DOI:
10.1039/C9RA08974H
(Paper)
RSC Adv., 2020,
10, 13872-13878
Renal-clearable hyaluronic acid functionalized NaGdF4 nanodots with enhanced tumor accumulation†
Received
31st October 2019
, Accepted 30th March 2020
First published on 6th April 2020
Abstract
Integration of high tumor-targeting capacity, controlling in vivo transport and low normal tissue retention into one engineered nanoparticle is a critical issue for future clinically translatable anti-cancer nanomedicines. Herein, hyaluronic acid functionalized 3.8 nm NaGdF4 nanodots (named NaGdF4 ND@HAs) have been prepared through conjugation of tryptone capped NaGdF4 nanodots (NaGdF4 ND@tryptone) with hyaluronic acid (HA, a naturally occurring glycosaminoglycan), which can recognize the overexpressed CD44 on cancer cell membranes. The as-prepared NaGdF4 ND@HAs have good paramagnetic properties (longitudinal relaxivity (r1) = 7.57 × 10−3 M S−1) and low cytotoxicity. The in vivo experimental results demonstrate that the NaGdF4 ND@HAs can not only efficiently accumulate in mouse-bearing MDA-MB-231 tumors (ca. 5.3% injection dosage (ID) g−1 at 2 h post-injection), but also have an excellent renal clearance efficiency (ca. 75% injection dosage (ID) at 24 h post-injection). The as-prepared NaGdF4 ND@HAs have good paramagnetic properties with enhanced tumor-targeting capacity, which provides a useful strategy for the preparation of renal clearable magnetic resonance imaging (MRI) contrast agents for tumors.
Introduction
As one of the prospective directions of contemporary medical sciences, nanomaterial-based theranostics (known as nanomedicines) have been intensively studied because of their potential applications in various domains including diagnosis of and therapies for diseases (e.g., tumor), tracking the location of biomarkers/signal molecules in vivo, and evaluating their therapeutic effect.1–10 To date, more than one hundred nanomedicines have been approved by the Food and Drug Administration (FDA) in the USA, or are in the FDA clinical trial stage.4 However, it is a great challenge to translate nanomedicines from pre-clinical proof-of-concept to clinical applications because there is an increasing consideration of the biosafety of nanomedicines.11–18 Currently, many promising nanomedicines normally eliminated through the hepatobiliary route are constructed from relatively large sized (>10 nm in diameter) and nonbiodegradable inorganic nanoparticles.16–19 However, the interactions of nanomedicines with mononuclear phagocyte system (MPS) cells (e.g., Kupffer cells) in spleen and liver significantly increase their retention time in vivo (even more than months), which causes potential toxicity.11–19 The biosafety concern can be addressed by the development of nanomedicines that undergo renal clearance.20–22 During the last few years, several ultra-small sized inorganic nanoparticles (<5.5 nm) have been employed to generate renal clearable nanomedicines for the diagnoses and therapies of various diseases including cancers.23–33 For instance, Zheng's group has been developed a series of gold nanoparticles/nanoclusters for delivering anticancer drugs,30 evaluating kidney function,31 and imaging tumors.32,33
As a powerful tool, magnetic resonance imaging (MRI) has been extensively applied for non-invasive diagnoses of various diseases through producing excellent soft-tissue contrast. Compared with other modalities of medical imaging, the sensitivity of MRI is poor. Because trivalent gadolinium ion (Gd3+) has seven unpaired electrons with a large magnetic moment, Gd-chelates and Gd nanoparticles have been employed as contrast agents for enhancing the sensitivity of T1-weighted MRI.34–38 Among Gd-based T1-weighted MRI contrast agents, ultrasmall Gd nanoparticles (also known as Gd nanodots (Gd NDs)) not only have high contrast enhancement capability, but also can be eliminated from body through renal clearance.39–47 The Gd NDs are normally coated hydrophilic or amphiphilic ligands for improving their colloidal stability and biocompatibility, then further modified with specific biomolecules for generating high tumor-targeting ability. As a major component of extracellular matrices, hyaluronic acid (hyaluronan, HA) has high binding affinity with cell surface receptor, CD44.48–50 Therefore, HA can be used as an active tumor-targeting ligand for constructing drug delivery systems since tumor cells normally express high level of CD44.50–54 For example, Parayath and coauthors have fabricated hyaluronic acid–poly(ethylenimine) (HA–PEI)-based nanoparticles encapsulating miR-125b for anticancer immunotherapy through the interactions of HA with CD44.53 In addition, Guo et al. have found that Gd3+-labeled peptide dendron-HA conjugate-based hybrid (dendronized-HA-DOTA-Gd) has better biocompatibility and higher accumulation in tumors than those of Gd-DTPA.51 The results suggest that HA might be employed as an active tumor-targeting ligand for generating renal clearable Gd NDs.
In this study, a highly renal-clearable HA functionalized NaGdF4 nanodots (NaGdF4 ND@HAs) were synthesized by a two-step reaction, and evaluated as an active tumor-targeting MRI contrast agent. The tryptone was employed as phase transfer agent for transferring hydrophobic oleic acid coated NaGdF4 nanodots (NaGdF4 ND@OAs, 3.8 nm in diameter) through the Gd–phosphate coordination reaction. HA was then conjugated with the tryptone coated NaGdF4 NDs (NaGdF4 ND@tryptone) by the use of 1-ethyl-3-(3-dimethylaminopropyl)carbodiimide (EDC) as coupling agent. Both in vitro cellular studies and in vivo small animal experiments of mouse-bearing MDA-MB-231 breast cancer model demonstrated that NaGdF4 ND@HAs exhibit low toxicity and can be used for specific detection of tumors with abundant HA receptor.
Experimental section
Materials
Tryptone was purchased from Oxoid Ltd (Hampshire, England). Oleic acid (OA, 90%), 1-octadecene (ODE, 90%) were obtained from Sigma-Aldrich Co. (St. Louis, USA). The GdCl3·6H2O was purchased from Beijing HWRK Chem Co. Ltd (Beijing, China). The culture medium Leibovitz L-15 and DMEM were purchased from Jiangsu KeyGen Biotech Co. Ltd (Jiangsu, China). The fetal bovine serum (FBS) was obtained from Gibco Co. (New York, USA). Trypsin–EDTA digest and 3-(4,5-dimethyl-2-thiazolyl)-2,5-diphenyl-2-H-tetrazolium bromide (MTT) were purchased from Beijing Dingguo Biotechnology Ltd (Beijing, China). Hyaluronic acid (HA, 4.2 kDa) was purchased from Bloomage Freda Biopharma Co., Ltd (Jinan, China). Other reagents (analytical grade) were purchased from Beijing Chemical Reagent Co. (Beijing, China), which were used without any purification. Deionized H2O (18.2 MΩ cm) were used in all experiments. All animal procedures were carried out with the procedures approved by the local Animal Research Committee at Jilin University (ethical approve document number: 2019515). Female Balb/c nude mice and Balb/c mice (ca., average body weight of 20 g) were purchased from Liaoning Changsheng Biotechnology Ltd (Liaoning, China). The mice having free access to food and water were kept for 12 h in light and 12 h in dawn daily at 20 °C.
Characterizations
TEM micrographs were recorded by TECNAI G2 high-resolution TEM (FEI Co., USA). Dynamic light scattering and zeta potential of the as-prepared samples were carried out on a Zetasizer Nano ZS (Malvern Instruments Ltd, UK). The analysis of elements was conducted with an ELAN 9000/DRC ICP-MS system (PerkinElmer, USA). The relaxation times of the samples were carried out on a Siemens Prisma 3.0 T MR scanner (Erlangen, Germany). Energy-dispersive X-ray spectra (EDS) were inspected on an energy dispersive spectroscopy (FEI Co., USA). X-ray diffraction analysis were carried out on a D8 ADVANCE diffractometer (Bruker Co., Germany) using Cu Kα (0.15406 nm) radiation. The infrared spectra were conducted with a Vertex 70 Fourier transform infrared (FTIR) spectrometer (Bruker, Germany). XPS measurements were conducted with a VG ESCALAB MKII X-ray photoelectron spectrometer (VG Scientific Ltd, UK) spectroscopy (XPS). Siemens Prisma 3.0 T MR scanner (Erlangen, Germany) was employed to acquire T1-weighted MR images.
Synthesis of NaGdF4 ND@HAs
The NaGdF4 ND@OAs were synthesized by previously reported methods (see ESI† for details).40,43,47 Subsequently, 10 mL NaGdF4 ND@OA cyclohexane solution (1 mg mL−1) were mixed with 10 mL tryptone aqueous solution (6.4 mg mL−1) under vigorously stirred, and incubated at room temperature for 12 h. Then, the as-prepared tryptone capped NaGdF4 nanodots (NaGdF4 ND@tryptone) were purified by centrifugation (13
000 rpm, 3 times) and redispersed in H2O. 0.5 mL 1-ethyl-3-(3-(dimethylaminopropyl)carbodiimide solution (EDC, 4 mg mL−1 in MES (2-(N-morpholino)ethanesulfonic acid) buffer) and 0.5 mL N-hydroxysulfo-succinimide solution (sulfo-NHS, 6 mg mL−1 in MES buffer) were mixed with 2 mL HA solution (2.5 mg mL−1 in MES buffer). After incubation at room temperature for 1 h, 2 mL NaGdF4 ND@tryptone (2.5 mg mL−1) in PBS (phosphate buffer saline, pH 7.4)) were added into the mixture and continuously incubated at 37 °C for 3 h. Finally, the as-prepared NaGdF4 ND@HAs were purified by centrifugation (10
000 rpm, 3 times) and redispersed in PBS (pH 7.4).
Cytotoxicity study
The MDA-MB-231 breast cancer cells, MCF-7 breast cancer cells and normal kidney tissue cells (293 cells) were obtained from Shanghai Cell Bank, CAS (Shanghai, China). MDA-MB-231 breast cancer cells were cultured with fresh L-15 culture medium supplemented with 10% FBS in a 96 well plate (1 × 104 cells in 100 μL per well) under full air conditions at 37 °C for 24 h, MCF-7 breast cancer cells and normal kidney tissue cells (293 cells) were cultured with fresh DMEM culture medium supplemented with 10% FBS and 100 U mL−1 penicillium–streptomycin in a 96 well plate (1 × 104 cells in 100 μL per well) under 5% CO2 air conditions at 37 °C for 24 h. Subsequently, those cells were washed by PBS. Next, 100 μL fresh culture medium containing various concentrations (6.25, 12.5, 25, 50, 100 and 200 μg mL−1) of NaGdF4 ND@tryptone or NaGdF4 ND@HAs were introduced into wells, and cultured for another 24 h. After fully washed by PBS (100 μL, 3 times), the cell viabilities were detected by traditional MTT (3-(4,5-dimethyl-2-thiazolyl)-2,5-diphenyl-2-H-tetrazolium bromide) assay.
In vitro MRI study
The MDA-MB-231 and MCF-7 cells were cultured at 37 °C for 24 h and divided into 4 groups ((1) MCF-7 + NaGdF4 ND@HAs; (2) free HAs (2 mg mL−1) + MDA-MB-231 incubated for 4 h, and then incubated with NaGdF4 ND@HAs; (3) MDA-MB-231 + NaGdF4 ND@tryptone; (4) MDA-MB-231 + NaGdF4 ND@HAs), which were co-cultured with different concentrations of NaGdF4 ND@tryptone or NaGdF4 ND@HAs in 2 mL fresh culture medium containing different concentrations of NaGdF4 NDs at 37 °C for 24 h, respectively. After washed with fresh culture medium (1 mL, 3 times) and PBS (1 mL, 3 times), the NaGdF4 ND stained cells were digested by 1 mL trypsin and centrifuged at 2000 rpm for 5 min. Subsequently, the as-obtained cell pellets were fixed by 1% agarose gel, and imaged by a Siemens 1.5 T MRI scanner with imaging parameters: 1.2 mm slice thickness, 15 ms echo time (TE), 358 ms repetition time (TR) and 50 mm × 50 mm field of view. In addition, 3 × 103 NaGdF4 ND stained cells were treated by 2 mL aqua regia. The amounts of Gd element in the NaGdF4 ND stained cells were measured by inductively coupled plasma mass spectrometry (ICP-MS, ELAN 9000/DRC, PerkinElmer Co., USA), respectively.
In vivo MRI study
Female Balb/c nude mice and Balb/c mice with average body weight of 20 g were purchased from Liaoning Changsheng Biotechnology Ltd (Liaoning, China). The mice having free access to food and water were kept for 12 h in light/12 h in dawn daily at 20 °C. All animal procedures were approved by the Local Ethics Committee for Institutional Animal Care and Use of Jilin University. MDA-MB-231 cells (1 × 106 cells in 100 μL PBS) were subcutaneously injected into the hind flank of female Balb/c nude mouse. The volume of tumor (V) was evaluated by the following formula: V = length × (width)2/2. When V reached about 60 mm3, the MDA-MB-231 tumor-bearing nude mice were injected intravenously with 100 μL 0.9 wt% NaCl solution containing 2 mg mL−1 (Gd3+ content) NaGdF4 ND@tryptone or NaGdF4 ND@HAs through tail veins. The in vivo MR images of tumors were taken at 0, 0.5, 2, 4, 8, 12, and 24 h post-injection by Siemens 3.0 T MRI scanner with the following scanning parameters: 1.2 mm slice thickness, 3000 ms TR, 9.1 ms TE and 120 mm × 72 mm field of view. In addition, the mice were sacrificed at 2 h post-injection, and the main organs as well as tumors were collected for ICP-MS measurement.
Biocompatibility analysis
The healthy female Balb/c mice were randomly divided into 2 groups: control group and NaGdF4 ND@HAs treated group. The mice in treated group were injected intravenously with 100 μL 0.9 wt% NaCl solution containing 10 mg kg−1 (Gd3+ content) NaGdF4 ND@HAs, respectively. The mice in control group were only injected intravenously with 100 μL 0.9 wt% NaCl solution respectively. The body weights of mice were measured every 2 days until 30 days after injection. The mice were sacrificed at the 1st and the 30th day post-injection, and main organs including heart, liver, spleen, lung and kidneys were fixed in 4% (w/v) paraformaldehyde solution, embedded in paraffin, sectioned, and finally stained with hematoxylin–eosin (H&E). Meanwhile, the tumors were fixed in 4% (w/v) paraformaldehyde solution, embedded in paraffin, sectioned, and finally stained by hematoxylin–eosin (H&E) and anti-CD44v6 immunohistochemistry. The blood samples of mice were collected at the 1st and the 30th day post-injection, also analysed by the blood routine assay.
Results and discussion
Synthesis and characterization of NaGdF4 ND@HAs
The synthetic route and application of NaGdF4 ND@HAs is shown in Scheme 1. The hydrophobic NaGdF4 ND@OAs (3.8 ± 0.4 nm in diameter) were prepared by previously reported procedure with a slight modification (as shown in Fig. 1a).40,43,47 As the digestion product of casein, tryptone contains ca. 10–20% casein phosphopeptide (CPP) with the sequence –Ser(P)–Ser(P)–Ser(P)–Glu–Glu–, which can form robust Gd3+–phosphate coordination bonds under mild conditions through the reaction of phosphoseryl serine residue (Ser(P)) and trivalent Gd ions.43,47 After mixing NaGdF4 ND@OAs with tryptone, hydrophilic NaGdF4 ND@tryptone were generated through replacing the original OA ligand of NaGdF4 ND@OAs by tryptone. Subsequently, HA was activated by EDC and sulfo-NHS, also conjugated on NaGdF4 ND@tryptone surface through the amidation reaction between carboxy group of HA and amine group of tryptone. HA and tryptone, which have been extensively used for producing drugs and health care products, are raw materials approved by the US Food and Drug Administration (FDA). Therefore, the toxicity of NaGdF4 ND can be reduced by HA and tryptone coating. After ligand exchange and HA functionalization, the morphology, size and crystalline nature of NaGdF4 NDs exhibit negligible changes (as shown in Fig. 1b and c). The hydrodynamic diameter and zeta potential of NaGdF4 ND@tryptone were 11.99 nm and −5.86 mV, respectively. The result was consistent with the structure of NaGdF4 ND@tryptone which contains individual solid NaGdF4 ND core and a flexible phosphopeptide outlayer. The phenomenon suggested that NaGdF4 ND@tryptone exhibits good monodispersity and negative surface charge. Because HA is negatively charged biomacromolecule, the hydrodynamic diameter of NaGdF4 ND@HAs was increased to 29.05 nm, while the zeta potential of NaGdF4 ND@HAs was deceased to −12.16 mV. The negative surface charge helps to reduce the nonspecific interactions of NaGdF4 NDs with cells. The successful preparation of NaGdF4 ND@tryptone and NaGdF4 ND@HAs were also investigated by XPS, EDS and FTIR. After incubation with tryptone, the phosphorus and nitrogen peaks were clearly observed in the XPS (P 2p (133 eV) and N 1s (400 eV)) and EDS (P (2.01 keV) and N (0.39 keV)) spectra of NaGdF4 NDs (as shown in Fig. S1 and S2†).43,47,55 In addition, the XPS spectrum of NaGdF4 ND@HAs exhibited relatively high intensity of C 1s (284 eV). Compared to NaGdF4 ND@OAs, two additional IR bands at 683 cm−1 and 1080 cm−1 are observed in FTIR spectrum of NaGdF4 ND@tryptone (as shown in Fig. S3†), which are corresponded to the out-of-plane bending vibration of C–H bond on benzene ring and antisymmetric bending mode of PO43−, respectively.56 A new IR band at 1010 cm−1 is observed in FTIR spectrum of NaGdF4 ND@HAs (as shown in Fig. S3†), which is corresponded to stretching vibration of C–O band of primary alcohol of HA.57 As shown in Fig. 2, the longitudinal relaxivity (r1) value (7.57 mM−1 S−1) of NaGdF4 ND@HAs is higher than those of NaGdF4 ND@tryptone (6.03 mM−1 S−1) as well as commercial Gd3+ chelates (e.g., (4.3 mM−1 S−1)). The high r1 value of NaGdF4 ND@HAs may stem from the strong polarity of HA molecule which may cause a spatial agglomeration of water around the Gd3+, leading to boost the relaxivity to high value. The result indicates that NaGdF4 ND@HAs can be used as efficient T1-weighted MRI contrast agent.
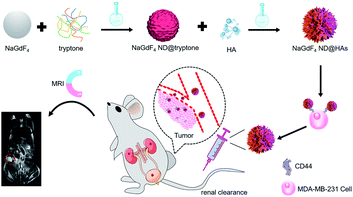 |
| Scheme 1 Schematic diagram of NaGdF4 ND@HAs synthesis, and the application in MRI of tumor through recognizing the overexpressed CD44 on cancer cell membrane. | |
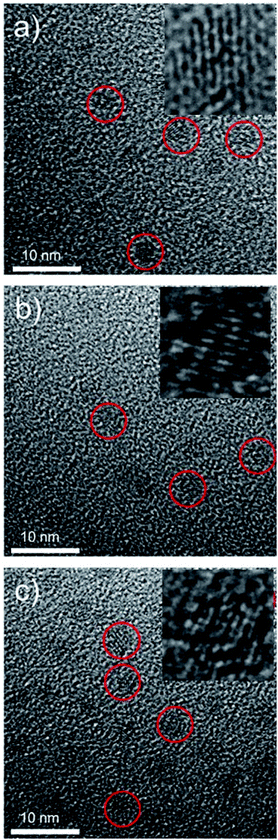 |
| Fig. 1 Transmission electron microscope micrographs (TEM) of (a) NaGdF4 ND@OAs, (b) NaGdF4 ND@tryptone and (c) NaGdF4 ND@HAs, respectively. Insets are corresponding HRTEM micrographs of NaGdF4. | |
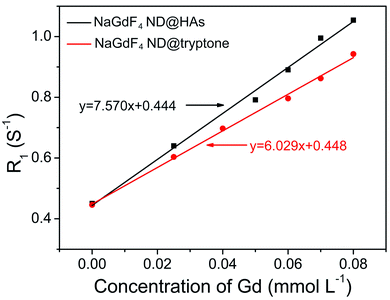 |
| Fig. 2 R1 relaxivities of NaGdF4 ND@tryptone and NaGdF4 ND@HAs as a function of the molar concentration of Gd3+ in solution, respectively. The slope of line represents the longitudinal relaxivity (r1) value. | |
The interactions of NaGdF4 ND with living cells
Human triple-negative breast carcinoma MDA-MB-231 cell line was selected as a typical model because it expresses high level of CD44.58 As shown in Fig. S4,† the MDA-MB-231, MCF-7 and 293 cells exhibit higher than 90% viability after incubated with up to 200 μg mL−1 NaGdF4 ND@HAs or NaGdF4 ND@tryptone for 24 h. The result indicates that both of NaGdF4 ND@HAs and NaGdF4 ND@tryptone have low cytotoxicity. The T1-weighted MR signal intensity of NaGdF4 ND@HAs stained MDA-MB-231 cells was stronger than which of NaGdF4 ND@tryptone stained MDA-MB-231 cells or NaGdF4 ND@HAs stained MCF-7 (as shown in Fig. 3a–c). The T1-weighted MR signal intensity (Fig. 3, group 2) of NaGdF4 ND@HAs stained HA treated MDA-MB-231 cells is much lower than which of NaGdF4 ND@HAs stained untreated MDA-MB-231 cells (Fig. 3, group 4). In addition, the cellular internalization amount of NaGdF4 ND@HAs is much higher than which of NaGdF4 ND@tryptone (as shown in Fig. 3d). The results demonstrate that the NaGdF4 ND@HAs have high affinity with MDA-MB-231 cells, and can be used to recognize CD44-overexpression cells with high specificity.
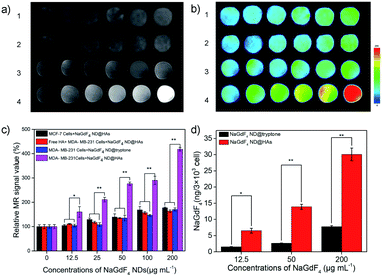 |
| Fig. 3 (a) MR images of NaGdF4 ND stained cells. ((1) MCF-7 cells + NaGdF4 ND@HAs; (2) free HA + MDA-MB-231 cells + NaGdF4 ND@HAs; (3) MDA-MB-231 cells + NaGdF4 ND@tryptone; (4) MDA-MB-231 cells + NaGdF4 ND@HA), (b) corresponding pseudo-color images and (c) corresponding data analysis of NaGdF4 ND stained cells. The cells were incubated with 0, 12.5, 25, 50, 100, 200 μg mL−1 NaGdF4 ND@tryptone or NaGdF4 ND@HAs from left to right, respectively. (d) The amounts of Gd element in the NaGdF4 ND stained cells. Error bars mean standard deviations (n = 5, *P < 0.05 or **P < 0.01 from an analysis of variance with Tukey's post-test). | |
In vivo MRI of MDA-MB-231 tumor
A Balb/c nude mouse bearing MDA-MB-231 tumor model was established for evaluating the active tumor-targeting capacity of NaGdF4 ND@HAs. Both of NaGdF4 ND@HAs and NaGdF4 ND@tryptone (10 mg Gd kg−1 body weight in 0.9 wt% NaCl) were intravenously injected into the mouse bearing MDA-MB-231 tumor through tail vein and the MR signals of tumor sites were recorded at desired timed intervals within 24 h post-injection. The NaGdF4 ND@tryptone can be accumulated in tumor site through enhanced permeability and retention (EPR) effect (i.e., passive tumor-targeting), while NaGdF4 ND@HAs can be accumulated in tumor site by EPR effect and binding of HA with CD44 (i.e., active tumor-targeting). As expected, the MR signals of the tumor sites gradually increased by numbers in between 0 and 2 h post-injection (as shown in Fig. 4). Maximum MR contrast enhancement is obtained at 2 h post-injection of NaGdF4 NDs. In particular, the MR signal intensity in tumor site of NaGdF4 ND@HAs treated mouse is higher than which of NaGdF4 ND@tryptone treated mouse at the same post-injection time point. The maximum MR contrast enhancement in tumor site of NaGdF4 ND@HAs treated mouse is 1.6 times higher than which of the NaGdF4 ND@tryptone treated mouse. The phenomenon may due to high binding affinity of HA with over expressed CD44 on MDA-MB-231 cells. The result demonstrated that the as-prepared NaGdF4 ND@HAs can be severed as an excellent T1-weighted MRI contrast agent for detection of CD44-overexpression tumors (e.g., triple-negative breast cancer). The active tumor-targeting capacity of NaGdF4 ND@HAs was also confirmed by ICP-MS measurement. As shown in Fig. 4d, the amounts of Gd in kidneys and tumors were relatively higher than in other organs, indicating that both NaGdF4 ND@tryptone and NaGdF4 ND@HAs can be efficiently accumulated in tumor sites and excreted by renal clearance. In particular, the Gd content in tumor of NaGdF4 ND@HAs treated mouse treated mouse was 1.87 times higher than which of NaGdF4 ND@tryptone treated mouse. The result indicated that the accumulation amount of NaGdF4 ND@HAs in tumor site is clearly improved through EPR effect as well as recognition of HA receptors of tumor cells.
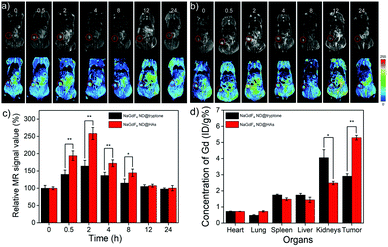 |
| Fig. 4 In vivo MR images and corresponding pseudo color images of Balb/c mouse bearing MDA-MB-231 tumor after intravenous injection of (a) NaGdF4 ND@tryptone or (b) NaGdF4 ND@HAs (10 mg Gd kg−1 body) at different timed intervals (0 (pre-injection), 0.5, 2, 4, 8, 12 and 24 h post-injection), respectively. (c) Corresponding data analysis of MR measurements. The tumor site was marked by circle. (d) The amounts of NaGdF4 NDs in main organs at 2 h post-injection. Error bars mean standard deviations (n = 5, *P < 0.05 or **P < 0.01 from an analysis of variance with Tukey's post-test). | |
In vivo biodistribution and toxicology of NaGdF4 ND@HAs
For evaluating its biodistribution and clearance pathway, NaGdF4 ND@HAs (10 mg Gd kg−1 body weight in 0.9 wt% NaCl) were injected into healthy Balb/c mice through tail vein. The MR signals in the liver, kidneys and bladder were recorded at different timed intervals of post-injection (as shown in Fig. 5a and S5†). The MR signal of liver exhibited little change during the whole period, indicating low accumulation of NaGdF4 ND@HAs in liver. MR signals in the kidneys and bladder were clearly enhanced within 24 h post-injection, and recovered to pre-injection levels after 24 h post-injection. The result demonstrated that NaGdF4 ND@HAs are excreted from the body by renal clearance. After intravenous injection of NaGdF4 ND@HAs, the Gd content in urine of mouse was measured for addressing the pharmacokinetics behavior of NaGdF4 ND@HAs (as shown in Fig. 5b). The total of Gd element in urine of NaGdF4 ND@HAs treated mouse increased significantly from 0 to 12 h post-injection. About 75% Gd was found in urine after 24 h administration, confirming that NaGdF4 ND@HAs were efficiently excreted with the urine. In addition, the NaGdF4 ND@HAs showed a negligible morphology change after in vivo circulation, which indicated that the NaGdF4 ND@HAs have good colloidal stability in vivo (as shown in Fig. S6†). The efficient renal clearance of NaGdF4 ND@HAs helped to eliminate potential hazards of long-term in vivo toxicity.
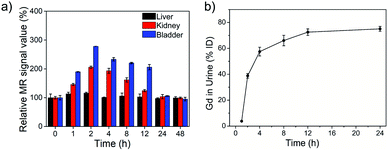 |
| Fig. 5 (a) The MR signal intensities of liver, kidney and bladder of healthy Balb/c mice after intravenous injection of NaGdF4 ND@HAs at different time intervals (0 (pre-injection), 1, 2, 4, 8, 12, 24 and 48 h) of post-injection, respectively. (b) The total amounts of NaGdF4 ND@HAs in mouse urine as a function of post-injection times. Error bars mean standard deviations (n = 5). | |
For further evaluating the biocompatibility, the healthy Balb/c mice were intravenously injected at a single dose of NaGdF4 ND@HAs (10 mg Gd kg−1 body weight in 0.9 wt% NaCl). The bodyweights of NaGdF4 ND@HAs treated mice increased steadily as the time prolonged, which was consistent with those of control group (as shown in Fig. S7†). The result suggested that NaGdF4 ND@HAs have little effect on the growth and development of mice. The main organs (heart, liver, spleen, lung and kidneys) were collected for histology analysis at the 1st day and the 30th day post-injection. Comparing with the control group, the main organs of NaGdF4 ND@HAs treated mice showed negligible lesions or abnormalities (as shown in Fig. 6). Tumor tissue from the mice were collected for H&E staining and anti-CD44v6 staining, respectively. The experimental result indicated that cell membrane surface receptor CD44 was overexpressed on the tumor tissue (as shown in Fig. S8†). Hematology analysis was carried out for monitoring acute and long-term toxicity of NaGdF4 ND@HAs at the 1st day and the 30th day post-injection, respectively. There was little difference between NaGdF4 ND@HAs treated group and control group (as shown in Table S1†). These results demonstrated that NaGdF4 ND@HAs have good biocompatibilities.
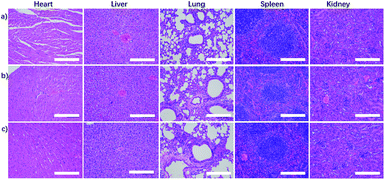 |
| Fig. 6 H&E staining revealed tissue sections from the mice with (a) 0.9% NaCl solution (control) and NaGdF4 ND@HAs (10 mg Gd kg−1 body) at (b) 1 day and (c) 30 days post-injection respectively. The scale bars are 200 μm. | |
Conclusions
In summary, the renal clearable NaGdF4 ND@HAs have been prepared by a two-step reaction through strong interaction of Gd3+ with phosphonate groups in tryptone and amidation reaction between carboxy group of HA and amine group of tryptone. In vitro and in vivo experimental results demonstrate that the as-prepared NaGdF4 ND@HAs display high MDA-MB-231 tumor-targeting capacity. The NaGdF4 ND@HAs have held great potential as an excellent MR contrast agent for detection CD44-overexpression tumor since advantages of NaGdF4 ND@HAs including high tumor targeting ability, efficient renal clearance capacity and excellent biocompatibility satisfied the basic standards of clinical applications.
Conflicts of interest
There are no conflicts to declare.
Acknowledgements
The authors would like to thank the National Natural Science Foundation of China (Grant No. 21775145) for financial support.
References
- D. Peer, J. M. Karp, S. Hong, O. C. Farokhzad, R. Margalit and R. Langer, Nat. Nanotechnol., 2007, 2, 751–760 CrossRef CAS PubMed.
