DOI:
10.1039/C9RA09860G
(Paper)
RSC Adv., 2020,
10, 2581-2588
Self-assembled CeVO4/Au heterojunction nanocrystals for photothermal/photoacoustic bimodal imaging-guided phototherapy†
Received
25th November 2019
, Accepted 4th January 2020
First published on
14th January 2020
Abstract
Phototherapy, including photothermal therapy (PTT) and photodynamic therapy (PDT), has attracted great attention because it can effectively inhibit the proliferation and propagation of cancer cells. Recently, heterojunction nanomaterials have shown tremendous application value in the field of biological medicine. In this work, the CeVO4/Au heterojunction nanocrystals (NCs) are designed for photothermal/photoacoustic bimodal imaging-guided phototherapy. The as-synthesized hydrophobic oleic acid (OA)-stabilized CeVO4 nanosheets were modified with HS-PEG-OH for translating into hydrophilic ones, which can significantly improve their stability and biocompatibility. Subsequently, the plasmonic Au nanoparticles were in situ successfully deposited on the surface of HS-PEG-coated CeVO4 to form CeVO4/Au heterojunction NCs for improving the visible and near-infrared light absorption, which results in enhanced photothermal conversion performance and reactive oxygen species (ROS) generation capacity. Thus, the CeVO4/Au can cause more severe damage to cancer cells than pure CeVO4 under NIR laser irradiation. Also, CeVO4/Au can provide distinct tumor contrast by photothermal/photoacoustic bimodal bioimaging. Our results demonstrate that CeVO4/Au NCs could be used as an effective theranostic anticancer agent for near-infrared (NIR) light-mediated PTT and PDT.
Introduction
Cancer has a very serious impact on human health, which causes high mortality worldwide.1–5 Although surgery,6 chemotherapy7–9 and radiotherapy10,11 are considered to be the main treatment approaches for cancer, they have many disadvantages, such as surgical risks and complications, serious side effects and the metastasis of residual cancer cells. Phototherapy, including photothermal and photodynamic therapy, was identified as an emerging noninvasive treatment method and has become a powerful candidate to replace traditional therapeutic methods because it can effectively inhibit the proliferation and propagation of cancer cells.12–14 Photothermal therapy (PTT) can effectively kill target cancer cells by utilizing NIR laser irradiation to produce heat energy from light energy.15–17 In recent years, a series of photothermal agents, including gold nanoparticles (NPs),18–20 carbon-based nanosheets,21 metal chalcogenides,22–25 and conjugated polymers26,27 have been used in PTT. Photodynamic therapy (PDT) can transfer the absorbed light to produce toxic reactive oxygen species (ROS) under NIR laser irradiation by exciting the photosensitizer molecules, in particular oxidative species (·O2−) can cause significant cancer cell apoptosis and necrosis.13,28,29 Recently, the semiconductor NPs (such as TiO2,30 ZnO,31 Fe3O4@SiO2–CdTe32), metal phthalocyanines,33 and chlorin derivatives34 have been used as photosensitizers for PDT by exciting the electron–hole pairs separation. In addition, the imaging technology such as photothermal (PT) imaging and photoacoustic (PA) imaging has been introduced for accurate diagnosis and treatment of cancer. The PT imaging can directly show the therapeutic effect of PTT through monitoring macroscopic temperature change. And the (PA) imaging provides a promising biomedical imaging modality, which provides higher spatial resolution and relatively deeper tissue penetration through the detection of ultrasonic signal waves.35–37
Inorganic semiconductor nanomaterials have been widely used as photothermal agents,38 photosensitizes39 and multimode imaging agents40–42 due to their unique optical performance. Cerium vanadate (CeVO4), a kind of wide band gap inorganic semiconductor nanomaterials,43 has relatively small optical cross-sections in the Vis/NIR regions.44 Thus, it was rarely applied as NIR-responsive anticancer agents.45 The local surface plasmon resonance (LSPR)46 offers a new strategy to enhance the optical absorption of semiconductor nanomaterials through loading plasmonic metal nanoparticles (NPs) on the surface of semiconductor NCs.47–51 Integrating plasmonic Au NPs with various semiconductors nanomaterials to form heterojunction nanostructures, such as Au@SiO2@Cu2O,52 Bi2S3@Au,53 Au@CuS,54 CuS@Cu2S@Au,55 Au/TiO2,56 Au@PB@Cu2O@ BPQDs/PAH,57 Au@NdVO4,49 Au/Fe3O4,58 could significantly expand the light absorption range to improve the photo-conversion performance.
Herein, we have successfully obtained CeVO4/Au heterojunction NCs in this study (Scheme 1). Firstly, the oleic acid (OA)-stabilized CeVO4 nanosheets were prepared through hydrothermal method.59 Next, the (OA)-stabilized CeVO4 was modified by HS-PEG-OH through thiolene click reaction. These modified materials can improved biocompatibility and stability.32,60–64 Finally, Au NPs were in situ successfully deposited on the surface of HS-PEG-coated CeVO4 to form CeVO4/Au heterojunction NCs. Once CeVO4/Au was transferred into water from THF, they presented self-assembled morphology due to hydrophobic–hydrophobic interactions because the OA-coated CeVO4 didn't completely modified by HS-PEG-OH. Due to the LSPR effect of Au, the CeVO4/Au NCs represents enhanced separation efficiency of electron and hole pairs and increased optical absorption in Vis/NIR region, resulting in improved ROS generation capability and photothermal conversion performance. Therefore, the CeVO4/Au could cause severe damage to cancer cells than CeVO4 under NIR laser irradiation. Also, the CeVO4/Au can provide distinct tumor contrast by photothermal/photoacoustic bimodal bioimaging. Our results demonstrate that CeVO4/Au NCs could be used as an effective theranostic anticancer agent for near-infrared (NIR) light-mediated PTT and PDT.
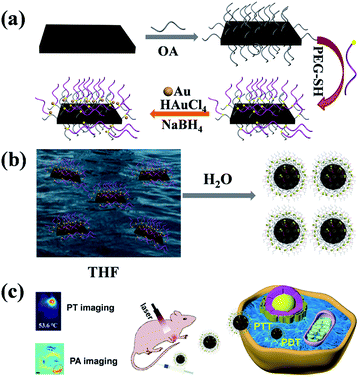 |
| Scheme 1 Schematic illustration of the synthesis process (a), self-assembly process (b) and biological application (c) of CeVO4/Au. | |
Experimental section
Synthesis of oleic acid-capped CeVO4 nanosheets
NaOH (0.6 g) and NH4VO3 (0.0585 g) were added into 5 mL of deionized water under magnetic stir. A mixed solution of oleic acid (9 mL) and ethanol (10 mL) was added into the above solution. Then, 1 mL of Ce(NO3)3·6H2O aqueous solution (1 M) was added dropwise. After stirring for 10 min, the mixed solution was sealed in a 40 mL Teflon-lined autoclave (140 °C, 8 h), then slowly cooled to room temperature, the samples were collected and dissolved in cyclohexane. Finally, CeVO4 nanosheets were isolated by centrifugation at 3000 rpm (5 min), and washed with cyclohexane and ethanol. Afterward, the CeVO4 nanosheets were distributed in 5 mL cyclohexane.
Synthesis of HS-PEG-functionalized CeVO4 nanosheets
The 2 mL of above CeVO4 nanosheets were precipitated by adding enough ethanol and isolated by centrifugation at 3000 rpm for 5 min. Then the samples were dissolved into 2 mL of THF solution. Then 0.2 g of HS-PEG-OH and 100 µL of photoinitiator DMPA (10 mg mL−1) dissolved in THF were added. The mixture was irradiated with UV-light (1000 W, 365 nm wavelength) for 60 min in an ice bath under stirring. After completion of UV irradiation, the HS-PEG-functionalized CeVO4 nanosheets were isolated by centrifugation at 12
000 rpm (15 min), and washed with water.
Synthesis of CeVO4/Au heterojunction NCs
The above HS-PEG functionalized CeVO4 nanosheet was distributed in ethanol (1.2 mL). After stirring for 30 min, 100 µL of HAuCl4·3H2O aqueous solution (0.029 M) was added. After stirring for 10 min, 50 µL of NaBH4 (0.06 M) was added and stirred for 30 min. The CeVO4/Au NCs were centrifuged at 12
000 rpm (10 min), and washed with ethanol. Afterward, the products were distributed in deionized water.
Toxicology evaluation
The CeVO4/Au (20 mg kg−1, 100 µL) was tail vein injected into healthy Blab/c mice and the mice injected with normal saline as a control group. For the blood of the experimental group and control group was collected (1 day, 7 days and 14 days post-injection). And then the blood panel and serum biochemical analysis were carried on.
Bio-distribution of CeVO4/Au in mice
The CeVO4/Au (20 mg kg−1, 100 µL) was tail vein injected into U14 tumor-bearing Balb/c mice. Then the mice (N = 4) were euthanized at different time points (1 hour, 4 hours, 8 hours, 12 hours, 1 day, 3 days and 7 days). The major organs (heart, liver, spleen, lung, and kidney) and tumor were collected and weighed. Afterward, these organs were dealt with concentrated nitric acid and H2O2 [V (HNO3)
:
V (H2O2) = 1
:
2] on heating (70 °C) until they became clear. The concentrations of Ce and Au were detected by inductively coupled plasma mass spectrometry (ICP-MS), and were calculated in each organ and tumor.
Live-dead cell staining experiments
HeLa cells were seeded at a density of 0.2 × 105 cells per well in 24-well culture plates and incubated at 37 °C in 5% CO2 for 24 h. Afterward, HeLa cells were incubated with 400 µL of CeVO4 or CeVO4/Au (200 µg mL−1) at 37 °C for 4 hours. Then, the culture media were replaced with fresh medium, and exposed to NIR laser irradiation (1.0 W cm−2) for 5 min. After that, the live cells were stained with Calcein-AM (green fluorescence) and the dead cells were stained with pyridine iodide (PI) (red fluorescence).
Histological analysis
For hematoxylin and eosin (H&E) staining, major organs (heart, liver, spleen, lung, and kidney) and tumor were harvested, fixed in 10% neutral buffered formalin, processed routinely into paraffin, sectioned into thin slices and stained with H&E for histological analysis at day 14.
Photothermal (PT) imaging
For in vitro PT imaging, the CeVO4 solution and CeVO4/Au solution (200 µg mL−1, 100 µL) were exposed to a cuvette and were placed on NIR laser irradiation (1.0 W cm−2) for 5 min. The real-time temperature was detected and recorded by an infrared camera. For in vivo PT imaging, U14 cells were subcutaneously inoculated into the left armpit of Blab/c mice. While the tumor volume changes reached about 100 mm3, normal saline (100 µL), CeVO4 and CeVO4/Au (20 mg kg−1) were tail vein injected, respectively. After 8 h post-injection, the tumor was exposed to NIR laser irradiation (0.5 W cm−2), and PT images were captured by an infrared camera.
Photoacoustic (PA) imaging
To measure the photoacoustic (PA) signal sensitivity of CeVO4 and CeVO4/Au, a phantom filled with the different concentrations of NCs (10, 20, 40, 80 and 160 µg mL−1) was measured using a real-time multispectral optoacoustic tomographic (MSOT) imaging system (inVision 128, iThera Medical GmbH, Neuherberg, Germany). Subsequently, the phantom was placed into a water tank and imaged at the 680–980 nm laser. Finally, the PA signals were measured in regions of interest (ROIs) for each sample and the correlation between the PA signal and concentration response curve was calculated.
To perform in vivo PA imaging, CeVO4 and CeVO4/Au NCs (20 mg kg−1, 100 µL) were tail vein injected into the tumor-bearing mice. After 8 h, the mice were placed in a container surrounded by a polyethylene film. In all data acquisitions, the light fibers and ultrasonic sensor arrays were in a fixed position. The mice were scanned before and after tail vein injection. ROIs were chosen and the PA signal was analyzed using ViewMOST™ software.
Animal xenograft model
Female Balb/c mice (six weeks old) were purchased from the Center for Experimental Animals, Jilin University. All animal studies were conducted in accordance with the guidelines of the National Regulation of China for Care and Use of Laboratory Animals. The U14 tumor models were successfully established by subcutaneous injection of 4 × 106 cells suspended in 100 µL PBS into the left armpit of each mouse. The mice were treated when the tumor volumes approached 100 mm3.
Results and discussion
Preparation and characterization of the CeVO4 and CeVO4/Au
The preparation process of CeVO4/Au was showed in Scheme 1. Firstly, the oleic acid (OA)-stabilized CeVO4 nanosheets were prepared through the hydrothermal method,59 which exhibits square-like sheet morphology as shown in the transmission electron microscopy (TEM) image (Fig. 1A). And the characteristic diffraction peaks of CeVO4 in powder X-ray diffraction (XRD) patterns match well with those of the tetragonal phase (CeVO4, JCPDS 82-1969) (Fig. 1B). Afterward, the (OA)-stabilized CeVO4 surface was modified by the HS-PEG-OH60 for transforming hydrophobic CeVO4 into hydrophilic and enhancing biocompatibility. On the basis of the FT-IR spectroscopy (Fig. 1C), we concluded that the
C–H stretching vibration appears at 3006 cm−1 decreased and the stretching vibration owing to –CH2–O– at 1106 cm−1 is gradually enhanced, which demonstrate that the OA-stabilized CeVO4 was successfully modified by HS-PEG-OH. Finally, Au nanoparticles were in situ deposited on the surface of HS-PEG-coated CeVO4 via the reduction of HAuCl4 by NaBH4. Fig. 1D shows the TEM image of as-prepared CeVO4/Au heterojunction nanocrystals, which presents self-assembled morphology due to hydrophobic–hydrophobic interactions because the OA-coated CeVO4 didn't completely modified by HS-PEG-OH. According to the high-resolution transmission electron microscopy (HR-TEM) image (Fig. 1E), the lattice fringes of CeVO4/Au nanohybrids display interplanar spacings of 0.2355 nm and 0.2949 nm, which match well with those of the (111) and the (211) planes of Au and CeVO4, respectively. As can be seen from the elemental mapping (Fig. 1F), the elements of Ce, V, O, C, S and Au are presented in the CeVO4/Au nanohybrids. And the changed zeta potential from 20.3 to −6.3 mV further verified the formation of CeVO4/Au (Fig. S1†). Moreover, the average hydrodynamic particle sizes of CeVO4/Au is about 127.5 nm, which could maintain constant within seven days in various physiological solutions (Fig. S2†).
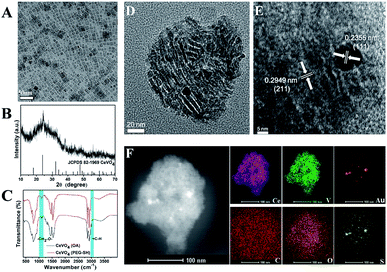 |
| Fig. 1 (A) TEM images of the OA-capped CeVO4 dispersed in hexane; (B) XRD patterns of OA-capped CeVO4; (C) FTIR spectra of OA-capped CeVO4 and CeVO4 modified by HS-PEG-OH; (D) TEM image of CeVO4/Au; (E) HR-TEM image and (F) EDS elemental mapping of CeVO4/Au. | |
Photothermal properties contrast among CeVO4 and CeVO4/Au
The CeVO4/Au displays exceptional optical properties due to LSPR of Au NPs as compared to pure CeVO4. According to the UV-Vis-NIR spectroscopy (Fig. 2A), a slight blue-shift of the Ce3+ characteristic absorption from 285 to 275 nm was observed due to the formation of CeVO4/Au nanohybrids. More importantly, the obviously enhanced absorption of CeVO4/Au could be found in the Vis/NIR region, which stimulated our study of the photothermal properties of CeVO4/Au. The temperature elevation of CeVO4, CeVO4/Au, and water under NIR (1.0 W cm−2) laser irradiation was showed in Fig. 2B. The temperature of CeVO4/Au aqueous solution increased to 54.7 °C, while that of CeVO4 solution and water only increase to 40 °C and 28.9 °C, respectively. In addition, both CeVO4/Au and CeVO4 showed concentration and power density-dependent temperature evolution activity (Fig. S3A–D†). However, the CeVO4/Au solution displayed a more excellent photothermal conversion performance than pure CeVO4 solution. According to the obtained test result (Fig. 2C and S4†), the time constant (τs) for the cooling process of CeVO4/Au and CeVO4 are determined to be 488.63 s and 581.51 s, respectively. Meanwhile, the photothermal conversion efficiency (η) of CeVO4/Au and CeVO4 were calculated to be 17.32% and 11.65%, respectively. Moreover, the photothermal conversion performance of CeVO4/Au is almost no change after three cycles of heating and cooling (heating 6 min and cooling 12 min for one cycle, Fig. 2D). Collectively, these results validate that CeVO4/Au heterojunction NCs are more excellent photothermal conversion agent than pure CeVO4 due to the LSPR of Au NPs.
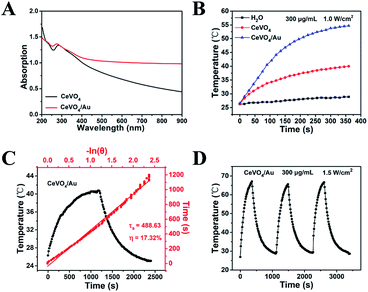 |
| Fig. 2 (A) Vis/NIR absorption spectra of CeVO4 and CeVO4/Au. (B) Photothermal activity of CeVO4 and CeVO4/Au (300 µg mL−1) under 1.0 W cm−2 of NIR laser irradiation for 5 min. (C) The temperature change of CeVO4/Au aqueous solution (300 µg mL−1, 1 mL) response to 1.3 W cm−2 of NIR laser on and off and Linear time data versus −ln θ obtained from the cooling period of CeVO4/Au aqueous solution. (D) The PT conversion cycling test of CeVO4/Au aqueous solution under 1.0 W cm−2 of NIR laser irradiation. | |
The ROS detection
The production capability of reactive oxygen species (ROS) over CeVO4 and CeVO4/Au heterojunction NCs upon NIR laser irradiation was further studied. As a result of LSPR excitation, Au NPs could generate hot electrons through absorbing resonant photons and transmit them to the adjacent CeVO4, which surmounts the wide band gap of CeVO4 and leads to effective separation of electrons–holes pairs in CeVO4/Au heterojunction NCs. In the first place, Au NPs could produce hot electrons upon resonant excitation. These hot electrons could focus on the conduction band (CB) of the CeVO4 by hot-electrons injection process,65,66 and they could be seized by molecular oxygen (O2) to produce ·O2−. The 1,3-diphenylisobenzofuran (DPBF) probe was used to monitor the performance of CeVO4 and CeVO4/Au to generate ·O2− under photo-excitation. On the basis of Fig. 3A and S5,† we concluded that the absorption intensity of DPBF decreased distinctly with the increasing of NIR laser irradiation time in the CeVO4/Au group, while that of the CeVO4 group was almost unchanged. In order to eliminate the interference of oxygen, we detected the fluorescence intensity of singlet oxygen sensor green (SOSG, highly specific fluorescent indicator to 1O2) in the presence of CeVO4 and CeVO4/Au with NIR laser (1.0 W cm−2) irradiation. As displayed in Fig. S6,† we concluded that the fluorescence intensity of SOSG were almost unchanged with the increasing of NIR laser irradiation time in the CeVO4/Au group and the CeVO4 group. From the results we have obtained no singlet oxygen (1O2) was generated. In the second place, the residual holes (hVB+) in the valence band of CeVO4 could oxidize H2O to produce hydroxyl radicals (·OH), which was monitored by the degradation of methylene blue (MB). Comparing with the CeVO4 group, the absorption intensity of MB decreased rapidly in the CeVO4/Au group under NIR laser irradiated for 20 min (Fig. 3B and S7†). From the results we have obtained, the separation efficiency of electron/hole pairs of CeVO4/Au was remarkably improved in comparison with that of CeVO4 due to the LSPR of Au NPs, which leads to affluent ROS generation more effectively (Fig. 3C). In order to evaluate NIR-induced ROS generation in the cellular level, the ROS production was detected by non-fluorescent 2′,7′-dichlorodihydrofluoresceindiacetate (DCFH-DA), which can be oxidized by ROS translated into 2′,7′-dichlorofluorescein (DCF) with green fluorescence. As could be seen from Fig. 3D, no visible fluorescence was monitored in the control, NIR, CeVO4, CeVO4/Au, or CeVO4 plus NIR groups. Nevertheless, the fluorescence of DCF (bright green) was showed in the group of CeVO4/Au plus NIR groups, which further confirmed that the boosted ROS production ability of CeVO4/Au owing to the LSPR of Au NPs.
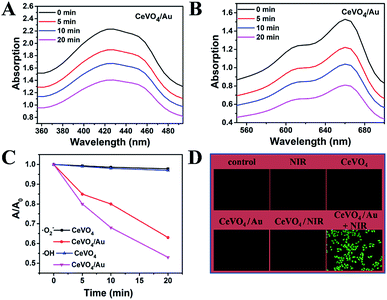 |
| Fig. 3 (A) Depletion of DPBF due to ·O2− generation: 65 µg mL−1 of CeVO4/Au with NIR laser (1.0 W cm−2) irradiation. (B) Depletion of MB due to ·OH generation: 65 µg mL−1 of NdVO4/Au with NIR laser (1.0 W cm−2) irradiation. (C) The comparison of ·OH and ·O2− generating ability over CeVO4 and CeVO4/Au NCs plus NIR laser irradiation. (D) Fluorescence microscope images of ROS generation in HeLa cells that received different treatments. | |
Cellular uptake imaging, cytocompatibility, and cytotoxicity assay
On the basis of the boosted ROS production ability and photothermal performance of CeVO4/Au, the synergistic photothermal and photodynamic anticancer effect in vitro was investigated. Before the in vitro anticancer application, the cellular uptake behavior of the CeVO4/Au heterojunction NCs was verified. As displayed in Fig. 4A, the overlapping fluorescence of DAPI-labeled cell nuclei (blue) and rhodamine B (RhB)-labeled CeVO4/Au (red) was observed, which suggested that the efficient uptake of CeVO4/Au NCs via endocytosis. To further prove the cell uptake of CeVO4/Au NCs was via endocytosis. We explore the intensity of red fluorescence from rhodamine B by different treatment times (HeLa cells treated with CeVO4/Au NCs-RhB at different times, 0 h, 2 h, 4 h and 6 h), which was detected by flow cytometry. As displayed in Fig. S8,† with the increase in time, the red fluorescence intensity of rhodamine B raised, which indicated the increased cell endocytosis and the efficient uptake of CeVO4/Au NCs by cell. The biocompatibility of CeVO4 and CeVO4/Au was monitored on HeLa and L929 cells via standard methyl thiazolyl tetrazolium (MTT) assay. As exhibited in Fig. 4B, both L929 and HeLa cell viability were unaffected even if the concentrations of CeVO4/Au NCs up to 200 µg mL−1, which identified that the CeVO4 and CeVO4/Au have well biocompatibility. Then the NIR-triggered photo-toxicity of CeVO4 and CeVO4/Au were assessed on HeLa cells. As could be seen from Fig. 4C and D, the cells mortality sharply rose to around 85% when HeLa cells were dealt with a low concentration (200 µg mL−1) of CeVO4/Au plus NIR (1.0 W cm−2) irradiation. However, only about 23% of HeLa cells were killed in the CeVO4 group even if the laser power density reached up to 1.5 W cm−2. The outstanding killing capability of CeVO4/Au to cancer cells were further confirmed via live-dead cell staining experiments (Fig. 4E). The above results demonstrated that the improved synergistic photothermal and photodynamic anticancer effect of CeVO4/Au in comparison with single CeVO4 in vitro, which was caused by boosted ROS production ability and photothermal performance of CeVO4/Au.
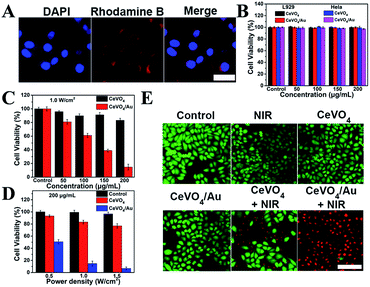 |
| Fig. 4 (A) Cellular uptake of the CeVO4/Au as detected in HeLa cells treated with CeVO4/Au-RhB and DAPI. (B) L929 cell and HeLa cell viability when incubated with CeVO4 and CeVO4/Au for 24 h at different concentrations. (C) HeLa cell viability when incubated with different concentrations of CeVO4 and CeVO4/Au for 24 h under NIR laser irradiation at 1.0 W cm−2 for 5 min. (D) HeLa cell viability when incubated with CeVO4 and CeVO4/Au (200 µg mL−1) for 24 h under NIR laser irradiation with different power densities for 5 min. (E) Live/dead cytotoxicity analysis of HeLa cells after different treatment. (Scale bar 50 µm). | |
PT/PA dual-modal imaging
Owing to the intrinsic absorption of CeVO4 and CeVO4/Au in the NIR region, their potentials as effective photothermal (PT) imaging and photoacoustic (PA) imaging37 agents in vitro and in vivo were investigated. Firstly, the PT imaging capability in vitro was studied by irradiating deionized water, CeVO4, and CeVO4/Au solution with NIR laser irradiation (1.0 W cm−2) for 5 min. Simultaneously, the real-time temperature changes were recorded prep 1 min by an infrared thermal camera. As displayed in Fig. 5A, the temperature of CeVO4/Au and CeVO4 could raise by about 21.6 °C and 10.7 °C, respectively. However, the temperature of the deionized water could only be enhanced by 2.3 °C. Whereafter, for in vivo PT imaging, tumor-bearing mice were tail vein injected with PBS, CeVO4, and CeVO4/Au (20 mg kg−1) solution and were exposed to NIR laser irradiation (0.5 W cm−2). All animal procedures were performed in accordance with the Guidelines for the Care and Use of Laboratory Animals of Jilin University (Changchun, China) and were approved by the Animal Ethics Committee of the National Regulation of China for the Care and Use of Laboratory Animals. And the real-time thermal images were detected by an infrared thermal camera. As could be seen from Fig. 5B, the tumor temperature in the mice injected with the CeVO4/Au increased by about 16.7 °C, which is obviously higher than that of CeVO4-injected mice (8.7 °C). While the tumor temperature of control group mice injected with PBS didn't show an evident increase. From the results, we concluded that the CeVO4/Au has more excellent photothermal imaging ability than single CeVO4. Then, the potential of CeVO4 and CeVO4/Au as a PA imaging agent in vitro and in vivo was evaluated. As far as is concerned in vitro PA imaging, the different concentrations of CeVO4 and CeVO4/Au were embedded in agar gel cylinders to generate PA imaging. As exhibited in Fig. 5C, PA signal intensity of CeVO4/Au exhibited remarkably increased with elevated concentration from 10 to 160 µg mL−1 in comparison with single CeVO4. And the quantitative analysis illustrated a linear relationship between the PA signal intensity and samples (CeVO4 and CeVO4/Au) concentrations from 10 to 160 µg mL−1 (Fig. S9†). To investigate in vivo PA imaging effect, tumor-bearing mice tail vein injected with PBS, CeVO4, and CeVO4/Au (20 mg kg−1) solution were detected, respectively. As displayed in Fig. 5D, the tumor site of the CeVO4/Au-injected group exhibited clearly stronger PA signals than a single CeVO4 injection group, while almost no PA signals in the tumor site of the PBS injection group could be detected. From the above results we have obtained, one can conclude that CeVO4/Au NCs could be used as an appropriate anticancer agent for PT and PA bimodal imaging in early cancer diagnosis and treatment for further clinical application.
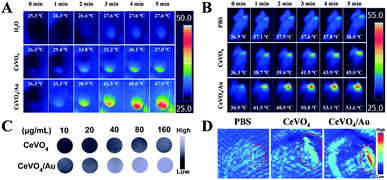 |
| Fig. 5 (A) The thermal images of H2O, CeVO4 and CeVO4/Au (200 µg mL−1, 100 µL) exposed to NIR laser irradiation (1.0 W cm−2) for different times (0, 1, 2, 3, 4 and 5 min). (B) The thermal images of U14-tumor-bearing mice with tail veins injection of normal saline, CeVO4 and CeVO4/Au (20 mg kg−1, 100 µL) for 12 h exposed to NIR laser light irradiation (0.5 W cm−2) for different times (0, 1, 2, 3, 4 and 5 min). (C) PA imaging phantoms, consisting of various concentrations of CeVO4 and CeVO4/Au embedded in agar gel cylinders. (D) PA images of tumor site after tail veins injection with normal saline, CeVO4 and CeVO4/Au (20 mg kg−1, 100 µL) for 8 h. (The red circle is tumor sites.) | |
In vivo long-term toxicity evaluation and tumor inhibition effect
To further explore the cancer therapeutic effects of CeVO4/Au NCs in vivo, we assess the long-term toxicity of the CeVO4/Au NCs firstly. The results were obtained by detecting bio-distribution, blood panel, and serum biochemical index. These tests were monitored by injecting CeVO4/Au NCs into mice via tail vein. The tumor and main organs (heart, liver, spleen, lung, kidney) were resected from the mice at different time periods (1 hour, 4 hours, 8 hours, 12 hours, 1 day, 3 days and 7 days) of post-injection, and the contents of the Ce and Au were detected via ICP-MS. As can be seen from Fig. 6, Ce and Au were dispersed in every main organs and tumor, while we could observe that the liver and spleen have high concentrations of Ce (Fig. 6A) and Au (Fig. 6B) accumulation after 8 h post-injection due to the cancellation of exotic substances by the reticuloendothelial system and macrophage at early stages. With the passage of time, the concentrations of Au and Ce in the main organs gradually declined, and the mass of the CeVO4/Au NCs were overtly eliminated from the spleen and liver at 7 d post-injection. From the bio-distribution experiment results, we can conclude that the CeVO4/Au NCs could be accumulated in the tumor site owing to the enhanced permeability and retention (EPR) effect, and the most effective treatment time is about 8 h post-injection. To achieve a better understanding of the long-term toxicity of the CeVO4/Au NCs in vivo, the blood panel, and serum biochemical index were also investigated. As shown in Table S1,† we can demonstrate that all the indexes were in good consistent with the normal range and very approaching to the control group, suggesting that no hepatic or renal necrocytosis and inflammatory response could be found. Based on the above results, the CeVO4/Au NCs had no apparent toxicity effects on healthy mice, which could be used as potential cancer therapeutic and diagnostic agents in vivo.
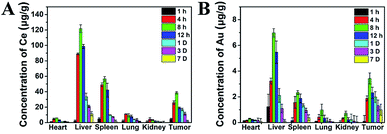 |
| Fig. 6 Bio-distribution of Ce (A) and Au (B) in major organs and tumors of mice after injection of CeVO4/Au (20 mg kg−1, 100 µL) at different time points. | |
Based on the distinguished photothermal conversion property, ROS production capability, and no obvious poison effects of CeVO4/Au NCs, we future investigated tumor inhibition effect of CeVO4/Au NCs in vivo. The U14 tumor-bearing mice were randomly divided into six groups: (a) control group (normal saline injection), (b) NIR, (c) CeVO4 injection, (d) CeVO4/Au injection, (e) CeVO4 injection + NIR and (f) CeVO4/Au injection + NIR. The laser irradiation was implemented under NIR laser irradiation (0.5 W cm−2) for 5 min. The tumor volume changes were recorded every 2 d after treatment (14 d) (Fig. 7B). We could found that the tumor in these groups of control group, NIR, CeVO4, and CeVO4/Au exhibited rapid growth trend during the 14 days treatment. Nevertheless, the tumor growth was suppressed slightly in the group of CeVO4 + NIR laser irradiation. It is noteworthy that no tumor growth was observed in CeVO4/Au + NIR laser irradiation during the 14 days treatment. The results could be further verified by the photos and tumors resection of each photographs of mice and tumors after different treatment (Fig. 7A). As shown in Fig. 7C, the tumor sections were stained with hematoxylin and eosin (H&E), which prove that tumor tissues were injured severely via CeVO4/Au + NIR laser irradiation and correspondingly slight damage induced via CeVO4 + NIR laser irradiation could also be observed. From the results we have obtained, the cancer therapy effect of CeVO4/Au is very prominent than CeVO4. Moreover, the body weights of these mice maintained growth steadily within 14 days in the different treatment groups (Fig. 7D). The main organs of mice after different treatments showed no obvious inflammatory lesion through histology analysis (Fig. 8). Taken together, CeVO4/Au could be served as a PT/PA dual-modal imaging-guided anticancer agent, which possesses an enhanced antitumor effect than pure CeVO4.
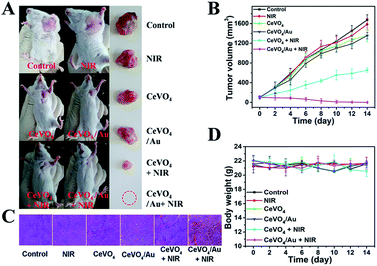 |
| Fig. 7 (A) Photos of representative mice and excised tumor tissues from different groups at the end of treatment. (B) Relative tumor volumes changes, (C) images of H&E stained tumor sections and (D) body weight changes of different groups with different treatments processes (n = 4). | |
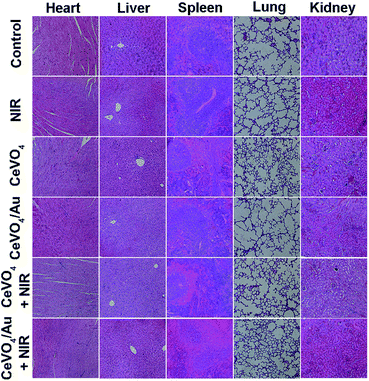 |
| Fig. 8 The H&E stained images of major organs after the treatment with normal saline as control; NIR laser irradiation only; CeVO4 only; CeVO4/Au only; CeVO4 + NIR laser irradiation; CeVO4/Au + NIR laser irradiation (0.5 W cm−2, 5 min). | |
Conclusions
In conclusion, the CeVO4/Au heterojunction nanocrystals (NCs) were successfully fabricated in this study. The CeVO4/Au NCs showed enhanced Vis/NIR light absorption and separation efficiency of electron and hole pairs because of the LSPR effect of Au NPs, which resulted in enhanced ROS generation capability and photothermal conversion ability than pure CeVO4. The CeVO4/Au could cause severe damage to cancer cells than CeVO4 under NIR laser irradiation. Noteworthy, the CeVO4/Au can provide distinct tumor contrast by photothermal/photoacoustic bimodal bioimaging. Also the CeVO4/Au could act as NIR light-mediated photothermal therapy (PTT) and photodynamic therapy (PDT) anticancer agent, which presented enhanced tumor inhibition rate than pure CeVO4. Taken together, our results demonstrate that CeVO4/Au NCs could be used as an effective PT/PA bimodal imaging-guided theranostic anticancer agent for near-infrared (NIR) light-mediated PTT and PDT.
Conflicts of interest
There are no conflicts to declare.
Acknowledgements
This work was financially supported by the projects from Science and Technology department of Jilin province (no. 20190201003 JC; 3D518NU13430).
Notes and references
- C. Zhang, W. H. Chen, L. H. Liu, W. X. Qiu, W. Y. Yu and X. Z. Zhang, Adv. Funct. Mater., 2017, 27, 1700626–1700639 CrossRef.
- W. Fan, B. C. Yung and X. Chen, Angew. Chem., Int. Ed., 2018, 57, 8383–8394 CrossRef CAS.
- J. Xu, F. He, Z. Cheng, R. Lv, Y. Dai, A. Gulzar, B. Liu, H. Bi, D. Yang and S. Gai, Chem. Mater., 2017, 29, 7615–7628 CrossRef CAS.
- G. Yang, L. Xu, Y. Chao, J. Xu, X. Sun, Y. Wu, R. Peng and Z. Liu, Nat. Commun., 2017, 8, 902–914 CrossRef.
- X. Zhu, J. Li, X. Qiu, Y. Liu, W. Feng and F. Li, Nat. Commun., 2018, 9, 2176–2186 CrossRef.
- O. A. Martin, R. L. Anderson, K. Narayan and M. P. MacManus, Nat. Rev. Clin. Oncol., 2017, 14, 32–44 CrossRef CAS PubMed.
- L. Liao, J. Liu, E. C. Dreaden, S. W. Morton, K. E. Shopsowitz, P. T. Hammond and J. A. Johnson, J. Am. Chem. Soc., 2014, 136, 5896–5899 CrossRef CAS.
- Y. Wen, W. Zhang, N. Gong, Y.-F. Wang, H.-B. Guo, W. Guo, P. C. Wang and X.-J. Liang, Nanoscale, 2017, 9, 14347–14356 RSC.
- N. Shah, A. S. Mohammad, P. Saralkar, S. A. Sprowls, S. D. Vickers, D. John, R. M. Tallman, B. P. Lucke-Wold, K. E. Jarrell and M. Pinti, Pharmacol. Res., 2018, 132, 47–68 CrossRef CAS.
- Y.-S. Yang, R. P. Carney, F. Stellacci and D. J. Irvine, ACS Nano, 2014, 8, 8992–9002 CrossRef CAS.
- K. Lu, C. He, N. Guo, C. Chan, K. Ni, G. Lan, H. Tang, C. Pelizzari, Y.-X. Fu and M. T. Spiotto, Nat. Biomed. Eng., 2018, 2, 600–610 CrossRef CAS PubMed.
- C. Liang, L. Xu, G. Song and Z. Liu, Chem. Soc. Rev., 2016, 45, 6250–6269 RSC.
- W. Fan, P. Huang and X. Chen, Chem. Soc. Rev., 2016, 45, 6488–6519 RSC.
- Z. Wan, H. Mao, M. Guo, Y. Li, A. Zhu, H. Yang, H. He, J. Shen, L. Zhou and Z. Jiang, Theranostics, 2014, 4, 399–411 CrossRef PubMed.
- D. Jaque, L. M. Maestro, B. Del Rosal, P. Haro-Gonzalez, A. Benayas, J. Plaza, E. M. Rodriguez and J. G. Sole, nanoscale, 2014, 6, 9494–9530 RSC.
- Y. Liu, P. Bhattarai, Z. Dai and X. Chen, Chem. Soc. Rev., 2019, 48, 2053–2108 RSC.
- T. Ramasamy, H. B. Ruttala, P. Sundaramoorthy, B. K. Poudel, Y. S. Youn, S. K. Ku, H.-G. Choi, C. S. Yong and J. O. Kim, NPG Asia Mater., 2018, 10, 197–216 CrossRef CAS.
- X. Yang, M. Yang, B. Pang, M. Vara and Y. Xia, Chem. Rev., 2015, 115, 10410–10488 CrossRef CAS PubMed.
- J. Nam, N. Won, H. Jin, H. Chung and S. Kim, J. Am. Chem. Soc., 2009, 131, 13639–13645 CrossRef CAS.
- Y. Yang, M. Chen, Y. Wu, P. Wang, Y. Zhao, W. Zhu, Z. Song and X.-B. Zhang, RSC Adv., 2019, 9, 28541 RSC.
- X. Zhu, W. Feng, J. Chang, Y.-W. Tan, J. Li, M. Chen, Y. Sun and F. Li, Nat. Commun., 2016, 7, 10437–10446 CrossRef CAS PubMed.
- M. Chang, M. Wang, M. Wang, M. Shu, B. Ding, C. Li, M. Pang, S. Cui, Z. Hou and J. Lin, Adv. Mater., 2019, 1905271–1905280 CrossRef CAS PubMed.
- B. Ding, C. Yu, C. Li, X. Deng, J. Ding, Z. Cheng, B. Xing and J. Lin, Nanoscale, 2017, 9, 16937–16949 RSC.
- Y. Wang, D. Cai, H. Wu, Y. Fu, Y. Cao, Y. Zhang, D. Wu, Q. Tian and S. Yang, Nanoscale, 2018, 10, 4452–4462 RSC.
- B. Zhou, J. Zhao, Y. Qiao, Q. Wei, J. He, W. Li, D. Zhong, F. Ma, Y. Li and M. Zhou, Appl. Mater. Today, 2018, 13, 285–297 CrossRef.
- C. Hu, Z. Zhang, S. Liu, X. Liu and M. Pang, ACS Appl. Mater. Interfaces, 2019, 11, 23072–23082 CrossRef CAS PubMed.
- Y. Shi, S. Liu, Y. Liu, C. Sun, M. Chang, X. Zhao, C. Hu and M. Pang, ACS Appl. Mater. Interfaces, 2019, 11, 12321–12326 CrossRef CAS PubMed.
- C. Pais-Silva, D. de Melo-Diogo and I. Correia, Eur. J. Pharm. Biopharm., 2017, 113, 108–117 CrossRef CAS PubMed.
- X. Hu, H. Tian, W. Jiang, A. Song, Z. Li and Y. Luan, Small, 2018, 14, 1802994–1803004 CrossRef PubMed.
- D. Yang, G. Yang, Q. Sun, S. Gai, F. He, Y. Dai, C. Zhong and P. Yang, Adv. Healthc. Mater., 2018, 7, 1800042–1800052 CrossRef PubMed.
- P. Sivakumar, M. Lee, Y.-S. Kim and M. S. Shim, J. Mater. Chem. B, 2018, 6, 4852–4871 RSC.
- Z. Wang, X. Jiang, W. Liu, G. Lu and X. Huang, Sci. China: Chem., 2019, 62, 889–896 CrossRef CAS.
- C. Yue, Y. Yang, C. Zhang, G. Alfranca, S. Cheng, L. Ma, Y. Liu, X. Zhi, J. Ni and W. Jiang, Theranostics, 2016, 6, 2352–2366 CrossRef CAS PubMed.
- L. Zeng, Y. Pan, R. Zou, J. Zhang, Y. Tian, Z. Teng, S. Wang, W. Ren, X. Xiao and J. Zhang, Biomaterials, 2016, 103, 116–127 CrossRef CAS PubMed.
- J. R. Cook, W. Frey and S. Emelianov, ACS Nano, 2013, 7, 1272–1280 CrossRef CAS PubMed.
- F. Mao, L. Wen, C. Sun, S. Zhang, G. Wang, J. Zeng, Y. Wang, J. Ma, M. Gao and Z. Li, ACS Nano, 2016, 10, 11145–11155 CrossRef CAS.
- Q. Miao and K. Pu, Adv. Mater., 2018, 30, 1801778–1801800 CrossRef PubMed.
- Q. Tian, F. Jiang, R. Zou, Q. Liu, Z. Chen, M. Zhu, S. Yang, J. Wang, J. Wang and J. Hu, ACS Nano, 2011, 5, 9761–9771 CrossRef CAS.
- W. Li, D. Li, S. Meng, W. Chen, X. Fu and Y. Shao, Environ. Sci. Technol., 2011, 45, 2987–2993 CrossRef CAS PubMed.
- Z. F. Huang, J. Song, L. Pan, X. Zhang, L. Wang and J. J. Zou, Adv. Mater., 2015, 27, 5309–5327 CrossRef CAS PubMed.
- H. Huang, K. Li, Q. Liu, Y. Zhao, H. Xu, W. Wu, K. Sun, J. Ni and J. Lin, RSC Adv., 2019, 9, 2718–2730 RSC.
- C. Xia, D. Xie, L. Xiong, Q. Zhang, Y. Wang, Z. Wang, Y. Wang, B. Li and C. Zhang, RSC Adv., 2018, 8, 27382–27389 RSC.
- A. Watanabe, J. Solid State Chem., 2000, 153, 174–179 CrossRef CAS.
- M. Conrad, F. Wang, M. Nevius, K. Jinkins, A. Celis, M. Narayanan Nair, A. Taleb-Ibrahimi, A. Tejeda, Y. Garreau and A. Vlad, Nano Lett., 2016, 17, 341–347 CrossRef PubMed.
- G. Lu, X. Zou, F. Wang, H. Wang and W. Li, Mater. Lett., 2017, 195, 168–171 CrossRef CAS.
- X. Ding, C. H. Liow, M. Zhang, R. Huang, C. Li, H. Shen, M. Liu, Y. Zou, N. Gao and Z. Zhang, J. Am. Chem. Soc., 2014, 136, 15684–15693 CrossRef CAS PubMed.
- Y. Peng, Y. Liu, X. Lu, S. Wang, M. Chen, W. Huang, Z. Wu, G. Lu and L. Nie, J. Mater. Chem. B, 2018, 6, 2813–2820 RSC.
- M. Chang, M. Wang, Y. Chen, M. Shu, Y. Zhao, B. Ding, Z. Hou and J. Lin, Nanoscale, 2019, 11, 10129–10136 RSC.
- M. Chang, M. Wang, M. Shu, Y. Zhao, B. Ding, S. Huang, Z. Hou, G. Han and J. Lin, Acta Biomater., 2019, 99, 295–306 CrossRef CAS PubMed.
- W. Cao, X. Wang, L. Song, P. Wang, X. Hou, H. Zhang, X. Tian, X. Liu and Y. Zhang, RSC Adv., 2019, 9, 18874–18887 RSC.
- M. H. Huang, S. Rej and C. Y. Chiu, Small, 2015, 11, 2716–2726 CrossRef CAS PubMed.
- C. Liu, H. Dong, N. Wu, Y. Cao and X. Zhang, ACS Appl. Mater. Interfaces, 2018, 10, 6991–7002 CrossRef CAS PubMed.
- Y. Cheng, Y. Chang, Y. Feng, H. Jian, Z. Tang and H. Zhang, Angew. Chem., Int. Ed., 2018, 57, 246–251 CrossRef CAS PubMed.
- Y. Chang, Y. Cheng, Y. Feng, H. Jian, L. Wang, X. Ma, X. Li and H. Zhang, Nano Lett., 2018, 18, 886–897 CrossRef CAS PubMed.
- X. Deng, K. Li, X. Cai, B. Liu, Y. Wei, K. Deng, Z. Xie, Z. Wu, P. a. Ma and Z. Hou, Adv. Mater., 2017, 29, 1701266–1701274 CrossRef PubMed.
- A. Tanaka, S. Sakaguchi, K. Hashimoto and H. Kominami, ACS Catal., 2012, 3, 79–85 CrossRef.
- T. Zheng, T. Zhou, X. Feng, J. Shen, M. Zhang and Y. Sun, ACS Appl. Mater. