DOI:
10.1039/C9RA06468K
(Paper)
RSC Adv., 2020,
10, 2634-2645
Comparative assessment of physicochemical and antioxidative properties of mung bean protein hydrolysates
Received
18th August 2019
, Accepted 6th January 2020
First published on 14th January 2020
Abstract
Two commercial plant proteases namely ficin and bromelain, were acquired to hydrolyze mung bean protein over 300 min hydrolysis, and the physicochemical and antioxidative properties of the obtained hydrolysates were investigated. Bromelain-treated mung bean protein hydrolysates presented a higher degree of hydrolysis in comparison with ficin-treated hydrolysates, further modifying their physicochemical and emulsifying properties. All mung bean protein hydrolysates exhibited 50% scavenging of DPPH radical (IC50) in the concentration range from 8.67 to 16.22 μg mL−1. Our results also showed that strong metal ion-chelating activity was found in the ficin- (higher activity) and bromelain-treated protein hydrolysates. In addition, oxidative stability of linoleic acid was significantly enhanced by two selected protein hydrolysates, particularly the bromelain-treated hydrolysate with the highest inhibition effect of linoleic acid oxidation (94.55 ± 0.10%). Interestingly, both of these two hydrolysates could effectively retard lipid oxidation of sunflower oil and sunflower oil-in-water emulsion, while the ficin-treated hydrolysate showed slightly better performance. Therefore, mung bean protein hydrolysates showed potential to inhibit lipid oxidation, which could be advantageous in the food industry for producing fortified food.
1. Introduction
With the worsening of environmental pollution, environmental-friendly foods have been attracting a lot of well-deserved attention lately. Tilman and Clark1 found that ruminant meats have greenhouse gas emissions per gram of protein about 250 times those of legumes. Given that animal-based foods contribute to more negative environmental impacts than plant-based foods, protein from legume sources is a wise alternative to replace protein from animal sources.2,3 Mung bean (Vigna radiata L.) is a leguminous seed with a protein content ranging from 20 to 33%, which is significantly higher than the levels found in cereal grains and conventional root crops.4 The protein in mung bean contains plenty of essential amino acids, comparing favorably with that of FAO/WHO reference protein.5 Accordingly, mung bean protein is recognized as a replacement for animal proteins in product formulations.
In addition to the fundamental nutritional role, beans protein may be capable to produce varied peptide sequences with specific biological properties.6 Since legume protein are more economical and practical in comparison with animal proteins,7 legume-derived peptides are attracting wide attention and interest of researchers for producing antioxidant peptides. For instance, Evangelho et al.8 found that black bean protein treated with alcalase digestion showed strong activities on scavenging free radicals and enhancing emulsion stability. Reports over the past decade also evidenced that protein hydrolysates from other legume like pea, Phaseolus lunatus and Phaseolus vulgaris seeds7,9 could effectively scavenge free radicals and chelate metal ion, presenting their excellent antioxidant capacity. Generally speaking, the antioxidant peptides remain latent as inactive sequences within their parent proteins and are released by proteolysis. Therefore, the antioxidant capacity of protein hydrolysates is mainly based on the extent of hydrolysis, which in turn plays a crucial role in their physicochemical properties.
Enzymatic hydrolysis is widely recognized to possess the capacity of translating proteins into free amino acids, peptides or polypeptides, yielding the hydrolysate with varying physicochemical properties.10 These properties are directly responsible for the action and bioactivity of protein hydrolysates when they interact with other components of food such as oil and water.11 In a similar way, physicochemical properties of the resulting hydrolysate are greatly affected by hydrolysis conditions and protease employed. Enzyme type generally dictates the cleavage patterns of the peptide bonds, thereby impacting the properties of resultant hydrolysate. Commercial enzymes ficin and bromelain were reported to produce protein hydrolysates with varied physicochemical properties and different antioxidant activity, such as the reported in fresh water carp protein hydrolysates,12 egg-white protein hydrolysates,13 and peptide hydrolysates from deer, sheep, pig and cattle red blood cell fractions.14 However, to date, limited consideration has been given to the systematic and comparative influence of protease specificity and reaction conditions on physicochemical and antioxidant properties of the resulting hydrolysates.
Taken all together, hydrolysis involved in enzyme types play a determinant role in the physicochemical and antioxidative characteristics of protein hydrolysates. Cysteine proteases ficin and bromelain from fig and pineapple respectively, are currently well-known plant proteases used in food processing. Nevertheless, the influences of these two cysteine proteases on the physicochemical properties and antioxidative activity of mung bean protein hydrolysates remain unclear. Therefore, this work aimed at investigating not only the physicochemical changes of mung bean protein hydrolyzed by ficin and bromelain at different hydrolysis time, but also their abilities on scavenging radicals and chelating metal ion. To further confirm their antioxidant activity and practicability, the oxidative stability of lipid products such as linoleic acid, sunflower oil and sunflower oil-in-water emulsion was assessed with the addition of the corresponding hydrolysates.
2. Materials and methods
2.1 Materials
Mung bean was obtained from Shiyuedaotian Co. Ltd (Beijing, China). Ficin and bromelain were purchased from J&K Scientific Co. Ltd (Shanghai, China). Sunflower oil (Arawana Brand, Jiali Food Ltd., Shanghai, China) and corn oil (Longevity Flower Co., Ltd., Jinan, Shandong, China) were purchased from local supermarket in China. Dithiothreitol (DTT), 8-anilino-1-naphthalenesulfonic acid (ANS) and linoleic acid were purchased from Macklin Co. Ltd. (Shanghai, China). 1,1-Diphenyl-2-picrylhydrazyl radical 2,2-diphenyl-1-(2,4,6-trinitrophenyl)hydrazyl (DPPH) and ferrozine were purchased from Sigma-Aldrich. All other chemicals used were of analytical reagent grade.
2.2 Preparation of mung bean protein isolate
Mung bean protein isolate was prepared according to the method of alkaline extraction and acid precipitation.15 Briefly, the defatted mung bean flour was suspended in water (1
:
8 flour/water, w/v) and adjusted to pH 9.0 with 15 mM NaOH. After stirring for 60 min at room temperature, the sample was centrifuged at 10
000 × g for 15 min at 4 °C in a centrifuge (ThermoFisher, Germany). The supernatant was collected and adjusted to pH 4.0 with 2 M HCl, and then centrifuged at 10
000 × g for 10 min. The precipitation was washed with distill water and lyophilized.
2.3 Preparation of mung bean protein hydrolysates
Mung bean protein powder was treated with pilot-scale Short-wave Infrared Radiation equipment (Senttech Infrared 94 Technology Co. Ltd, Taizhou, China) at 100 °C for 20 min. According to our previous study,16 after cooling down at room temperature, sample was dispersed in deionized water to obtain a 10% solution (w/v), which was divided into two aliquots. One aliquot was incubated with ficin (2%, w/w) at pH 5.7 and 65 °C, and the second one was incubated with bromelain (2%, w/w) at pH 7.0 and 55 °C. The respective control was prepared under the same incubation conditions without enzyme addition. One aliquot was collected every 60 min and heated at 90 °C for 15 min to inactive the enzymes. After cooling down at room temperature, the pH of sample was adjusted to neutral. Finally, the solutions were centrifuged at 10
000 × g for 10 min and the supernatants were collected and lyophilized.
2.4 Determination of degree of hydrolysis
Degree of hydrolysis (DH) was determined using the o-phthaldialdehyde (OPA) method as described by Nielsen et al.17 Briefly, 1.5 mL OPA reagent and 200 μL sample (or control) were mixed and incubated at room temperature for exactly 2 min. Then the absorbance of the mixture was measured at 340 nm in a UV-visible spectrophotometer (GE Healthcare, USA).
2.5 SDS-PAGE electrophoresis
SDS-PAGE was carried out to estimate the molecular weight profiles of protein and hydrolysates by using the SDS-PAGE Preparation kit (Sangon Biotech, Shanghai, China). Protein sample and loading buffer (Beyotime, Shanghai, China) were mixed in a 4
:
1 ratio and incubated in boiling water bath for 10 min. Aliquots of 10 μL were loaded into the gels (5% stacking gel and 15.5% separating gel). The electrophoresis was performed in a Mini-PROTEAN Tetra Cell system (Bio-Rad, USA).
2.6 Structural characterization
2.6.1 Ultraviolet-visible spectroscopy. The sample was diluted with deionized water, and the ultraviolet-visible spectrum of mung bean protein or hydrolysate was measured over the wavelength range from 200 to 800 nm by a UV-visible spectrophotometer (GE Healthcare, USA).
2.6.2 Relative fluorescence intensity. The relative fluorescence intensity of samples was conducted as described by Evangelho et al.8 with slight modification. Sample was dissolved in 10 mM phosphate buffer (pH 7.0) to get the concentration of 1 μg mL−1. 1.5 mL solution was mixed with 20 μL of 8-anilino-1-naphthalenesulfonic acid (ANS) stock solution (8 mM). The fluorescence intensity of the mixture was measured using a spectrofluorometer (F-7000, Hitachi, Japan) at the wavelength ranges of 400–700 nm with excitation at 390 nm, conducted at 20 °C with a constant slit of 5 nm for excitation and emission.
2.6.3 Circular dichroism (CD) spectroscopy. The structure changes of protein and hydrolysates were analyzed in the wavelengths from 185 to 265 nm by Chirascan Circular Dichroism Spectrometer (Applied Photophysics Ltd., UK). Sample was diluted with ultrapure water to get the concentration of 0.1 mg mL−1 and placed into the quartz cell with a path length of 1 mm. On the basis of three scans, CD spectrum was expressed as ellipticity, θ (mdeg).
2.7 Surface charge (ζ-potential)
According to the method of Teh et al.,18 ζ-potential of protein and hydrolysates was measured by using a Zetasizer Nano ZS (Malvern Instruments, Malvern, UK). An aliquot (1.4 mL) of each sample was added into a visibly clear disposable zeta cell without any air bubbles. The equilibrium time was 1 min.
2.8 Emulsifying properties
The emulsifying activity index (EAI) and emulsion stability index (ESI) of mung bean protein hydrolysates were measured by the method of Suppavorasatit et al.19 3 mL of sample (2 mg mL−1) and 1 mL of corn oil were homogenized at 20
000 rpm for 60 s using IKA T25 homogenizer (Staufen, Germany). A 25 μL aliquot of emulsion was immediately mixed with 2.5 mL of SDS solution (0.1%, w/v) and 60 min after homogenization. The absorbance of the mixture was measured at 500 nm by a UV-visible spectrophotometer (GE Healthcare, USA).
2.9 DPPH radical scavenging activity
DPPH radical scavenging activity of protein hydrolysates were determined according to the method of Li et al.20 with a slight modification. Briefly, two-fold serial dilutions of protein hydrolysates were prepared, 50 μL aliquot was mixed with 50 μL of 95% ethanol containing 0.1 mM DPPH. The mixture was allowed to stand in darkness for 30 min, and the absorbance was measured at 517 nm with a microplate reader (SpectraMax 190, Molecular Devices, USA).
2.10 Metal-chelating activity
Metal-chelating activity was tested on basis of a previous work.21 Two-fold serial dilutions of protein hydrolysates (50 μL) were prepared in a 96-well plate and mixed with 100 μL of 20 μM FeCl2. Then, the mixture was incubated with 100 μL of 0.5 mM ferrozine at room temperature for 10 min. The control used was deionized water. Absorbance of the resulting solution was measured at 562 nm with a microplate reader (SpectraMax 190, Molecular Devices, USA).
2.11 Inhibition of lipid oxidation
2.11.1 Measurement of antioxidant activity in linoleic acid. Ferric thiocyanate method was used to detect the antioxidative effects of protein hydrolysates in a linoleic acid model system.22 1 mL of 2.5% linoleic acid in ethanol was mixed with 1 mL hydrolysate (50 μg mL−1) and 2 mL of 0.05 M phosphate buffer (pH 7.0) and kept in a storage bottle with a screw cap under dark condition at 60 °C. One aliquot was collected every day for measuring the degree of oxidation by using the ferric thiocyanate method. Briefly, 5 μL of this aliquot was mixed with 235 μL of 75% ethanol, 5 μL of 30% (w/v) ammonium thiocyanate, and 5 μL of 0.02 M ferrous chloride solution in 3.5% HCl. After 3 min, the OD (optical density) at 500 nm for the color solution was measured by a microplate reader. 0.05 M phosphate buffer (pH 7.0) instead of hydrolysate was used as control. Higher OD value means higher degree of linoleic acid oxidation.
2.11.2 Measurement of antioxidant activity in sunflower oil. The ability of protein hydrolysates to inhibit lipid oxidation was firstly measured by using an 892 Professional Rancimat Instrument (Metrohm, Switzerland). 4.0 g of sunflower oil was added into glass test tube, followed by the addition of 0.5% (w/w) sample. Sunflower oil without any addition was used as control. After mixed, sunflower oil was placed into rancimat instrument under constant airflow of 20 L h−1 and heated temperature of 120 ± 1.6 °C. The induction period of sunflower oil was measured, and this experiment was conducted at least in duplicate.
2.11.3 Measurement of antioxidant activity in oil-in-water emulsion. The ability of protein hydrolysates to inhibit lipid oxidation was then evaluated in the oil-in-water (O/W) emulsion. In brief, 30% sunflower oil, 1% Tween 20, and 69% ultrapure water were used to prepare O/W emulsion. The mixture was first homogenized in an Ultra-Turrax homogenizer (IKA, Ultra-Turrax® T25-digital, Staufen, Germany) at 12
000 rpm for 2 min, and then emulsified by a two-stage high-pressure homogenizer (150/50 bar) with an AH-2010 homogenizer (ATS Engineering Inc., Ontario, Canada). Protein hydrolysate was added into emulsion in a final protein concentration of 400 μg mL−1. According to the method of Caetano-Silva et al.,23 the malonaldehyde (MDA) production in emulsion was measured on days 0, 1, 2, 4, 6 and 8. 1.5 mL emulsions were incubated with 1 mL of 1% thiobarbituric acid (w/v) and 2.5 mL of 10% trichloroacetic acid (w/v) for 30 min in a boiling water bath. After cooled down at room temperature, 2.5 mL of solution was mixed with equivalent volume of trichloromethane, and centrifuged at 3000 × g for 10 min. The absorbance of supernatants at 532 nm were measured in a plate reader (SpectraMax 190, Molecular Devices, USA). Moreover, the microstructure of emulsion was observed by using a polarized light microscope (Leica, Germany) installed with a Leica DFC450 video camera.
2.12 Statistical analysis
All experiments were carried out at least in duplicate, and results were expressed as mean ± standard deviation. One-way analysis of variance (ANOVA) combined with Student's t test were conducted by using Graphpad prism 7 (Graphpad Software Inc., San Diego, USA). The resulting data were analyzed graphically using OriginPro 2016 (OriginLab, Northampton, MA).
3. Results and discussion
3.1 Degree of hydrolysis (DH)
The mung bean protein was subjected to hydrolysis by ficin and bromelain under different time, and DH were presented in Fig. 1A and B, respectively. Compared with the slight changed DH values of control without ficin or bromelain digestion (P < 0.05), hydrolysis progress with two plant proteases increased in a time-dependent manner. This indicated pH and temperature did not provide any significant influence on DH of protein, corresponding to the observation by Evangelho et al.8 In terms of enzymatic hydrolysis, bromelain promoted a rapid growth in the DH during the first 5 h of reaction, achieving the value of 15.04 ± 0.33%. Similar trend was also found in the ficin hydrolysis, which presented much lower rate of hydrolysis on the mung bean protein. The difference for the DH by ficin and bromelain might be accounted for partly by protein conformation, which contains less cleavage sites for ficin to act on (Fig. 1C). On the other hand, the DH difference might result in the varied structural and functional properties of ficin and bromelain hydrolysates. Overall, both of mung bean protein hydrolysates obtained from ficin and bromelain reached the highest DH values (11.45 ± 0.02% and 15.04 ± 0.33%, respectively) after 300 min of hydrolysis. Therefore, mung bean proteins hydrolyzed for up to 300 min were used for further study.
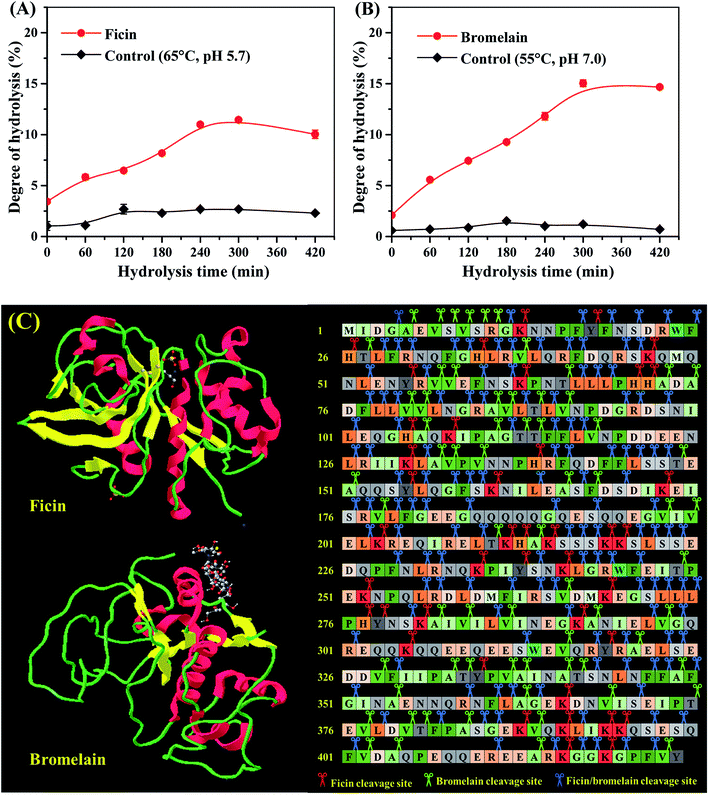 |
| Fig. 1 Hydrolysis curves of mung bean protein treated with ficin (A) and bromelain (B); (C) the predicted cleavage sites of ficin and bromelain on the major seed storage protein of mung bean (PDB: 2CV6). | |
3.2 Molecular mass distribution of hydrolysate
To further compare ficin and bromelain hydrolysis, SDS-PAGE was conducted to describe the molecular weight distribution of mung bean proteins as a function of hydrolysis time. Fig. 2 shows the molecular weight distribution of mung bean protein (control) with very intense broad bands in the range of 37 to 75 kDa, which probably corresponds to the 8S vicilin.24,25 32 and 25 kDa peptide might be 8S vicilin subunit, and the distinct bands of approximately 20 kDa most likely corresponded to 11S fractions or basic 7S subunit of seed storage protein.26 However, large molecular weight bands of mung bean proteins decreased gradually with the increasing hydrolysis time of ficin treatment. An obvious shift from higher molecular weight bands to lower molecular mass units was observed in all ficin hydrolysates, particularly hydrolysates prepared with ficin for 4 and 5 h. Similarly, the intensity of the higher molecular weight bands of bromelain hydrolysates decreased and even disappeared as a function of reaction time. Regardless of hydrolysis time, all bromelain-treated hydrolysates showed the explicit and uniform band around 15 kDa, which was different with that of ficin hydrolysates. Nevertheless, both ficin and bromelain caused the obvious downward shifts to increased percentages of the small peptides (<10 kDa), indicating the bioactive properties of mung bean protein hydrolysates.27
 |
| Fig. 2 Electrophoretic profiles of mung bean protein and hydrolysates obtained from ficin and bromelain. M: molecular weight markers (Precision Plus Protein Dual Color Standards, Biorad); control: mung bean protein without proteolysis; 60–300 min of enzymatic hydrolysis, respectively. | |
3.3 Structural characterization of mung bean protein hydrolysates
3.3.1 Ultraviolet-visible spectra analysis. Ultraviolet-visible spectroscopy assay was conducted to investigate the absorption spectra of hydrolysates obtained with ficin and bromelain. As shown in Fig. 3A, all ficin-treated hydrolysates had the similar ultraviolet-visible shapes in the range of 200–800 nm, whereas the absorption spectra of each hydrolysate in ultraviolet and visible area were different. For instance, ficin-treated hydrolysates fluctuated weakly under the visible light, but two noticeable absorption peaks (around 210 nm and 280 nm) were observed in the ultraviolet area. Interestingly, the maximum and minimum peak values of ficin hydrolysates were achieved after 0 min and 300 min hydrolysis, respectively. This might be attributed to the feature of ficin, resulting in the specific cleavage of peptide bonds. Unlikely, the hydrolysates treated with bromelain, regardless of hydrolysis time, presented almost overlapping ultraviolet-visible spectra (Fig. 3B). Both hydrolysates prepared with ficin and bromelain showed much higher ultraviolet absorption than that of control, implying that enzymatic hydrolysis led to the structural change of mung bean protein.