- E. C. Dreaden, A. M. Alkilany, X. Huang, C. J. Murphy and M. A. El-Sayed, Chem. Soc. Rev., 2012, 41, 2740–2779 RSC.
- G. Chen, I. Roy, C. Yang and P. N. Prasad, Chem. Rev., 2016, 116, 2826–2885 CrossRef PubMed.
- D. Bobo, K. J. Robinson, J. Islam, K. J. Thurecht and S. R. CCorrie, Pharm. Res., 2016, 33, 2373–2387 CrossRef PubMed.
- X. Huang, Y. Liu, B. Yung, Y. Xiong and X. Chen, ACS Nano, 2017, 11, 5238–5292 CrossRef.
- Z. Liu, W. Jiang, J. Nam, J. J. Moon and B. Y. S. Kim, Nano Lett., 2018, 18, 6655–6659 CrossRef PubMed.
- C. M. Hartshorn, M. S. Bradbury, G. M. Lanza, A. E. Nel, J. Rao, A. Z. Wang, U. B. Wiesner, L. Yang and P. Grodzinski, ACS Nano, 2018, 12, 24–43 CrossRef PubMed.
- M. Overchuk and G. Zheng, Biomaterials, 2018, 156, 217–237 CrossRef PubMed.
- J. Gupta, M. T. Fatima, Z. Islam, R. H. Khan, V. N. Uversky and P. Salahuddin, Int. J. Biol. Macromol., 2019, 130, 515–526 CrossRef PubMed.
- Y. Liu, P. Bhattarai, Z. Dai and X. Chen, Chem. Soc. Rev., 2019, 48, 2053–2108 RSC.
- E. K. Lim, T. Kim, S. Paik, S. Haam, Y. M. Huh and K. Lee, Chem. Rev., 2015, 115, 327–394 CrossRef.
- J. I. Hare, T. Lammers, M. B. Ashford, S. Puri, G. Storm and S. T. Barry, Adv. Drug Delivery Rev., 2017, 108, 25–38 CrossRef.
- M. M. Alvarez, J. Aizenberg, M. Analoui, A. M. Andrews, G. Bisker, E. S. Boyden, R. D. Kamm, J. M. Karp, D. J. Mooney, R. Oklu, D. Peer, M. Stolzoff, M. S. Strano, G. Trujillo-de Santiago, T. J. Webster, P. S. Weiss and A. Khademhosseini, ACS Nano, 2017, 11, 5195–5214 CrossRef PubMed.
- J. Shi, P. W. Kantoff, R. Wooster and O. C. Farokhzad, Nat. Rev. Cancer, 2017, 17, 20–37 CrossRef.
- J. Paradise, AMA J. Ethics, 2019, 21(4), E347–E355 CrossRef.
- R. A. Petros and J. M. DeSimone, Nat. Rev. Drug Discovery, 2010, 9, 615–627 CrossRef.
- P. Cai, X. Zhang, M. Wang, Y. L. Wu and X. Chen, ACS Nano, 2018, 12, 5078–5084 CrossRef CAS.
- S. Sharifi, S. Behzadi, S. Laurent, M. Laird Forrest and P. Stroeve, Chem. Soc. Rev., 2012, 41, 2323–2343 RSC.
- X. Wang, L. Guo, S. Zhang, Y. Chen, Y. T. Chen, B. Zheng, J. Sun, Y. Qian, Y. Chen, B. Yan and W. Lu, ACS Nano, 2019, 13, 5720–5730 CrossRef.
- E. B. Ehlerding, F. Chen and W. Cai, Adv. Sci., 2016, 3, 1500223 CrossRef PubMed.
- L. Gong, Y. Wang and J. Liu, Biomater. Sci., 2017, 5, 1393–1406 RSC.
- B. Du, M. Yu and J. Zheng, Nat. Rev. Mater., 2018, 3, 358–374 CrossRef.
- T. Cao, Y. Yang, Y. Sun, Y. Wu, Y. Gao, W. Feng and F. Li, Biomaterials, 2013, 34, 7127–7134 CrossRef.
- F. Liu, X. He, H. Chen, J. Zhang, H. Zhang and Z. Wang, Nat. Commun., 2015, 6, 8003 CrossRef.
- M. Yu, J. Liu, X. Ning and J. Zheng, Angew. Chem., Int. Ed., 2015, 54, 15654–15658 CrossRef.
- D. Ni, D. Jiang, H. F. Valdovinos, E. B. Ehlerding, B. Yu, T. E. Barnhart, P. Huang and W. Cai, Nano Lett., 2017, 17, 3282–3289 CrossRef.
- Y. Cui, J. Yang, Q. Zhou, P. Liang, Y. Wang, X. Gao and Y. Wang, ACS Appl. Mater. Interfaces, 2017, 9, 5900–5906 CrossRef.
- Q. Wei, Y. Chen, X. Ma, J. Ji, Y. Qiao, B. Zhou, F. Ma, D. Ling, H. Zhang, M. Tian Mei, J. Tian and Z. Min, Adv. Funct. Mater., 2018, 28, 1704634 CrossRef.
- Y. Wang, S. Ma, Z. Dai, Z. Rong and J. Liu, Nanoscale, 2019, 11, 16336–16341 RSC.
- C. Peng, M. Yu, J. T. Hsieh, P. Kapur and J. Zheng, Angew. Chem., 2019, 58, 12076–12080 CrossRef.
- M. Yu, J. Zhou, B. Du, X. Ning, C. Authement, L. Gandee, P. Kapur, J.-T. Hsieh and J. Zheng, Angew. Chem., Int. Ed., 2016, 55, 2787–2791 CrossRef.
- J. Liu, M. Yu, C. Zhou, S. Yang, X. Ning and J. Zheng, J. Am. Chem. Soc., 2013, 135, 4978–4981 CrossRef.
- C. Peng, X. Gao, J. Xu, B. Du, X. Ning, S. Tang, R. M. Bachoo, M. Yu, W. P. Ge and J. Zheng, Nano Res., 2017, 10, 1366–1376 CrossRef.
- M. Bottrill, L. Kwok and N. J. Long, Chem. Soc. Rev., 2006, 35, 557–571 RSC.
- H. B. Na, I. C. Song and T. Hyeon, Adv. Mater., 2009, 21, 2133–2148 CrossRef.
- L. Zhang, R. Liu, H. Peng, P. Li, Z. Xu and A. K. Whittaker, Nanoscale, 2016, 8, 10491–10510 RSC.
- Y. Cao, L. Xu, Y. Kuang, D. Xiong and R. Pei, J. Mater. Chem. B, 2017, 5, 3431–3461 RSC.
- L. Zeng, D. Wu, R. Zou, T. Chen, J. Zhang and A. Wu, Curr. Med. Chem., 2018, 25, 2970–2986 CrossRef.
- J. Y. Park, M. J. Baek, E. S. Choi, S. Woo, J. H. Kim, T. J. Kim, J. C. Jung, K. S. Chae, Y. Chang and G. H. Lee, ACS Nano, 2009, 3, 3663–3669 CrossRef.
- N. J. J. Johnson, W. Oakden, G. J. Stanisz, R. S. Prosser and F. C. J. M. van Veggel, Chem. Mater., 2011, 23, 3714–3722 CrossRef.
- G. Liang, L. Cao, H. Chen, Z. Zhang, S. Zhang, S. Yu, X. Shen and J. Kong, J. Mater. Chem. B, 2013, 1, 629–638 RSC.
- J. Fang, P. Chandrasekharan, X. L. Liu, Y. Yang, Y. B. Lv, C. T. Yang and J. Ding, Biomaterials, 2014, 35, 1636–1642 CrossRef.
- F. Liu, X. He, J. Zhang, H. Zhang and Z. Wang, Small, 2015, 11, 3676–3685 CrossRef.
- X. Jin, F. Fang, J. Liu, C. Jiang, X. Han, Z. Song, J. Chen, G. Sun, H. Lei and L. Lu, Nanoscale, 2015, 7, 15680–15688 RSC.
- K. Ni, Z. Zhao, Z. Zhang, Z. Zhou, L. Yang, L. Wang, H. Ai and J. Gao, Nanoscale, 2016, 8, 3768–3774 RSC.
- X. Zheng, K. Zhao, J. Tang, X. Wang, L. Li, N. Chen, Y. Wang, S. Shi, X. Zhang and S. Malaisamy, ACS Nano, 2017, 11, 3642–3650 CrossRef.
- H. Chen, X. Li, F. Liu, H. Zhang and Z. Wang, Mol. Pharmaceutics, 2017, 14, 3134–3141 CrossRef.
- B. P. Toole, Nat. Rev. Cancer, 2004, 4, 528–539 CrossRef.
- C. Underhill, J. Cell Sci., 1992, 103, 293–298 Search PubMed.
- J. Lesley, R. Hyman, N. English, J. B. Catterall and G. A. Turne, Glycoconjugate J., 1997, 14, 611–622 CrossRef PubMed.
- C. Guo, L. Sun, H. Cai, Z. Duan, S. Zhang, Q. Gong, K. Luo and Z. Gu, ACS Appl. Mater. Interfaces, 2017, 9, 23508–23519 CrossRef PubMed.
- G. Huang and H. Huang, J. Controlled Release, 2018, 278, 122–126 CrossRef PubMed.
- N. N. Parayath, A. Parikh and M. M. Amiji, Nano Lett., 2018, 18, 3571–3579 CrossRef CAS PubMed.
- Y. Cheng, X. Tan, J. Wang, Y. Wang, Y. Song, Q. You, Q. Sun, L. Liu, S. Wang, F. Tan, J. Li and N. Li, J. Controlled Release, 2018, 277, 77–88 CrossRef CAS PubMed.
- Y. Cao, M. L. Mei, J. Xu, E. C. M. Lo, Q. Li and C. H. Chu, J. Dent., 2013, 41, 818–825 CrossRef CAS.
- C. Holt, N. M. Wahlgren and T. Drakenberg, Biochem. J., 1996, 314, 1035–1039 CrossRef CAS.
- R. Gilli, M. KacurBkova, M. Mathlouthi, L. Navarini and S. Paoletti, Carbohydr. Res., 1994, 263, 315–326 CrossRef CAS.
- Y. Fan, Q. Wang, G. Lin, Y. Shi, Z. Gu and T. Ding, Acta Biomater., 2017, 62, 257–272 CrossRef CAS.
Footnote |
† Electronic supplementary information (ESI) available. See DOI: 10.1039/c9ra08974h |
|
This journal is © The Royal Society of Chemistry 2020 |
Click here to see how this site uses Cookies. View our privacy policy here.