were analyzed by nitrogen adsorption–desorption measurements (Fig. S6†). The Brunauer–Emmett–Teller (BET) surface area of NiCo-NWs/G is 87.8 m2 g−1, which is higher than that of NiCo-NWs (48.7 m2 g−1). This comparison indicates that the presence of graphene can increase the BET surface area due to the loose architecture of graphene and the dispersed NiCo-NWs on the graphene surface. The increased surface area of NiCo-NWs/G can effectively facilitate fast penetration of the electrolyte into the electrode material, thus achieving good capacitive performance.
3.2 Electrochemical performance of the NiCo-NWs and NiCo-NWs/G
The electrochemical performance of the NiCo-NWs and NiCo-NWs/G electrodes was first evaluated in a three-electrode cell in 6 M KOH aqueous electrolyte. Fig. 6a compares the electrochemical performances of NiCo-NWs and NiCo-NWs/G by cyclic voltammetry (CV) curves at the scan rate of 5 mV s−1. Both the CV curves exhibit pairs of broad redox couple peaks, which can be attributed to the reversible faradaic redox reactions related to Ni2+/Ni3+ and Co2+/Co3+.32 Obviously, NiCo-NWs/G exhibits a higher specific capacity with larger CV areas compared to that of NiCo-NWs without graphene, indicating its higher specific capacitance. When increasing the scan rate to 100 mV s−1 (Fig. 6b), the CV curve of the NiCo-NWs/G electrode has increased intense redox peaks, suggesting an improved rate capability in contrast to a poorly performing NiCo-NWs electrode. Fig. 6c shows the galvanostatic charge–discharge (GC) curves of the samples at a current density of 2 A g−1. Both NiCo-NWs and NiCo-NWs/G show significant deviations from the linear curves, indicating that the capacitance mainly comes from the faradaic redox reactions.33 This is consistent with the CV curves. The specific capacitances of NiCo-NWs and NiCo-NWs/G calculated based on the GC curves are shown in Fig. 6d. The NiCo-NWs/G electrode yields an excellent specific capacitance of 1449 F g−1 at 1 A g−1, which is higher than 1070 F g−1 of the NiCo-NWs. It should be noted that higher capacitance retention can be achieved for NiCo-NWs/G (61%) at a high current density of 20 A g−1, compared with the capacitance retention of only about 30% for the NiCo-NWs electrode. The electrochemical performance of NiCo-NWs/G is competitive in comparison with previous reports (Table S1†).10,20,34–39 The charge transport characteristics of the electrodes were measured by electrochemical impedance spectroscopy (EIS) in the frequency range of 0.01 Hz–100 kHz (Fig. 6e). It can be seen that the diameter of the semicircle in the high-frequency range for the NiCo-NWs/G is smaller than that for NiCo-NWs, indicating a relatively low charge-transfer resistance. As an important characteristic for high-performance supercapacitors, the cycling stability of the NiCo-NWs and NiCo-NWs/G electrodes was further evaluated at a current density of 10 A g−1. Fig. 6f shows that the specific capacitance of the NiCo-NWs/G electrode can maintain ca. 94% of the initial capacitance after 10
000 cycles while capacitance retention is only ca. 68% of the NiCo-NWs after 2000 cycles. The possible reason behind this is that the open channels generated by the synergistic effect of 1D “spacer” NiCo-NWs and 2D conductive graphene within the electrode matrix provide more redox reaction sites and fast transport pathways for the electrolyte ions to the active material surface. Another possible reason is that the thin NiCo-NWs on the graphene ensure that electrons move rapidly from the reaction sites to the current collectors.
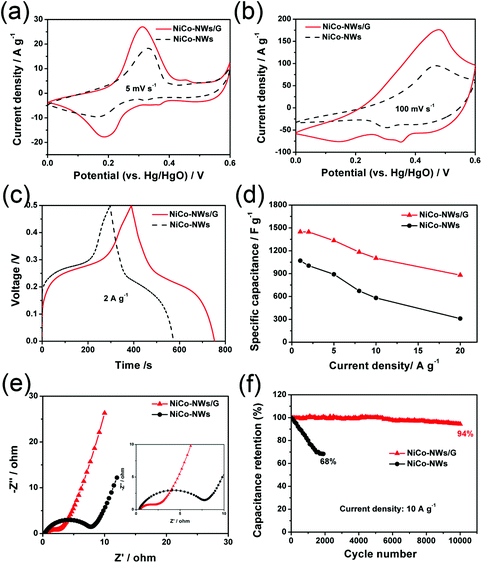 |
| Fig. 6 Electrochemical performance of NiCo-NWs and NiCo-NWs/G for supercapacitors: (a, b) CV curves at different scan rates of 5 and 100 mV s−1; (c) galvanostatic charge–discharge curves at a current density of 2 A g−1; (d) specific capacitances at different current densities; (e) Nyquist plots; (f) cycling performance of different samples at a current density of 10 A g−1. | |
Moreover, a series of NiCo-NWs/G samples with different reaction times were also fabricated. Samples are labeled as NiCo-NWs/G-4, NiCo-NWs/G-8 and NiCo-NWs/G-24 for the reaction times of 4 h, 8 h and 24 h, respectively. As shown in Fig. S7,† it can be found that the NiCo-NWs are dispersed on the graphene surface for all the samples. The length of NiCo-NWs on NiCo-NWs/G-4 is shorter than others, which may be due to incomplete reaction in a short time. The electrochemical performance of the NiCo-NWs/G-based electrodes was also investigated. The pairs of broad redox couple peaks in the CV curves and the non-linearity of GC curves indicate that the capacitances of the samples mainly comes from the faradaic redox reactions (Fig. S8a and b†). It can be seen that the as-made NiCo-NWs/G sample also shows the highest specific capacitance at the current density of 1 A g−1 in comparison with other samples (Fig. S8c†). The NiCo-NWs/G-4 shows the lowest specific capacitance, but the highest capacitance retention of 78% can be obtained at 20 A g−1, which may be a result of the low content of formed NiCo-NWs in such a short reaction time. All the samples can maintain above 90% of the initial capacitance after 10
000 cycles, suggesting that the introduction of graphene can improve cycling stability significantly (Fig. S8d†).
To further evaluate the real applications of the as-synthesized NiCo-NWs/G for supercapacitors, an asymmetric supercapacitor has been assembled by utilizing the as-prepared NiCo-NWs/G as the positive electrode, home-made active carbon (AC) as the negative electrode and 6 M KOH as the electrolyte. As shown in Fig. S9,† the potential window of the NiCo-NWs/G electrode is in the voltage range of 0–0.6 V while that of the AC electrode is in the voltage range of −1.0–0 V at a scan rate of 10 mV s−1. Therefore, the NiCo-NWs/G//AC asymmetric supercapacitors can be operated in a stable electrochemical window of 1.6 V without significant polarization. The CV curves of the ASC at scan rates from 5 to 30 mV s−1 are shown in Fig. 7a, exhibiting the quasi-rectangular geometry combination of both pseudocapacitance and electric double-layer capacitance. The GC curves of NiCo-NWs/G//AC also suggest the coexistence of electrostatic and redox reactions in the ASC (Fig. 7b). The specific capacitances calculated from GC curves at different current densities are shown in Fig. 7c, the NiCo-NWs/G//AC yields a high specific capacitance of ca. 118 F g−1 at a current density of 1 A g−1, and a capacitance retention of 46% can be obtained with a 10-fold increase in current density. The Ragone plot in Fig. 7d shows that a high energy density of 42.1 W h kg−1 can be achieved for NiCo-NWs/G//AC at a power density of 0.8 kW kg−1, and the energy density can still remain at 19.1 W h kg−1 at a power density of 8.0 kW kg−1. The cycling performance of the NiCo-NWs/G//AC asymmetric supercapacitor was also evaluated at the current density of 10 A g−1. Fig. 6e shows that the NiCo-NWs/G//AC can retain ca. 79% of the initial specific capacitance after 3000 cycles, indicating good cycling stability. In addition, the assembled NiCo-NWs/G//AC cell can lighten up six LED indicators, indicating an excellent energy storage capacity (Fig. S10†).
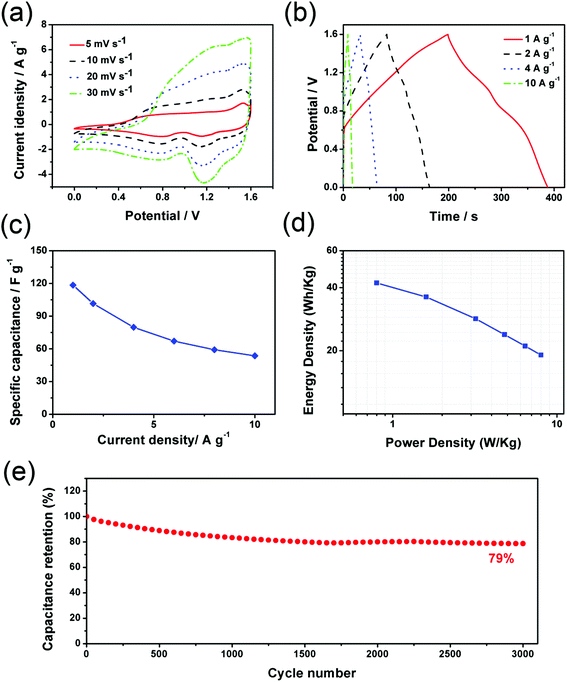 |
| Fig. 7 Electrochemical performance of the as-fabricated NiCo-NWs/G//AC asymmetric supercapacitor: (a) CV curves at scan rates from 5 to 30 mV s−1; (b) galvanostatic charge/discharge curves at various current densities of 1–10 A g−1; (c) the corresponding specific capacitance at different current densities; (d) the Ragone plots of the as-fabricated NiCo-NWs/G//AC; (e) cycling performance at a current density of 10 A g−1. | |
4. Conclusions
In summary, we have developed a 1D/2D hybrid design to synthesize a NiCo-NWs/G composite for supercapacitors. GO can function as anchoring sites to induce the nucleation and uniform growth of NiCo-NWs, and the existence of graphene in NiCo-NWs/G can provide a conductive network to boost the faradaic reaction. In addition, the bimetallic NiCo-NWs possess rich redox reaction sites and can mitigate the restacking of graphene for facilitating the charge and mass transportations. Benefiting from the synergic effects of 1D NiCo-NWs and 2D graphene, the developed NiCo-NWs/G electrode exhibits an excellent performance of 1449 F g−1 at a current density of 1 A g−1 and a good rate capability of 881 F g−1 at 20 A g−1. Moreover, an ASC cell based on the NiCo-NWs/G positive electrode and an AC negative electrode delivers a high energy density of 42.1 W h kg−1 at a power density of 0.8 kW kg−1. This work may provide an innovative strategy to prepare 1D/2D composites for high-performance asymmetric supercapacitors.
Conflicts of interest
The authors declare no competing financial interest.
Acknowledgements
This work is supported by the National Science Foundation of China (51802251), the National Key Research & Development Program (2018YFB0604604), the China Postdoctoral Science Foundation (2018M631168 and 2019M651118), the Natural Science Foundation of Liaoning Province (2019-ZD-0020), the Doctoral Start-up Foundation of Liaoning Province (20170520263) and the Fundamental Research Funds for the Central Universities (DUT18RC(4)059 and DUT19JC54).
References
- L. Yu, H. Wu and X. Lou, Acc. Chem. Res., 2017, 50, 293–301 CrossRef CAS.
- L. Jiang, L. Sheng and Z. Fan, Sci. China Mater., 2018, 61, 133–158 CrossRef CAS.
- R. R. Salunkhe, Y. V. Kaneti, J. Kim, J. H. Kim and Y. Yamauchi, Acc. Chem. Res., 2016, 49, 2796–2806 CrossRef CAS.
- X. Shi, S. Zheng, Z. Wu and X. Bao, J. Energy Chem., 2018, 27, 25–42 CrossRef.
- X. Tang, Y. Lui, B. Chen and S. Hu, J. Power Sources, 2017, 352, 118–126 CrossRef CAS.
- Y. Zhao, N. Jiang, X. Zhang, J. Guo, Q. Yang, L. Gao, Y. Li and T. Ma, J. Alloys Compd., 2018, 765, 396–404 CrossRef CAS.
- R. Zhao, M. Wang, D. Zhao, H. Li, C. Wang and L. Yin, ACS Energy Lett., 2018, 3, 132–140 CrossRef CAS.
- H. Hu, B. Guan and X. Lou, Chem, 2016, 1, 102–113 CAS.
- W. Guo, C. Yu, S. Li, J. Yang, Z. Liu, C. Zhao, H. Huang, M. Zhang, X. Han, Y. Niu and J. Qiu, Small, 2017, 13, 1701288 CrossRef.
- J. Yang, C. Yu, C. Hu, M. Wang, S. Li, H. Huang, K. Bustillo, X. Han, W. Guo, Z. Zeng, H. Zheng and J. Qiu, Adv. Funct. Mater., 2018, 28, 1803272 CrossRef.
- J. Liu, M. Zheng, X. Shi, H. Zeng and H. Xia, Adv. Funct. Mater., 2016, 26, 919–930 CrossRef CAS.
- J. Yang, C. Yu, S. Liang, S. Li, H. Huang, X. Han, C. Zhao, X. Song, C. Hao, P. M. Ajayan and J. Qiu, Chem. Mater., 2016, 28, 5855–5863 CrossRef CAS.
- X. Zheng, Y. Ye, Q. Yang, B. Geng and X. Zhang, Chem. Eng. J., 2016, 290, 353–360 CrossRef CAS.
- B. Li, P. Gu, Y. Feng, G. Zhang, K. Huang, H. Xue and H. Pang, Adv. Funct. Mater., 2017, 27, 1605784 CrossRef.
- Q. Gui, J. Jiang, Y. Li and J. Liu, Dalton Trans., 2018, 47, 15416–15423 RSC.
- W. Zou, W. Guo, X. Liu, Y. Luo, Q. Ye, X. Xu and F. Wang, Chem. – Eur. J., 2018, 24, 19309–19316 CrossRef CAS.
- K. Zhou, W. Zhou, L. Yang, J. Lu, S. Cheng, W. Mai, Z. Tang, L. Li and S. Chen, Adv. Funct. Mater., 2015, 25, 7530–7538 CrossRef.
- J. Yang, C. Yu, X. Fan and J. Qiu, Adv. Energy Mater., 2014, 4, 1400761 CrossRef.
- X. Zhang, Q. Fan, Y. Zhao, M. Wang, Y. Meng, Y. Xiao, X. Lei and J. Yang, Sustainable Energy Fuels, 2018, 2, 2494–2501 RSC.
- J. Yang, C. Yu, X. Fan, C. Zhao and J. Qiu, Adv. Funct. Mater., 2015, 25, 2109–2116 CrossRef CAS.
- Y. Zhu, S. Murali, W. Cai, X. Li, J. Suk, J. R. Potts and R. S. Ruoff, Adv. Mater., 2010, 22, 3906–3924 CrossRef CAS.
- S. C. G. Gnana Kumar, T. Raj Kumar and A. Manthiram, ACS Appl. Mater. Interfaces, 2018, 10, 20627–20634 CrossRef PubMed.
- H. Yang, X. Zhang, W. Zhu, F. Wang, Y. Li, Q. Fan, H. Xiao and F. Zhang, ChemElectroChem, 2019, 6, 1115–1121 CrossRef CAS.
- L. Yang, Y. Lv and D. Cao, J. Mater. Chem. A, 2018, 6, 3926–3932 RSC.
- S. Huang, L. Zhang, J. Wang, J. Zhu and P. K. Shen, Nano Res., 2018, 11, 1731–1743 CrossRef CAS.
- X. Zhang, Y. Zhou, C. Wang and J. Qiu, New Carbon Mater., 2016, 31, 407–414 Search PubMed.
- N. I. Kovtyukhova, P. J. Ollivier, B. R. Martin, T. E. Mallouk, S. A. Chizhik, E. V. Buzaneva and A. D. Gorchinskiy, Chem. Mater., 1999, 11, 771–778 CrossRef CAS.
- S. Li, C. Yu, J. Yang, C. Zhao, M. Zhang, H. Huang, Z. Liu, W. Guo and J. Qiu, Energy Environ. Sci., 2017, 10, 1958–1965 RSC.
- N. Qu, X. Zhang, Q. Fan and H. Yang, Mater. Res. Express, 2019, 6, 085606 CrossRef.
- D. Zhang, H. Wang, G. Chen, H. Wan, N. Zhang, X. Liu and R. Ma, Nanoscale, 2019, 11, 6165–6173 RSC.
- Y. Zhao, N. Jiang, X. Zhang, J. Guo, Y. Li, L. Gao, H. Wang and T. Ma, Mater. Chem. Phys., 2018, 217, 291–299 CrossRef CAS.
- Z. Jiang, Z. Li, Z. Qin, H. Sun, X. Jiao and D. Chen, Nanoscale, 2013, 5, 11770–11775 RSC.
- X. Chen, M. Cheng, D. Chen and R. Wang, ACS Appl. Mater. Interfaces, 2016, 8, 3892–3900 CrossRef CAS PubMed.
- H. D. Lim, B. K. Kang, S. N. Tiruneh and D. H. Yoon, Sci. Adv. Mater., 2017, 9, 1241–1247 CrossRef CAS.
- X. Cai, X. Shen, L. Ma, Z. Ji, C. Xu and A. Yuan, Chem. Eng. J., 2015, 268, 251–259 CrossRef CAS.
- L. Jiang, Y. Sui, J. Qi, Y. Chang, Y. He, Q. Meng, F. Wei, Z. Sun and Y. Jin, Appl. Surf. Sci., 2017, 426, 148–159 CrossRef CAS.
- K. Qin, L. Wang, S. Wen, L. Diao, P. Liu, J. Li, L. Ma, C. Shi, C. Zhong, W. Hu, E. Liu and N. Zhao, J. Mater. Chem. A, 2018, 6, 8109–8119 RSC.
- H. Ma, J. He, D.-B. Xiong, J. Wu, Q. Li, V. Dravid and Y. Zhao, ACS Appl. Mater. Interfaces, 2016, 8, 1992–2000 CrossRef CAS.
- B. Mehrabimatin, E. P. Gilshteyn, M. E. M. Buan, O. Sorsa, H. Jiang, A. I. Zad, S. Shahrokhian, A. G. Nasibulin and T. Kallio, Energy Technol., 2019, 7, 1801002 CrossRef.
Footnote |
† Electronic supplementary information (ESI) available. See DOI: 10.1039/c9qi00681h |
|
This journal is © the Partner Organisations 2020 |
Click here to see how this site uses Cookies. View our privacy policy here.