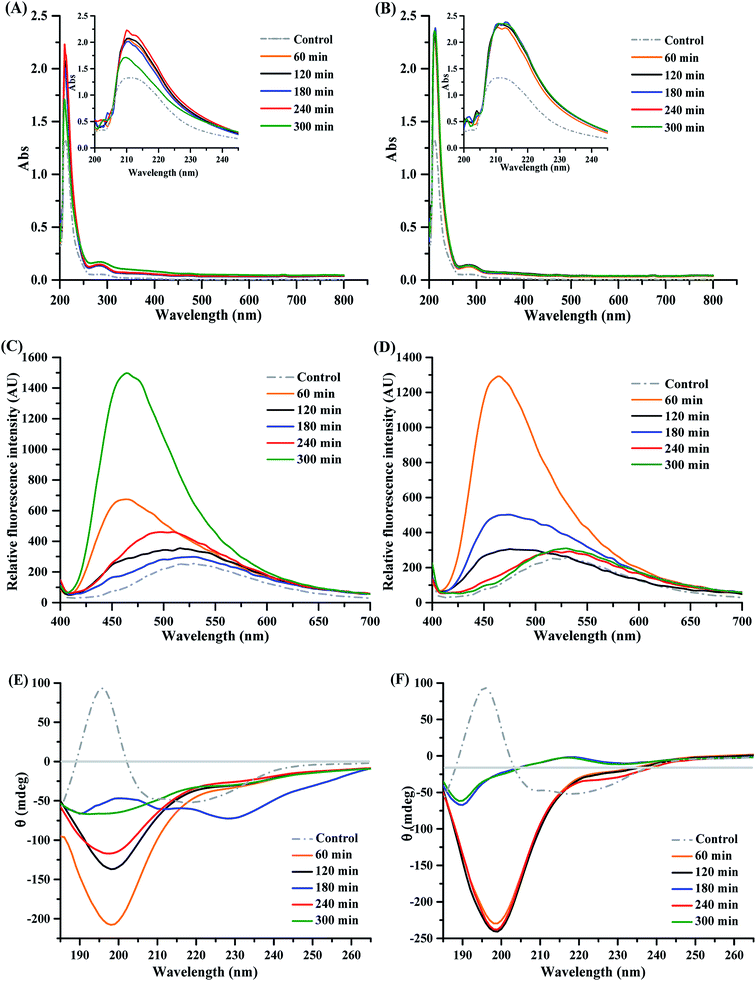 |
| Fig. 3 Structural characteristics of mung bean protein and hydrolysates using spectral analysis. UV-visible spectra of mung bean protein and hydrolysates prepared with ficin (A) and bromelain (B); relative fluorescence spectra of mung bean protein and hydrolysates prepared with ficin (C) and bromelain (D); circular dichroism (CD) spectra of mung bean protein and hydrolysates prepared with ficin (E) and bromelain (F). Control was mung bean protein without proteolysis. | |
3.3.2 Emission fluorescence spectroscopic analysis. The structural changes of mung bean protein as a function of ficin or bromelain hydrolysis were presented as the relative fluorescence intensity of ANS (a polarity-sensitive fluorescent probe) combined with the resulting protein hydrolysates (Fig. 3C and D). All hydrolysates had higher fluorescence intensity than that of control, reflecting the active affection of these two plant proteases on the hydrophobicity of mung bean protein. This result supported the point that hydrophobic amino acids (e.g. tyrosine, phenylalanine and tryptophan) in native protein are buried inside the protein cluster, while partial hydrolysis enables cleavage peptide bonds of protein molecules, and cause more exposure of hydrophobic groups and regions.8,28 However, different proteases and hydrolysis time resulted in various fluctuations of the fluorescence intensity. As shown in Fig. 3C, mung bean protein treated by ficin for 300 min led to the highest fluorescence emission spectra, following by the 60 min ficin-treated hydrolysates. Noticeably, ficin treatment resulted in the declination of the emission maximum (λmax) of the ANS-/hydrolysate complex, shifting from 530 to 465 nm. Interestingly, the hypsochromic shift in λmax also occurred in the hydrolysates prepared with bromelain (Fig. 3D). Nevertheless, the highest fluorescence spectrum was observed at the hydrolysate obtained with bromelain for 60 min, where the maximum fluorescence intensity was 2.6 folds over the second one (180 min-bromelain-treated hydrolysate). This may ascribe to the rapid depolymerization of proteins by bromelain during the first 1 h of hydrolysis, leading to the release of hydrophobic groups. Thereafter, as the hydrolysis time increased, these exposed hydrophobic groups was re-buried by their aggregation via hydrophobic interactions or increased protein flexibility, resulting in the declination of hydrophobicity in hydrolysates.8,29,30 The difference between ficin and bromelain hydrolysates might due to DH and enzyme specificity, which determined the cleavage of peptide bonds and the exposure of hydrophobic residues.
3.3.3 Circular dichroism (CD). As mentioned above, enzymatic hydrolysis could modify the tertiary structural conformation of mung bean protein. Based on this, we speculated that the change of secondary structure should occur. To confirm this, the structural modification of mung bean protein and hydrolysates at the secondary structure level was investigated by the CD spectroscopy (Fig. 3E and F). The CD spectrum of untreated mung bean protein (control) presented an obvious positive peak at 196 nm and two perceptible negative peaks around 209 and 220 nm, denoting a highly ordered structure, notably α type. However, ficin hydrolysis induced significant shift towards negative bands as compared to that of the control, indicating the disruption of ordered structures (Fig. 3E), which was also observed in soybean protein hydrolysates.31,32 More specifically, all ficin hydrolysates (excluding the one obtained at 180 min of hydrolysis) presented an obvious negative peak at 190–200 nm, implying the dominance of β-sheet and random coil conformations. Similar behavior was also found in the bromelain-treated hydrolysates, presenting as apparent negative bands in the tested wavelength ranges (Fig. 3F). The mung bean proteins treated with bromelain for 60, 120 and 240 min displayed similar CD spectra with sharp single and strong peak at 198 nm, which confirmed the high proportion of β-structures. One negative band at 190–195 nm and a small positive shoulder at around 220 nm were observed in the remaining hydrolysates, characterizing the absorption peak of irregular coiled conformation. These changes in CD spectra for both ficin and bromelain hydrolysates might due to the partial unfolding and reorganization of proteins during the formation procedure of hydrolysates.33
3.4 Surface charge (ζ-potential) and emulsifying properties
The frequently-used indicator for electrostatic interaction of protein hydrolysates is ζ-potential, which can be influenced by different factors such as particle composition.18,34 Thus, we evaluated the ζ-potential of mung bean protein treated with ficin or bromelain under different reaction time. As shown in Table 1, the ζ-potential values of mung bean protein and hydrolysates were all negative charges, demonstrating that particles of these samples stay apart to avoid molecular aggregation.18 Obviously, the negative charges on ficin hydrolysates were superior to that of untreated mung bean protein. Interestingly, similar trends toward ζ-potential of ficin- and bromelain-treated hydrolysates were also observed as the increasing of hydrolysis time. More specifically, the ζ-potential values of ficin hydrolysates decreased gradually over the hydrolysis time of 240 min, excluding 120 min, where the lowest value was observed. At the end of ficin hydrolysis, the resultant hydrolysate displayed the highest ζ-potential value of −9.35 ± 0.57 mV, whereas the lowest values were observed in the bromelain hydrolysates obtained at 240 and 300 min of hydrolysis. These differences might be correlated to the molecular mass distribution of ficin and bromelain hydrolysates (Fig. 2), which influenced the numbers of anionic and cationic groups they contain.35
Table 1 ζ-Potential (mV), emulsifying activity index (EAI, m2 g−1) and emulsion stability index (ESI, h) of ficin and bromelain hydrolysates obtained with different hydrolysis timea
Hydrolysis time (min) |
Ficin hydrolysate |
Bromelain hydrolysate |
ζ-Potential (mV) |
EAI (m2 g−1) |
ESI (h) |
ζ-Potential (mV) |
EAI (m2 g−1) |
ESI (h) |
Results having the same letter in each column are not significantly different (P > 0.05). |
60 |
−11.94 ± 1.00 c |
50.66 ± 0.81 a |
1.59 ± 0.04 c |
−11.88 ± 0.33 b |
23.34 ± 0.09 d |
2.28 ± 0.02 b |
120 |
−21.62 ± 0.57 f |
28.97 ± 2.78 c |
2.76 ± 0.63 b |
−13.30 ± 0.73 c |
22.99 ± 0.08 d |
0.93 ± 0.00 b |
180 |
−14.44 ± 0.41 d |
16.73 ± 0.00 d |
2.74 ± 0.00 b |
−11.06 ± 0.30 ab |
52.45 ± 0.08 b |
78.11 ± 18.51 a |
240 |
−19.62 ± 0.60 e |
15.62 ± 0.00 de |
2.62 ± 0.00 b |
−16.82 ± 0.32 d |
80.94 ± 0.08 a |
1.50 ± 0.01 b |
300 |
−9.35 ± 0.57 a |
13.37 ± 0.00 e |
6.48 ± 0.00 a |
−15.96 ± 1.88 d |
82.07 ± 0.14 a |
2.43 ± 0.02 b |
Control |
−10.50 ± 0.29 b |
43.60 ± 2.74 b |
1.43 ± 0.19 c |
−10.50 ± 0.29 a |
43.60 ± 2.74 c |
1.43 ± 0.19 b |
The emulsifying activity index (EAI) and emulsion stability index (ESI) for mung bean protein and hydrolysates as a function of increasing hydrolysis time are shown in Table 1. Hydrolysis time of ficin was found to exert a negative effect on its emulsifying activity, which declined from 50.66 ± 0.81 to 13.37 ± 0.00 m2 g−1 (P < 0.05). On the contrary, ESI values of ficin hydrolysates were superior to control group, presenting a tendency to grow over hydrolysis time. Similar results were reported by Thaiphanit et al.,36 where coconut protein hydrolysates in sunflower oil-in-water system showed the decreasing EAI and increasing ESI values as the increasing time. Unlikely, the EAI of bromelain hydrolysate was markedly increased by protein hydrolysis (P < 0.05), mainly in the second half of reaction time, possibly attributing to the molecular flexibility of polypeptides and the exposure of hydrophobic areas.29 However, no obvious fluctuation of ESI was observed in emulsion stabilized by bromelain hydrolysates along with the progress of hydrolysis, excluding 180 min hydrolysis time. The highest ESI value of 78.11 h was found in the 180 min-bromelain-treated hydrolysate, which might be due to its high hydrophobicity and secondary structure. Intriguingly, all hydrolysates exhibited the ESI values more than 55 min, which were higher than those of fish protein hydrolysates,37 porcine plasma protein hydrolysates38 and wheat gluten hydrolysates.39 Overall, the emulsifying properties of bromelain hydrolysates were superior to ficin hydrolysates.
3.5 DPPH radical scavenging activity
Apart from the physicochemical characteristics of mung bean protein hydrolysates, we also measured their capacity on scavenging DPPH radical. As the concentrations increased, DPPH radical scavenging activity of each hydrolysate increased gradually until a steady state was achieved (Fig. 4A and B). Mung bean hydrolysates obtained from ficin showed strong DPPH radical scavenging activity with the IC50 values ranging from 9.45 to 16.22 μg mL−1, and the lowest and highest values were respectively observed at 120 min and 60 min of hydrolysis (Fig. 4A). In terms of bromelain hydrolysates, excellent ability for capturing the DPPH radical was found at 300 min of hydrolysis, with IC50 value of 8.67 μg mL−1 (Fig. 4B), which were lower than those of documented protein hydrolysates, such as black bean hydrolysate (IC50 = 21.23 μg mL−1)16 and gelatin protein hydrolysate (IC50 = 660 μg mL−1).40 Our results indicated that enzyme type and hydrolysis time greatly influence the DPPH radical scavenging activity of mung bean protein hydrolysates, going along with the previous reports that antioxidant activity of protein hydrolysates relied on multiple factors, especially protein substrate, the specificity of proteases and hydrolysis conditions employed.22,41
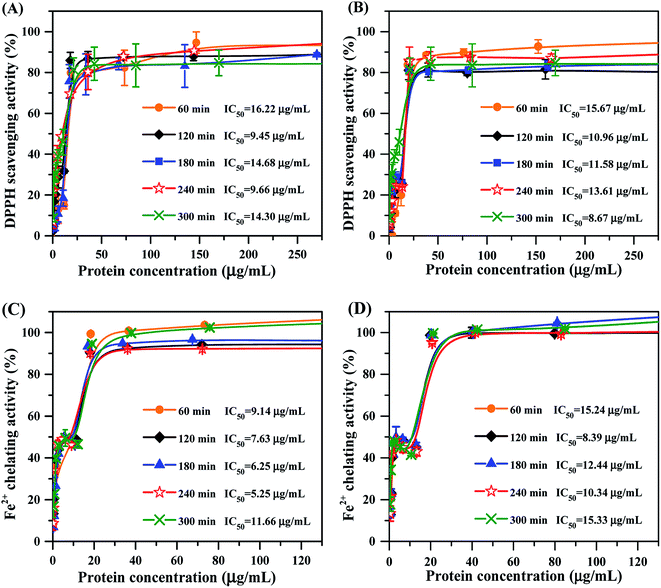 |
| Fig. 4 DPPH radical scavenging activity and Fe2+-chelating activity of mung bean protein hydrolysates. (A) DPPH radical scavenging activity of ficin hydrolysates; (B) DPPH radical scavenging activity of bromelain hydrolysates; (C) Fe2+-chelating activity of ficin hydrolysates; (D) Fe2+-chelating activity of bromelain hydrolysates. | |
3.6 Metal ion-chelating activity
Ferrous ion (Fe2+) is well-known to catalyze generation of free radicals, leading to oxidative damage for biomacromolecules.42 So, we evaluated the ability of mung bean protein hydrolysates on chelating Fe2+. As depicted in Fig. 4C and D, Fe2+-chelating activity of all hydrolysates were enhanced with increase in the protein concentration up to approximately 40 μg mL−1, but further increases in concentration affected their activity slightly. Mung bean protein hydrolysates obtained from ficin hydrolysis presented prominent capacity to chelate metal ion with IC50 values ranging from 5.25 to 11.66 μg mL−1 (Fig. 4C), which were significantly lower than these of previous reports.43,44 The IC50 values of bromelain hydrolysate were between 8.39 and 15.33 μg mL−1 over the range of hydrolysis time studied. Obviously, hydrolysates prepared with bromelain showed much weaker Fe2+-chelating activity than the hydrolysates obtained from ficin hydrolysis at the same reaction time. This result was coincided with the molecular weight distribution of bromelain-treated hydrolysates, which presented higher molecular weight bands. Accordingly, we reason that ficin hydrolysis was favorable to the release of acidic and alkaline amino acids, thereby promoted the resultant hydrolysates to chelate Fe2+ and thus to retard the oxidation reaction.45
3.7 Inhibition of lipid oxidation
3.7.1 Antioxidant activity in linoleic acid. Ficin- and bromelain-treated hydrolysates respectively obtained at 120 min and 300 min, with the highest DPPH radical scavenging activity, were selected to investigate the antioxidant activity in retarding lipid oxidation. Thus, ferric thiocyanate method was adopted to detect the peroxides levels during the initial stage of linoleic acid oxidation, which are the primary products of oxidation.46 As shown in Fig. 5A, a sharp rise in the OD values of control group was observed at the first two days, indicating a rapid increase of peroxides production in the autoxidation of linoleic acid. Later, control group presented a decrease in the absorbance possibly because the peroxide decomposition had begun.23 When added the selected ficin hydrolysate to linoleic acid, OD values were kept around 0.08 and peroxide inhibition reached up to 94.35 ± 0.26%, demonstrating that ficin hydrolysate had the potential to suppress the linoleic acid peroxidation formation. From day 3 on, however, an increase in OD values occurred. This phenomenon showed that the selected ficin hydrolysate could retard the autoxidation of linoleic acid over the range of investigated time but not totally inhibit. Intriguingly, linoleic acid with addition of selected bromelain hydrolysate had the lowest absorbances of approximately 0.08 and the highest inhibition effects up to 94.55 ± 0.10%, through the experimental period. This result showed that this bromelain hydrolysate could effectively inhibit the formation of peroxides, and thus increase the oxidative stability of linoleic acid. In a word, linoleic acid oxidation was remarkably retarded by both mung bean protein hydrolysates, and thereinto bromelain hydrolysate performed better.
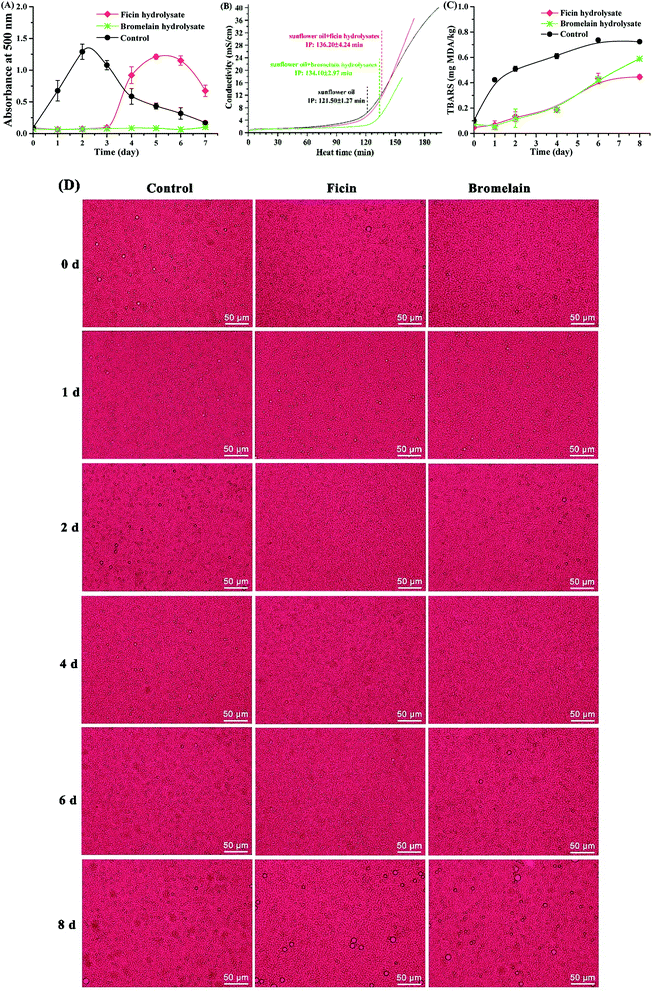 |
| Fig. 5 Antioxidant effects of mung bean protein hydrolysates in linoleic acid (A), sunflower oil (B), oil-in-water emulsion (C) and emulsion micrographs (D). Control: without the addition of protein hydrolysates; ficin hydrolysate: mung bean protein treated with ficin for 120 min; bromelain hydrolysate: mung bean protein treated with bromelain for 300 min. | |
3.7.2 Antioxidant activity in sunflower oil. Since these two mung bean protein hydrolysates were capable to retard linoleic acid oxidation, we reasoned that the samples might be active on the oxidative stability of edible oils enriched in linoleic acid. Thus, sunflower oil high in linoleic acid was used to investigate the antioxidant activity of these two hydrolysates by means of rancimat stability assay. As described in Fig. 5B, the conductivity of sunflower oil with or without hydrolysates showed a time-dependent manner. A rapid increase of conductivity in sunflower oil was observed after 121 min, reflecting the start of lipid oxidation. The presence of protein hydrolysates obtained from ficin and bromelain hydrolysis elevated the induction period to 136.20 ± 4.24 min and 134.10 ± 2.97 min, respectively, which significantly enhanced the oxidative stability of sunflower oil (P < 0.05). Notably, the efficiencies of these two hydrolysates were comparable to that of coriander extract (1600 mg kg−1)47 and tea polyphenol palmitate (100 mg kg−1).48 As for the antioxidant effects between ficin and bromelain hydrolysates, there existed no appreciable difference on induction period.
3.7.3 Antioxidant activity in O/W emulsion. To further confirm the antioxidant activity of protein hydrolysates, TBARS test was used to measure the formation of secondary products in sunflower oil-in-water emulsion at the presence of hydrolysates. As the storage progressed, the MDA production in O/W emulsions increased gradually (Fig. 5C), corresponding to previous reports on the oxidative stability of O/W emulsion with rice bran protein hydrolysate49 or potato protein hydrolysate.50 Compared with control emulsion, the presence of these two mung bean protein hydrolysates could prominently reduce the MDA production. More specifically, TBARS levels in O/W emulsions with the addition of ficin- and bromelain-hydrolysates were markedly decreased by 6.4 and 8.1 folds, respectively, at day 2. Both hydrolysates showed similar inhibitory behavior on the MDA production during 6 days-storage. At the end of the storage period, i.e. 8 d, ficin-treated hydrolysate presented the lowest TBARS value in comparison with other groups. This was in accordance with the microstructural observation of the emulsions (Fig. 5D). All the emulsions with or without protein hydrolysates had uniform and small droplets during the storage period of 6 days, whereas emulsions yielded some large droplets at the last day studied. This phenomenon was in accordance with the point that small droplet size could be beneficial for enhancing the oxidative stability of emulsion.51,52Taken together, mung bean protein treated with ficin and bromelain could inhibit the formation of primary and secondary products during lipid oxidation. As Fig. 6 described, peptides derived from mung bean protein hydrolysates could effectively scavenge radicals by means of donating hydrogen to lipid radicals, further retarding the radical-mediated oxidative chain reactions so as to enhance the oxidative stability of lipid products.53 Also, the hydrolysates showed excellent capacity on chelating metal ions, which could catalyze the generation of free radicals.54 Therefore, lipid oxidation was suppressed by mung bean hydrolysates, particularly the bromelain hydrolysate.
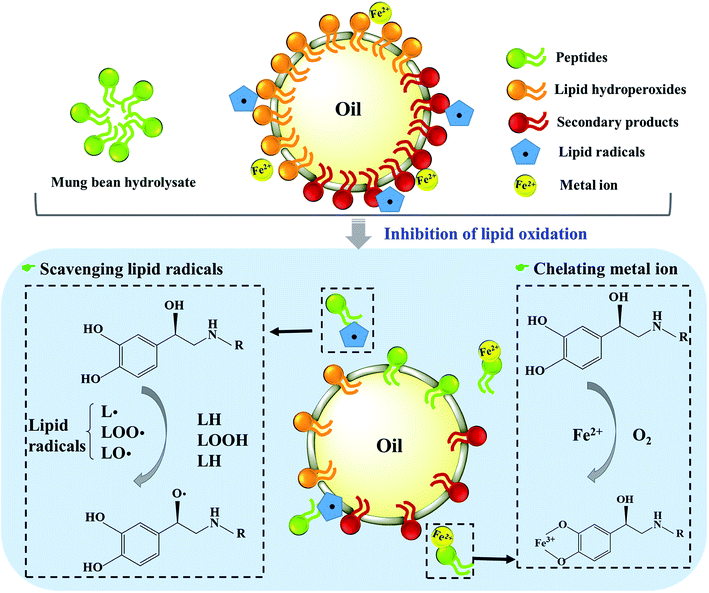 |
| Fig. 6 Schematic representation of mung bean protein hydrolysates on retarding lipid oxidation. | |
4. Conclusions
In summary, we compared the physicochemical and antioxidative properties of mung bean protein hydrolysates obtained by proteolytic treatments with two plant proteases (ficin and bromelain). Mung bean proteins treated with ficin and bromelain under different hydrolysis time showed different physicochemical properties, but bromelain hydrolysate was superior in DH and emulsifying properties. All protein hydrolysates prepared with ficin and bromelain exhibited excellent DPPH radical scavenging activity with the lowest IC50 values of 9.66 and 8.67 μg mL−1, respectively. Also, Fe2+-chelating activity of each hydrolysate prepared with ficin was stronger than that treated by bromelain at the identical hydrolysis time. Ficin- and bromelain-treated hydrolysates with the highest DPPH radical activity were further evidenced to enhance the oxidative stability of linoleic acid, sunflower oil and O/W emulsion, and the latter appeared to be more effective. Therefore, mung bean protein hydrolysates treated with ficin and bromelain favored oil preservation, since these hydrolysates could potentially scavenge DPPH radicals, chelate metal ions and inhibit lipid oxidation.
Conflicts of interest
There are no conflicts to declare.
Acknowledgements
This research was financially supported by Natural Science Foundation of China (31901730, 31671786), Natural Science Foundation of Jiangsu Province (BK20190587), China Postdoctoral Science Foundation (2018M632235) and National Key R&D Program of China (2016YFD0401404).
References
- D. Tilman and M. Clark, Nature, 2014, 515, 518 CrossRef CAS PubMed.
- M. D. Kristensen, N. T. Bendsen, S. M. Christensen, A. Astrup and A. Raben, Food Nutr. Res., 2016, 60, 32634 CrossRef PubMed.
- E. H. Temme, I. B. Toxopeus, G. F. Kramer, M. C. Brosens, J. M. Drijvers, M. Tyszler and M. C. Ocke, Public Health Nutr., 2015, 18, 2433–2445 CrossRef PubMed.
- T. G. Kudre, S. Benjakul and H. Kishimura, J. Sci. Food Agric., 2013, 93, 2429–2436 CrossRef CAS PubMed.
- Food and Agriculture Organization of the United Nations, FAO Food Nutr. Pap., 2013, 92, 1–66 Search PubMed.
- A. Connolly, M. B. O'Keeffe, C. O. Piggott, A. B. Nongonierma and R. J. FitzGerald, Food Chem., 2015, 176, 64–71 CrossRef CAS PubMed.
- T. L. Pownall, C. C. Udenigwe and R. E. Aluko, J. Agric. Food Chem., 2010, 58, 4712–4718 CrossRef CAS PubMed.
- J. A. Evangelho, N. L. Vanier, V. Z. Pinto, J. J. Berrios, A. R. Dias and E. R. Zavareze, Food Chem., 2017, 214, 460–467 CrossRef CAS PubMed.
- J. Torruco-Uco, L. Chel-Guerrero, A. Martínez-Ayala, G. Dávila-Ortíz and D. Betancur-Ancona, LWT--Food Sci. Technol., 2009, 42, 1597–1604 CrossRef CAS.
- R. Pachecoaguilar, M. A. Mazorramanzano and J. C. Ramírezsuárez, Food Chem., 2008, 109, 782–789 CrossRef CAS PubMed.
- S. He, C. Franco and W. Zhang, Food Res. Int., 2013, 50, 289–297 CrossRef CAS.
- K. Elavarasan, V. N. Kumar and B. A. Shamasundar, J. Food Process. Preserv., 2014, 38, 1207–1214 CrossRef CAS.
- D. Y. Cho, K. Jo, S. Y. Cho, J. M. Kim, K. Lim, H. J. Suh and S. Oh, Korean Journal for Food Science of Animal Resources, 2014, 34, 362–371 CrossRef PubMed.
- C. S. F. Bah, A. Carne, M. A. McConnell, S. Mros and A. E. D. A. Bekhit, Food Chem., 2016, 202, 458–466 CrossRef CAS PubMed.
- M. Du, J. Xie, B. Gong, X. Xu, W. Tang, X. Li, C. Li and M. Xie, Food Hydrocolloids, 2018, 76, 131–140 CrossRef CAS.
- Z. Zheng, J. Li, J. Li, H. Sun and Y. Liu, Food Hydrocolloids, 2019, 97, 105222 CrossRef.
- P. M. Nielsen, D. Petersen and C. Dambmann, J. Food Sci., 2001, 66, 642–646 CrossRef CAS.
- S.-S. Teh, A. E.-D. A. Bekhit, A. Carne and J. Birch, Food Chem., 2016, 203, 199–206 CrossRef CAS PubMed.
- I. Suppavorasatit, E. G. De Mejia and K. R. Cadwallader, J. Agric. Food Chem., 2011, 59, 11621–11628 CrossRef CAS PubMed.
- C. Li, P. Sun, H. Yu, N. Zhang and J. Wang, RSC Adv., 2017, 7, 1869–1876 RSC.
- A. Mohan, M. C. Udechukwu, S. R. C. K. Rajendran and C. C. Udenigwe, RSC Adv., 2015, 5, 97400–97407 RSC.
- A. Bougatef, N. Nedjar-Arroume, L. Manni, R. Ravallec, A. Barkia, D. Guillochon and M. Nasri, Food Chem., 2010, 118, 559–565 CrossRef CAS.
- M. E. Caetano-Silva, L. R. B. Mariutti, N. Bragagnolo, M. T. B. Pacheco and F. M. Netto, J. Agric. Food Chem., 2018, 66, 1981–1989 CrossRef CAS PubMed.
- C. H. Tang and X. Sun, J. Agric. Food Chem., 2010, 58, 6395–6402 CrossRef CAS PubMed.
- Z. Yi-Shen, S. Shuai and R. FitzGerald, Food Nutr. Res., 2018, 62 DOI:10.29219/fnr.v62.1290.
- E. M. Mendoza, M. Adachi, A. E. Bernardo and S. Utsumi, J. Agric. Food Chem., 2001, 49, 1552–1558 CrossRef CAS PubMed.
- Z. J. Zheng, Y. Huang, R. J. Wu, L. M. Zhao, C. F. Wang and R. J. Zhang, Poult. Sci., 2014, 93, 2641–2650 CrossRef CAS PubMed.
- R. He, A. Alashi, S. A. Malomo, A. T. Girgih, D. Chao, X. Ju and R. E. Aluko, Food Chem., 2013, 141, 153–159 CrossRef CAS PubMed.
- S. B. Zhang and Q. Y. Lu, Food Hydrocolloids, 2015, 47, 51–60 CrossRef CAS.
- N. A. Avramenko, N. H. Low and M. T. Nickerson, Food Res. Int., 2013, 51, 162–169 CrossRef CAS.
- J. Zhao, Y. L. Xiong and D. H. McNear, J. Food Sci., 2013, 78, C152–C159 CrossRef CAS PubMed.
- A. Achouri and Z. Wang, Food Res. Int., 2001, 34, 507–514 CrossRef CAS.
- M. Mohammadian and A. Madadlou, Food Hydrocolloids, 2016, 52, 221–230 CrossRef CAS.
- E. da Rosa Zavareze, A. C. Telles, S. L. Mello El Halal, M. da Rocha, R. Colussi, L. Marques de Assis, L. A. Suita de Castro, A. R. Guerra Dias and C. Prentice-Hernández, LWT--Food Sci. Technol., 2014, 59, 841–848 CrossRef CAS.
- X. Xu, J. Zhong, J. Chen, C. Liu and D. J. Mcclements, Food Chem., 2016, 213, 700–707 CrossRef CAS PubMed.
- S. Thaiphanit, G. Schleining and P. Anprung, Food Hydrocolloids, 2016, 60, 252–264 CrossRef CAS.
- Y. Liu, X. Li, Z. Chen, J. Yu, F. Wang and J. Wang, Food Chem., 2014, 151, 459–465 CrossRef CAS PubMed.
- Q. Liu, B. H. Kong, Y. L. L. Xiong and X. F. Xia, Food Chem., 2010, 118, 403–410 CrossRef CAS.
- W. He, R. Yang and W. Zhao, Food Chem., 2019, 277, 655–663 CrossRef CAS PubMed.
- D.-H. Ngo, Z.-J. Qian, B. Ryu, J. W. Park and S.-K. Kim, J. Funct. Foods, 2010, 2, 107–117 CrossRef CAS.
- V. Klompong, S. Benjakul, D. Kantachote and F. Shahidi, Food Chem., 2007, 102, 1317–1327 CrossRef CAS.
- Y. Shi, J. Kovacs-Nolan, B. Jiang, R. Tsao and Y. Mine, J. Funct. Foods, 2014, 10, 35–45 CrossRef CAS.
- R. He, A. T. Girgih, S. A. Malomo, X. Ju and R. E. Aluko, J. Funct. Foods, 2013, 5, 219–227 CrossRef CAS.
- Q. Zhang, X. Tong, B. Qi, Z. Wang, Y. Li, X. Sui and L. Jiang, J. Funct. Foods, 2018, 42, 298–305 CrossRef CAS.
- S. Dong, M. Zeng, D. Wang, Z. Liu, Y. Zhao and H. Yang, Food Chem., 2008, 107, 1485–1493 CrossRef CAS.
- H. T. Balaydın, İ. Gülçin, A. Menzek, S. Göksu and E. Şahin, J. Enzyme Inhib. Med. Chem., 2010, 25, 685–695 CrossRef PubMed.
- P. M. Angelo and N. Jorge, J. Am. Oil Chem. Soc., 2008, 85, 1045–1049 CrossRef CAS.
- K. Wang, Z. Zheng, C. Liu, Y. Wang, J. Li and Y. Liu, LWT--Food Sci. Technol., 2020, 118, 108726 CrossRef.
- N. Cheetangdee and S. Benjakul, J. Sci. Food Agric., 2015, 95, 1461–1468 CrossRef CAS PubMed.
- Y. Cheng, Y. L. Xiong and J. Chen, Food Chem., 2010, 120, 101–108 CrossRef CAS.
- W. Dridi, W. Essafi, M. Gargouri, F. Leal-Calderon and M. Cansell, Food Chem., 2016, 202, 205–211 CrossRef CAS PubMed.
- K. Nakaya, H. Ushio, S. Matsukawa, M. Shimizu and T. Ohshima, Lipids, 2005, 40, 501–507 CrossRef CAS PubMed.
- S. Maqsood and S. Benjakul, Food Chem., 2010, 119, 123–132 CrossRef CAS.
- C. L. Salcedo and M. A. Nazareno, RSC Adv., 2015, 5, 45878–45887 RSC.
|
This journal is © The Royal Society of Chemistry 2020 |
Click here to see how this site uses Cookies. View our privacy policy here.