DOI:
10.1039/C9RA08723K
(Paper)
RSC Adv., 2020,
10, 9063-9069
MOF-derived ZnCo2O4 porous micro-rice with enhanced electro-catalytic activity for the oxygen evolution reaction and glucose oxidation†
Received
24th October 2019
, Accepted 13th December 2019
First published on 2nd March 2020
Abstract
A porous ZnCo2O4 micro-rice like microstructure was synthesized via calcination of a Zn–Co MOF precursor at an appropriate temperature. The as-prepared ZnCo2O4 sample presented good electrocatalytic oxygen evolution reaction performance with a small overpotential (η10 = 389 mV) and high stability in basic electrolyte. Furthermore, in basic medium, the as-synthesized ZnCo2O4 micro-rice also showed good electrocatalytic activity for glucose oxidation. A ZnCo2O4 micro-rice modified glass carbon electrode may be used as a potential non-enzymatic glucose sensor. The excellent electrocatalytic OER and glucose oxidation performances of ZnCo2O4 might be attributed to the unique porous structure formed by the nanoparticles. The porous architecture of the micro-rice can provide a large number of electrocatalytically active sites and high electrochemical surface area (ECSA). The result may offer a new way to prepare low-cost and high performance oxygen evolution reaction and glucose oxidation electrocatalysts.
1 Introduction
Metal–organic frameworks (MOFs), also named metal–organic coordination polymers (MOCPs), are a new class of porous materials and have attracted great attention due to their potential applications in the gas storage and separation, catalysis, luminescence, sensing, and energy storage fields.1–4 In recent years, the micro-nano structured MOFs with special morphologies have been prepared from metal ions and organic ligands in different solvent systems.5–11 Utilizing MOFs as hard templates or precursors can provide an effective strategy to synthesize novel micro-nano structured materials including metal oxides, metal chalcogenides, metal phosphides, metal carbides, porous carbon materials, and other complex micro-nanostructures.5–14 The MOF-derived micro-nano materials can exhibit several structure-dependent merits in the electrocatalytic oxygen evolution reaction (OER) and glucose oxidation fields.
In recent years, spinel AIIBIII2O4 micro-nano structures have aroused great attention because of their potential application in batteries, supercapacitors, catalytic, and sensing fields.15 So far, a great of spinel Co3O4 (CoIICoIII2O4) structures with different sizes and morphologies have been synthesized as electrode materials for electrochemical oxygen evolution reaction.16,17 Unfortunately, the Co3O4-based electrodes own poor electrical conductivity and high-cost, thus hampered to extensively practical applications. Therefore, using low-cost metal Zn(II) ions to partially substitute Co(II) ions of Co3O4 to get ZnCo2O4 could improve the electrocatalytic properties.18–20 Ternary ZnCo2O4 materials reveal higher electrical conductivity and enhanced electrochemical activity ascribed to the co-existence of the Zn and Co species. Therefore, zinc cobalt oxides (ZnCo2O4) with unique porous structure are anticipated with good performance for electrochemical energy storage and conversion.
Recently, a great of attentions have been paid to the development of electrocatalysts for OER, and OER plays a critical role in water splitting, Zn–air batteries, and regenerative fuel cells fields.21–27 To date, different Co-based micro-nano structured catalysts, including Co3O4,16,17 Co3S4,28 CoSe2,29 CoMoO4,30 NixCo3−x(PO4)2,31 have been developed as OER catalysts and showed good OER performances. On other hand, in recent ten years, electrochemical non-enzymatic glucose sensors based on metal oxides have caused widespread interest due to their easy preparation, cheap cost, and portable characteristics in the detection of glucose.32–34 Up to now, many metal oxides micro/nano-structures such as NiO, CuO, Co3O4, CuCo2O4, and NiCo2O4, have been extensively used for glucose sensing.35–42 Porous hierarchical micro/nano-structures have attracted great interest in electrocatalytic OER and glucose sensing attribute to the unique structure with rich active sites, large electrolyte contact area, and quick electron/active species transfer rate. Based on the above considerations, we exhibit the rational design of ZnCo2O4 hierarchical porous micro-rice like structure based on thermolysis of a Zn–Co MOF precursor, and the as-prepared material shows enhanced electrocatalytic activity for OER and glucose oxidation.
2 Experimental
2.1 Synthesis of ZnCo2O4 porous micro-rice like structure
In a typical synthesis procedure, zinc nitrate hexahydrate Zn(NO3)2·6H2O (0.010 g, 0.03 mmol), cobalt nitrate hexahydrate Co(NO3)2·6H2O (0.0189 g, 0.06 mmol), p-phthalic acid (0.012 g, 0.07 mmol), and polyvinylpyrrolidone (PVP, K30, 0.92 g) were added to the mixture of 5.0 mL N-N-dimethylformamide (DMF) and 3.0 mL ethanol, the above mixed solution was stirred vigorously for 30 min, sealed and heated to 160 °C for 3 h. After cooling to room temperature, the obtained powder was isolated by centrifugation and washed by ethanol three times, and then dried at 60 °C for 2 h. The porous ZnCo2O4 micro-rice like structure was obtained by annealing the as-synthesized MOF precursor at 400 °C for 2 h in air (2 °C min−1).
2.2 Reagents and materials characterization
The chemicals include Zn(NO3)2·6H2O, Co(NO3)2·6H2O, glucose (GLU), dopamine (DA), uric acid (UA), ascorbic acid (AA), and polyvinyl pyrrolidone (PVP) were purchased from Beijing Chemical Reagent Co., China. p-Phthalic acid was purchased from Aladin Ltd. (Shanghai, China). All of these chemical reagents are of analytical grade and used as received without further purification.
The phase of ZnCo2O4 was characterized by X-ray diffraction (XRD) on a Rigaku-Ultima III diffractometer with CuKα radiation. Field scanning electron microscopy (FSEM, Hitachi SU8010) and transmission electron microscopy (TEM, FEI Tecnai G2 s-twin D573) equipments were used to characterize the morphology and microstructure for the as-synthesized samples. The data of specific surface areas was collected by N2 adsorption on Gemini VII 2390 Analyzer at 77 K. The chemical composition and valence of the obtained materials were analyzed by X-ray photoelectron spectroscopy on XPS Thermo 250Xi. Thermogravimetric analysis (TGA) was carried out in the nitrogen atmosphere on a Netzsch STA-449F3 thermogravimetric analyzer at a heating rate of 10 °C min−1.
2.3 Preparation and measurement of OER electrocatalytic electrode
The electrochemical test was conducted on an electrochemical workstation (CHI 760E) equipped with high-speed rotators from Pine instrument, using O2 pre-saturated 1.0 M KOH solution as the electrolyte (pH = 14.0). For the electrochemical measurement, a three-electrode setup was employed in which a glassy carbon electrode (GCE) with a diameter of 5 mm (0.196 cm2), a platinum wire and a Ag/AgCl electrode were employed as the working electrode, counter electrode and reference electrode, respectively. The catalyst ink was prepared as follow, an amount of 5 mg ZnCo2O4 sample was added to a mixture of H2O/isopropanol (v/v = 3
:
1, 1 mL) with 50 μL Nafion solution, and the above solution was strongly ultrasonic to acquire a homogenous ink dispersion. Then, 10 μL of the catalyst ink was casted onto the polished GCE and dried at room temperature. The linear sweep voltammetry (LSV) test was carried out with a scan rate of 1 mV s−1 at 1600 rpm. Electrochemical impedance spectroscopy (EIS) was recorded at open circuit potential in the frequency scan range from 100 kHz to 0.01 Hz.
2.4 Preparation and measurement of electrochemical sensor electrode
The bare GC electrode was carefully polished with different sizes of Al2O3 slurries (1.0, 0.3, and 0.05 μm) prior to modification. The as-obtained ZnCo2O4 catalyst (1.0 mg) was dispersed to 1.0 mL ultrapure water (18.2 MΩ cm), giving a dispersion with strongly ultrasonic technique. After that, a 5.0 μL of the micro-rice catalysts suspension was dropped on the cleaning GC electrode with a diameter of 3 mm and dried at room temperature before to use.
3 Results and discussion
The scanning electron microscopy (SEM) images of the as-prepared Zn–Co-based MOF precursors and porous ZnCo2O4 micro-rice like structure was shown in Fig. 1. The as-prepared MOF precursor displays micro-rice structure roughly 1.71 μm in length and 0.85 μm in width, which assembled by nanosheets (Fig. 1a, b, S1a and b†).
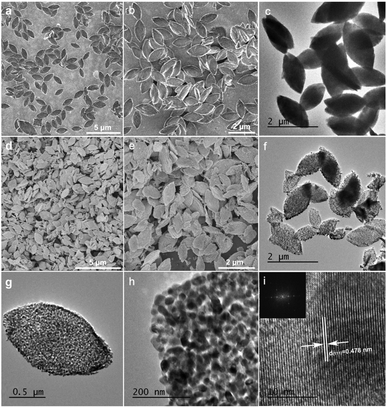 |
| Fig. 1 (a and b) The SEM images of the 3D structure of Zn–Co-based MOF precursors, (c) TEM image of Zn–Co-based MOF precursors, (d and e) the SEM images of the 3D structure of ZnCo2O4 porous micro-rice like structure obtained after calcination of Zn–Co-based MOF precursors, (f) the TEM image of ZnCo2O4 porous micro-rice like structure, (g) the TEM image of a single ZnCo2O4 micro-rice, (h) magnified TEM image of (g), (i) the HRTEM image and FFT of the ZnCo2O4 porous micro-nano structure (inset). | |
The formation process of the Zn–Co-based MOF precursors was tuned by a series of control experiment. When 0.09 mmol of Zn(NO3)2·6H2O was used as raw material with the absence of Co(NO3)2·6H2O, and kept other reaction parameters unchanged, only microspheres can be obtained. The large size of irregular rectangle-like nanosheets can be obtained when the usage of Co(NO3)2·6H2O increased to 0.27 mmol with the Zn/Co molar ratio of 1
:
9 (Fig. S2†). Although most of the micro-rice morphology is remained as decreasing the amount of p-phthalic acid to half, however, the dispersibility of nanosheets becomes inferior (Fig. S3†). When tuned the solvent volume ratio of DMF and ethanol from 5
:
3 to 4
:
4, the yield of micro-rice morphology of nanosheets became fewer (Fig. S4†). As shown in Fig. S5,† other things being equal, spindle-like microparticles can be synthesized when DMF was substituted by isometric N,N-dimethylacetamide (DMA). The TEM image also reveals that a nonporous feature of the precursors and the micro-rice morphology composing of closely stacked lamella with different contrast of the observed layers as shown in Fig. 1c, S6a and b.† Thermogravimetric analysis (TGA) was carried out to evaluate the thermal stability of the Zn–Co MOF sample between 40 and 800 °C. TG curve of MOF shows sharp weight loss of 46.40% from 328 to 399 °C corresponds to the decomposition of the p-phthalic acid ligand (Fig. S7†). Thus, we selected 400 °C as the calcination temperature. After annealing at 400 °C for 2 h in atmosphere condition, the MOF precursors can be easily converted to ZnCo2O4, which maintains the initial micro-rice precursor morphology well with a slight dimension shrinkage (Fig. 1d, e, S8a and b†). The high magnified SEM image in Fig. 1e further demonstrated that the distinctly hierarchical structure of ZnCo2O4, as shown in the lateral section, which is easy to yield by sintering. The TEM images of ZnCo2O4 were presented in Fig. 1f and h, clearly certifying porous architecture can be derived from pyrolysis of Zn–Co-based MOF. The porous and hierarchical structure of ZnCo2O4 with accessible surface facilitated to the fast ions and molecules transfer, suggesting potential good properties. The high resolution TEM image in Fig. 1i indicates the micro-rice presence clear lattice finger with a lattice interplanar space of 0.478 nm, which is corresponded to the (111) crystal plane of spinel ZnCo2O4. The FFT inset in Fig. 1i also revealed the high crystallinity of the annealed sample.
The XRD pattern of the as-prepared ZnCo2O4 is shown in Fig. 2a. All the peaks present in the pattern can well match with the standard cards of ZnCo2O4 (JCPDS 23-1390). Energy-dispersive X-ray (EDX) analysis reveals the coexistence of Zn, Co, O elements in the ZnCo2O4 micro-rice (Fig. 2b), and the atomic ratio of Zn and Co is about 1
:
2.15. The Brunauer–Emmett–Teller (BET) specific surface area of ZnCo2O4 obtained from N2 sorption isotherms is 25 m2 g−1. The as-synthesized sample shows a typical mesoporous structure with an average pore diameter of ∼3.6 nm (inset in Fig. 2c). This unique mesoporous structure is beneficial for molecules/ions diffusion and adsorption. The surface chemical state of ZnCo2O4 micro-rice was also investigated by XPS, the survey spectrum in Fig. S5† illustrated the existance of Zn, Co, and O elements. The high-resolution XPS spectrum of Zn 2p in Fig. 2d exhibited two peaks at binding energy of 1020.16 and 1043.40 eV, which is corresponded to Zn 2p3/2 and Zn 2p1/2 of Zn2+ ions. Meanwhile, the doublet peaks located at 780.16 and 795.42 eV was assigned to Co 2p3/2 and Co 2p1/2 of Co2+ ions, and another pair of peaks at 778.95 and 794.11 eV was ascribed to Co3+ ions (Fig. 2e), which is benefitting for the electrocatalytic process of OER. The O 1s peaks can be deconvoluted into three separated peaks, the peaks of BEs at 529.02 and 531.71 eV matched well with metal–oxygen bonds and physisorption of oxygen in hydroxyl groups, respectively, while, the peak at 530.18 eV suggested the present of oxygen vacancy (Fig. 2f), which could be attributed to the high conductivity of ZnCo2O4 electrocatalyst.
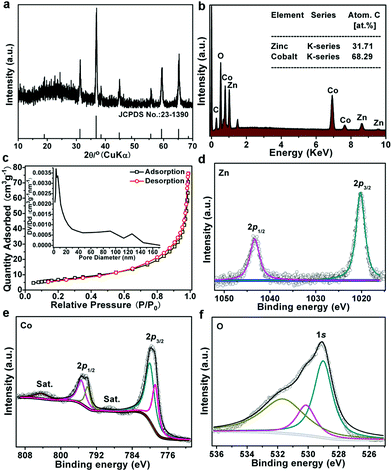 |
| Fig. 2 (a) Powder X-ray diffraction patterns of the simulated and as-synthesized ZnCo2O4, (b) EDX of the ZnCo2O4 micro-rice structure, (c) N2 adsorption/desorption isothermal curve and pore size distribution of the ZnCo2O4 micro-nano structure, high-resolution XPS spectrum of (d) Zn 2p, (e) Co 2p, (f) O 1s. | |
3.1 OER activity and stability
The OER test of ZnCo2O4 micro-rice like structure was recorded in an O2-saturated alkaline solution (1.0 M KOH) with a three-electrode setup. Fig. 3a showed the linear sweep voltammogram (LSV) of catalyst with a scan rate of 1 mV s−1, the overpotential of ZnCo2O4 micro-rice like structure is 389 mV at current density of 10 mA cm−2, which can compare to some ZnCo2O4 and Co3O4 based catalysts, such as ZnCo2O4 micro-spindle,18 Zn0.3Co2.7O4 porous willow-leaf like structure,19 mesoporous Co3O4,43 and CuCo2O4 sample.44 The Tafel plot of ZnCo2O4 catalyst was investigated to evaluate the OER performance and as shown in Fig. 3b. The ZnCo2O4 micro-rice like structure exhibits small Tafel slope of 61.84 mV dec−1. The small Tafel slope of ZnCo2O4 sample implied a fast OER reaction kinetics and practical applications. Furthermore, stability of the sample was evaluated by chronoamperometry test, as shown in Fig. 3c, the OER overpotential of ZnCo2O4 micro-rice exhibits almost unchanged during 2 h at the current density of 10 mAcm−2, indicating the good durability of the porous sample in alkaline medium. The ECSA of ZnCo2O4 was evaluated by double-layer capacitance (Cdl) and shown in Fig. 3d, the Cdl value (1.12 mF cm−2) of ZnCo2O4 micro-rice structure is not very big (Fig. 3e). The electrochemical impedance spectroscopy (EIS) of ZnCo2O4 exhibits a small charge transfer resistance of 9.20 Ω (Fig. 3f), revealing that ZnCo2O4 porous structure own fast charge transfer and small charge transfer resistance for OER process.
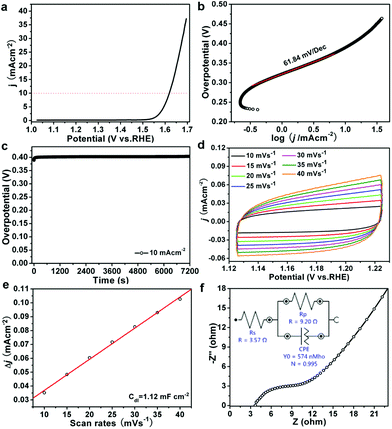 |
| Fig. 3 The catalytic OER process of porous ZnCo2O4 micro-rice sample: (a) LSV curves obtained at a sweep rate of 1 mV s−1, (b) Tafel plots of OER currents in (a). (c) The chronoamperometry curves at the current density of 10 mA cm−2 (d) CVs at different scan rates in 1 M KOH. (e) Plot of current density at 1.17 V (vs. RHE) versus the scan speed. (f) Nyquist plots of porous ZnCo2O4 measured at open circuit potential. | |
3.2 Non-enzymatic glucose sensor
To evaluate the ZnCo2O4 catalytic performance for nonenzymatic glucose sensing, the CVs was conducted at first. The CV curves of ZnCo2O4/GC electrodes were recorded in 0.1 M NaOH solution containing 0–2 mM glucose (Fig. 4a), a quick current density response was observed as the increasing of glucose concentration from 0.1–2 mM, which suggested that the porous ZnCo2O4 modified electrode owns rich active sites for glucose oxidation. Co(II) ions occupy the largest part of the ZnCo2O4 surface are easily converted to Co(OH)2 in alkaline solution, and the Co(OH)2 can be oxidized to CoOOH intermediate, which can electrocatalytic oxidation the glucose to gluconolactone.
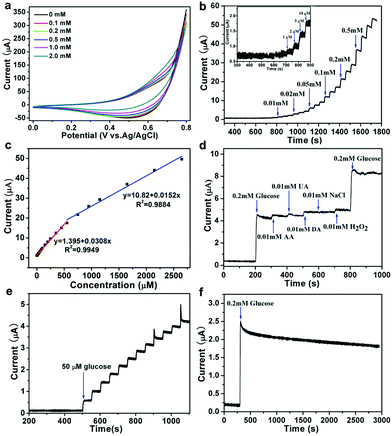 |
| Fig. 4 (a) The CV curves of ZnCo2O4/GC electrode in the absence and presence of 0.1–2 mM glucose. (b) Amperometric responses of the mesoporous ZnCo2O4/GC electrode towards successive addition of glucose at the potential of 0.55 V in 0.1 M NaOH. (c) The calibration curve of current response and glucose concentration. (d) The selectivity and anti-interference. (e) The reproducibility of ZnCo2O4/GC electrode for detection 50 μM glucose. (f) The stability for 2600 s. | |
In 0.10 M NaOH solution, the typical amperometric response of the ZnCo2O4/GC electrode was recorded by the successive addition of glucose at a potential of 0.55 V (Fig. 4b), the modified electrode needs less than 3s to equilibrate each additive amount of glucose. As shown in Fig. 4c, ZnCo2O4/GC not only showed excellent responds in low detection limit, but also in high concentration, the corresponding calibration curve for glucose detection displayed two linearity regions of 0.01–0.55 mM (R2 = 0.9949) and 0.55–2.65 mM (R2 = 0.9884), and the matched sensitivity of ZnCo2O4/GC electrode in low and high concentration regions is 436.1 and 215.1 μA mM−1 cm−2, respectively. Fig. 4b exhibits the detection limit of ZnCo2O4/GC electrode is 5 μM, which is lower than CoOOH nanosheet arrays sensor (30.9 μM),47 Co3O4 nanocrystals sensor (50 μM),49 Co nanobeads/rGO sensor (47.5 μM),56 octahedral Cu2O sensor (128 μM),57 and FeOOH nanowires sensor (15 μM).59 Both the sensitivity values of the constructed sensor electrode are higher than that of the reported Co3O4 porous film electrode (366.03 μA mM−1 cm−2),48 Co3O4 nanofibers modified electrode (36.25 μA mM−1 cm−2),52 Co3O4/NiCo2O4 double-shelled nanocages@G modified electrode (304 μA mM−1 cm−2),53 CuO microspheres modified electrode (349.6 μA mM−1 cm−2),58 CoOOH nanosheet arrays electrode (341 μA mM−1 cm−2),47 and FeOOH nanowires modified electrode (12.13 μA mM−1),59 but lower than that the NiCo2O4 hollow nanorods modified electrode with a sensitivity of 1685.1 μA mM−1 cm−2.60 The performance of the as-prepared ZnCo2O4/GC electrode for glucose sensing is compared with some of recently reported glucose sensors based on Co-based and other transition metal compounds nanomaterials (Table 1).
Table 1 Comparison of the performance of the as-prepared ZnCo2O4 micro-rice sensor with other reported nonenzymatic glucose sensors
Electrode material |
Potential (V vs. Ag/AgCl) |
Linear range (mM) |
Detection limit (μM) |
Sensitivity (μA mM−1 cm−2) |
Ref. |
Cobalt oxide microspheres |
+0.55 |
0.00083–8.61 |
0.46 |
669.78 |
45 |
Porous CoOOH nanosheet arrays |
+0.52 |
0.003–1.109 |
1.37 |
526.8 |
46 |
CoOOH nanosheet arrays |
+0.40 |
0.03–0.7 |
30.9 |
341.0 |
47 |
Co3O4 porous film |
+0.60 |
Up to 3.0 |
1 |
366.03 |
48 |
Co3O4 nanocrystals |
+0.55 |
0.1–0.9 |
50 |
743.6 |
49 |
Co3O4 nanowires |
+0.20 |
0.001–1.2 |
0.265 |
45.8 |
50 |
Nanoporous Co3O4 nanowires |
+0.60 |
0.005–0.57 |
5 |
300.8 |
51 |
Co3O4 nanofibers |
+0.59 |
Up to 2.04 |
0.97 |
36.25 |
52 |
Co3O4/NiCo2O4 double-shelled nanocages@GO |
+0.55 |
0.01–3.54 |
0.384 |
304 |
53 |
Cobalt oxide NP/r-GO |
+0.45 (vs. SCE) |
0.04–4 |
1.44 |
1.21 |
54 |
CuOx–CoOx/graphene |
+0.50 (vs. SCE) |
0.005–0.57 |
0.5 |
507 |
55 |
Co nanobeads/rGO |
+0.55 (vs. SCE) |
0.15–6.25 |
47.5 |
39.32 |
56 |
Octahedral Cu2O |
+0.60 |
0.3–4.1 |
128 |
241 |
57 |
CuO microspheres |
+0.45 (vs. SCE) |
0.001–4 |
0.5 |
349.6 |
58 |
FeOOH nanowires |
+0.40 (vs. SCE) |
0.015–3 |
15 |
12.13 (μA mM−1) |
59 |
NiCo2O4 hollow nanorods |
+0.60 |
0.0003–1 |
0.16 |
1685.1 |
60 |
ZnCo2O4 microrice |
+0.55 |
0.01–0.55 |
5 |
436.1 |
This work |
0.55–2.65 |
215.1 |
The selectivity is also a critical factor to assess the performance of an electrochemical sensor. The common existing interferences such as ascorbic acid (AA), uric acid (UA), and dopamine (DA) in human blood serum were researched to evaluate the selectivity of ZnCo2O4/GC electrode. Fig. 4d shows amperometric response of successive addition of 200 μM glucose, 10 μM AA, UA, DA, 10 μM NaCl, and 200 μM glucose, all the interfering species exhibit negligible responses compared with glucose. The ZnCo2O4/GC electrode exhibit superior selectivity toward glucose. The reproducibility tested by parallel addition of 50 μM glucose for 12 times, the relative stand deviation is 9.4% (Fig. 4e). The stability of the ZnCo2O4/GC was tested for 2600 s via continuous detection of the amperometric response, and it still retained 72% of the initial value (Fig. 4f). The above results of the ZnCo2O4/GC electrode demonstrate low detection limit, high sensitivity and good stability for glucose detection, and it may be used as non-enzymatic glucose sensor in the future.
4 Conclusion
A porous ZnCo2O4 micro-rice like structure was synthesized from a MOF precursor calcinated at a high temperature. The as-prepared ZnCo2O4 sample presented good electrocatalytic OER activity with a small overpotential (η10) and high stability. The excellent electrocatalytic performance of ZnCo2O4 may be attributed to the unique porous structure exposed large active sites and high conductivity of ZnCo2O4. The micro-rice porous structure assembled by nanoparticles can provide many electrocatalytic sites and high ECSA. The result should offer a new designing way to prepare low-cost and high performance electrocatalysts.
Conflicts of interest
There are no conflicts to declare.
Acknowledgements
This work was supported by the National Science Foundation of China (No. 21603004, U1604119, 21501006), the Program for Innovative Research Team of Science and Technology in the University of Henan Province (18IRTSTHN006), and the Natural Science Foundation of Henan Province (182300410194).
Notes and references
- J. R. Li, Y. G. Ma, M. Colin McCarthy, J. Sculley, J. M. Yu, H.-K. Jeong, P. B. Balbuena and H. C. Zhou, Carbon dioxide capture-related gas adsorption and separation in metal-organic frameworks, Coord. Chem. Rev., 2011, 255, 1791–1823 CrossRef.
- Y. B. He, W. Zhou, G. D. Qian and B. L. Chen, Methane storage in metal-organic frameworks, Chem. Soc. Rev., 2014, 43, 5657–5678 RSC.
- W. P. Lustig, S. Mukherjee, N. D. Rudd, A. V. Desai, J. Li and S. K. Ghosh, Metal- organic frameworks: functional luminescent and photonic materials for sensing applications, Chem. Soc. Rev., 2017, 46, 3242–3285 RSC.
- Z. B. Liang, C. Qu, W. H. Guo, R. Q. Zou and Q. Xu, Pristine Metal–Organic Frameworks and their Composites for Energy Storage and Conversion, Adv. Mater., 2018, 30, 1702891 CrossRef.
- R. R. Salunkhe, Y. V. Kaneti, J. Kim, J. H. Kim and Y. Yamauchi, Nano-architectures for Metal−Organic Framework-Derived Nanoporous Carbons toward Supercapacitor Applications, Acc. Chem. Res., 2016, 49, 2796–2806 CrossRef.
- B. Y. Guan, X. Y. Yu, H. B. Wu and X. W. Lou, Complex Nanostructures from Materials based on Metal–Organic Frameworks for Electrochemical Energy Storage and Conversion, Adv. Mater., 2017, 29, 1703614 CrossRef.
- A. Indra, T. Song and U. Paik, Metal Organic Framework Derived Materials: Progress and Prospects for the Energy Conversion and Storage, Adv. Mater., 2018, 30, 1705146 CrossRef.
- H. Zhang, X. M. Liu, Y. Wu, C. Guan, A. K. Cheetham and J. Wang, MOF-derived nanohybrids for electrocatalysis and energy storage: current status and perspectives, Chem. Commun., 2018, 54, 5268–5288 RSC.
- H. D. Mai, K. Rafiq and H. Yoo, Nano Metal-Organic Framework-Derived Inorganic Hybrid Nanomaterials: Synthetic Strategies and Applications, Chem.–Eur. J., 2017, 23, 5631–5651 CrossRef.
- X. H. Cao, C. L. Tan, M. Sindoro and H. Zhang, Hybrid micro-/nano-structures derived from metal–organic frameworks: preparation and applications in energy storage and conversion, Chem. Soc. Rev., 2017, 46, 2660–2677 RSC.
- G. Q. Zou, H. S. Hou, P. Ge, Z. D. Huang, G. G. Zhao, D. L. Yin and X. B. Ji, Metal–Organic Framework-Derived Materials for Sodium Energy Storage, Small, 2018, 14, 1702648 CrossRef.
- S. S. Zhou, C. Hao, J. J. Wang, X. H. Wang and H. W. Gao, Metal-organic framework templated synthesis of porous NiCo2O4/ZnCo2O4/Co3O4 hollow polyhedral nanocages and their enhanced pseudocapacitive properties, Chem.–Eur. J., 2018, 351, 74–84 Search PubMed.
- S. S. Zhou, Z. C. Ye, S. Z. Hu, C. Hao, X. H. Wang, C. X. Huang and F. S. Wu, Designed formation of Co3O4/ZnCo2O4/CuO hollow polyhedral nanocages derived from zeolitic imidazolate framework-67 for high-performance supercapacitors, Nanoscale, 2018, 10, 15771–15781 RSC.
- C. X. Huang, C. Hao, Z. C. Ye, S. S. Zhou, X. H. Wang, L. L. Zhu and J. B. Wu, In situ growth of ZIF-8-derived ternary ZnO/ZnCo2O4/NiO for high performance asymmetric supercapacitors, Nanoscale, 2019, 11, 10114–10128 RSC.
- Q. Zhao, Z. H. Yan, C. C. Chen and J. Chen, Spinels: Controlled Preparation, Oxygen Reduction/Evolution Reaction Application, and Beyond, Chem. Rev., 2017, 117, 1012–10211 CrossRef.
- L. Xu, Q. Q. Jiang, Z. H. Xiao, X. Y. Li, J. Huo, S. Y. Wang and L. M. Dai, Plasma-engraved Co3O4 nanosheets with oxygen vacancies and high surface area for the
oxygen evolution reaction, Angew. Chem., Int. Ed., 2016, 55, 5277–5281 CrossRef CAS.
- Y. Li, F. M. Li, X. Y. Meng, S. N. Li, J. H. Zeng and Y. Chen, Ultrathin Co3O4 Nanomeshes for the Oxygen Evolution Reaction, ACS Catal., 2018, 8, 1913–1920 CrossRef CAS.
- J. C. Zhang, D. J. Zhang, Y. J. Yang, J. Y. Ma, S. F. Cui, Y. M. Li and B. Q. Yuan, Facile synthesis of ZnCo2O4 mesoporous structures with enhanced electrocatalytic oxygen evolution reaction properties, RSC Adv., 2016, 6, 92699–92704 RSC.
- J. C. Zhang, B. Q. Yuan, J. Y. Ma, J. J. Wei, J. J. Wang, J. Y. Zhou, R. C. Zhang and D. J. Zhang, Synthesis of Zn0.3Co2.7O4 porous willow-leaf like structure for enhanced electrocatalytic oxygen evolution reaction, Mater. Lett., 2017, 198, 196–200 CrossRef CAS.
- Q. H. Wang, L. X. Zhu, L. Q. Sun, Y. C. Liu and L. F. Jiao, Facile synthesis of hierarchical porous ZnCo2O4 microspheres for high-performance supercapacitors, J. Mater. Chem. A, 2015, 3, 982–985 RSC.
- Y. Jiao, Y. Zheng, M. Jaroniecb and S. Z. Qiao, Design of electrocatalysts for oxygen- and hydrogen-involving energy conversion reactions, Chem. Soc. Rev., 2015, 44, 2060–2086 RSC.
- W. T. Hong, M. Risch, K. A. Stoerzinger, A. Grimaud, J. Suntivich and Y. Shao- Horn, Toward the rational design of non-precious transition metal oxides for oxygen electrocatalysis, Energy Environ. Sci., 2015, 8, 1404–1427 RSC.
- J. H. Wang, W. Cui, Q. Liu, Z. C. Xing, A. M. Asiri and X. P. Sun, Recent Progress in Cobalt-Based Heterogeneous Catalysts for Electrochemical Water Splitting, Adv. Mater., 2016, 28, 215–230 CrossRef CAS.
- X. X. Zou and Y. Zhang, Noble metal-free hydrogen evolution catalysts for water splitting, Chem. Soc. Rev., 2015, 44, 5148–5180 RSC.
- Z. L. Wang, D. Xu, J. J. Xu and X. B. Zhang, Oxygen electrocatalysts in metal–air batteries: from aqueous to nonaqueous electrolytes, Chem. Soc. Rev., 2014, 43, 7746–7786 RSC.
- D. J. Yang, L. J. Zhang, X. C. Yan and X. D. Yao, Recent Progress in Oxygen Electrocatalysts for Zinc–Air Batteries, Small Methods, 2017, 1, 1700209 CrossRef.
- J. Fu, Z. P. Cano, M. G. Park, A. P. Yu, M. Fowler and Z. W. Chen, Electrically Rechargeable Zinc-Air Batteries: Progress, Challenges, and Perspectives, Adv. Mater., 2017, 29, 1604685 CrossRef.
- Y. W. Liu, C. Xiao, M. J. Lyu, Y. Lin, W. Z. Cai, P. C. Huang, W. Tong, Y. M. Zou and Y. Xie, Ultrathin Co3S4 nanosheets that synergistically engineer spin states and exposed polyhedra that promote water oxidation under neutral conditions, Angew. Chem., Int. Ed., 2015, 54, 11231–11235 CrossRef CAS.
- M. R. Gao, X. Cao, Q. Gao, Y. F. Xu, Y. R. Zheng, J. Jiang and S. H. Yu, Nitrogen-doped graphene supported CoSe2 nanobelt composite catalyst for efficient water oxidation, ACS Nano, 2014, 8, 3970–3978 CrossRef CAS.
- M. Q. Yu, L. X. Jiang and H. G. Yang, Ultrathin nanosheets constructed CoMoO4 porous flowers with high activity for electrocatalytic oxygen evolution, Chem. Commun., 2015, 51, 14361–14364 RSC.
- J. C. Zhang, Y. Yang, Z. C. Zhang, X. B. Xu and X. Wang, Rapid synthesis of mesoporous NixCo3-x(PO4)2 hollow shells showing enhanced electrocatalytic and supercapacitor performance, J. Mater. Chem. A, 2014, 2, 20182–20188 RSC.
- K. Dhara and D. R. Mahapatra, Electrochemical nonenzymatic
sensing of glucose using advanced nanomaterials, Microchim. Acta, 2018, 185, 49 CrossRef.
- G. F. Wang, X. P. He, L. L. Wang, A. X. Gu, Y. Huang, B. Fang, B. Y. Geng and X. J. Zhang, Non-enzymatic electrochemical sensing of glucose, Microchim. Acta, 2013, 180, 161–186 CrossRef CAS.
- P. Si, Y. J. Huang, T. H. Wang and J. M. Ma, Nanomaterials for electrochemical non-enzymatic glucose biosensors, RSC Adv., 2013, 3, 3487–3502 RSC.
- F. Y. Xie, X. Q. Cao, F. L. Qu, A. M. Asirie and X. P. Sun, Cobalt nitride nanowire array as an efficient electrochemical sensor for glucose and H2O2 detection, Sens. Actuators, B, 2018, 255, 1254–1261 CrossRef CAS.
- Y. H. Song, C. T. Wei, J. He, X. Li, X. P. Lu and L. Wang, Porous Co nanobeads/rGO nanocomposites derived from rGO/Co-metal organic frameworks for glucose sensing, Sens. Actuators, B, 2015, 220, 1056–1063 CrossRef CAS.
- L. Wang, Y. L. Zheng, X. P. Lu, Z. Li, L. L. Sun and Y. H. Song, Dendritic copper- cobalt nanostructures/reduced grapheme oxide-chitosan modified glassy carbon electrode for glucose sensing, Sens. Actuators, B, 2014, 195, 1–7 CrossRef CAS.
- Y. W. Liu, X. Q. Cao, R. M. Kong, G. Du, A. M. Asiri, Q. Lu and X. P. Sun, Cobalt phosphide nanowire array as an effective electrocatalyst for non-enzymatic glucose sensing, J. Mater. Chem. B, 2017, 5, 1901–1904 RSC.
- G. L. Li, H. H. Huo and C. L. Xu, Ni0.31Co0.69S2 nanoparticles uniformly anchored on a porous reduced graphene oxide framework for a high-performance non- enzymatic glucose sensor, J. Mater. Chem. A, 2015, 3, 4922–4930 RSC.
- Y. Shu, B. Li, J. Y. Chen, Q. Xu, H. Pang and X. Y. Hu, Facile Synthesis of Ultrathin Nickel−Cobalt Phosphate 2D Nanosheets with Enhanced Electrocatalytic Activity for Glucose Oxidation, ACS Appl. Mater. Interfaces, 2018, 10, 2360–2367 CrossRef CAS.
- Y. Y. Su, B. B. Luo and J. Z. Zhang, Controllable Cobalt Oxide/Au Hierarchically Nanostructured Electrode for Nonenzymatic Glucose Sensing, Anal. Chem., 2016, 88, 1617–1624 CrossRef CAS.
- W. Huang, Y. Cao, Y. Chen, J. Peng, X. Y. Lai and J. C. Tu, Fast synthesis of porous NiCo2O4 hollow nanospheres for a high-sensitivity non-enzymatic glucose sensor, Appl. Surf. Sci., 2017, 396, 804–811 CrossRef CAS.
- Y. J. Sa, K. Kwon, J. Y. Cheon, F. Kleitzc and S. H. Joo, Ordered Mesoporous Co3O4 Spinels as Stable, Bifunctional, Noble Metal-Free Oxygen Electrocatalysts, J. Mater. Chem. A, 2013, 1, 9992–10001 RSC.
- Y. K. Zhao, X. C. Zhou, Y. Ding, J. W. Huang, M. Zheng and W. C. Ye, A study of photocatalytic, chemical, and electrocatalytic water oxidation on ACo2O4 (A = Ni, Cu, Zn) samples through doping different metal ions, J. Catal., 2016, 338, 30–37 CrossRef CAS.
- S. Q. Ci, S. Mao, T. Z. Huang, Z. H. Wen, D. A. Steeber and J. H. Chen, Enzymeless Glucose Detection Based on CoO/Graphene Microsphere Hybrids, Electroanal, 2014, 26, 1326–1334 CrossRef CAS.
- L. Zhang, C. L. Yang, G. Y. Zhao, J. S. Mu and Y. Wang, Self-supported porous CoOOH nanosheet arrays as a non-enzymatic glucose sensor with good reproducibility, Sens. Actuators, B, 2015, 210, 190–196 CrossRef CAS.
- K. K. Lee, P. Y. Loh, C. H. Sow and W. S. Chin, CoOOH nanosheets on cobalt substrate as a non-enzymatic glucose sensor, Electrochem. Commun., 2012, 20, 128–132 CrossRef CAS.
- S. S. Fan, M. G. Zhao, L. J. Ding, J. J. Liang, J. Chen, Y. C. Li and S. G. Chen, Synthesis of 3D hierarchical porous Co3O4 film by eggshell membrane for non-enzymatic glucose detection, J. Electroanal. Chem., 2016, 775, 52–57 CrossRef CAS.
- M. Li, C. Han, Y. F. Zhang, X. J. Bo and L. P. Guo, Facile synthesis of ultrafine Co3O4 nanocrystals embedded carbon matrices with specific skeletal structures as efficient non-enzymatic glucose sensors, Anal. Chim. Acta, 2015, 861, 25–35 CrossRef CAS.
- K. Khuna, Z. H. Ibupoto, X. Liu, V. Beni and M. Willander, The ethylene glycol template assisted hydrothermal synthesis of Co3O4 nanowires; structural characterization and their application as glucose non-enzymatic sensor, Mater. Sci. Eng., B, 2015, 194, 94–100 CrossRef.
- L. Q. Kang, D. P. He, L. L. Bie and P. Jiang, Nanoporous cobalt oxide nanowires for non-enzymatic electrochemical glucose detection, Sens. Actuators, B, 2015, 220, 888–894 CrossRef CAS.
- Y. Ding, Y. Wang, L. Su, M. Bellagamba, H. Zhang and Y. Lei, Electrospun Co3O4 nanofibers for sensitive and selective glucose detection, Biosens. Bioelectron., 2010, 26, 542–548 CrossRef CAS.
- B. Xue, K. Z. Li, L. Feng, J. H. Lu and L. L. Zhang, Graphene wrapped porous Co3O4/NiCo2O4 double-shelled nanocages with enhanced electrocatalytic performance for glucose sensor, Electrochim. Acta, 2017, 239, 36–44 CrossRef CAS.
- H. Heidari and E. Habibi, Amperometric enzyme-free glucose sensor based on the use of a reduced graphene oxide paste electrode modified with electrodeposited cobalt oxide nanoparticles, Microchim. Acta, 2016, 183, 2259–2266 CrossRef CAS.
- S. J. Li, L. L. Hou, B. Q. Yuan, M. Z. Chang, Y. Ma and J. M. Du, Enzyme-free glucose sensor using a glassy carbon electrode modified with reduced graphene oxide decorated with mixed copper and cobalt oxides, Microchim. Acta, 2016, 183, 1813–1821 CrossRef CAS.
- Y. H. Song, C. T. Wei, J. He, X. Li, X. P. Lu and L. Wang, Porous Co nanobeads/rGO nanocomposites derived from rGO/Co-metal organic frameworks for glucose sensing, Sens. Actuators, B, 2015, 220, 1056–1063 CrossRef CAS.
- Y. C. Li, Y. M. Zhong, Y. Y. Zhang, W. Weng and S. X. Li, Carbon quantumdots/octahedral Cu2O nanocomposites for non-enzymatic glucose and hydrogen peroxide amperometric sensor, Sens. Actuators, B, 2015, 206, 735–743 CrossRef CAS.
- X. D. Liu, Y. Yang, R. Y. Liu, Z. L. Shi, L. Y. Ma and M. Wei, Synthesis of porous CuO microspheres assembled from (001) facet exposed nanocrystals with excellent glucose-sensing performance, J. Alloys Compd., 2017, 718, 304–310 CrossRef.
- C. Xia and W. Ning, A novel non-enzymatic electrochemical glucose sensor modified with FeOOH nanowire, Electrochem. Commun., 2010, 12, 1581–1584 CrossRef.
- J. Yang, M. Cho and Y. Lee, Synthesis of hierarchical NiCo2O4 hollow nanorods via sacrificial-template accelerate hydrolysis for electrochemical glucose oxidation, Biosens. Bioelectron., 2016, 75, 15–22 CrossRef.
Footnote |
† Electronic supplementary information (ESI) available: Size distribution of the Zn–Co-MOF precursor and the calcined sample, the control experiment of Zn–Co-MOF synthesis, TEM images of Zn–Co-based MOF, TG curves, the XPS survey spectrum of ZnCo2O4. See DOI: 10.1039/c9ra08723k |
|
This journal is © The Royal Society of Chemistry 2020 |
Click here to see how this site uses Cookies. View our privacy policy here.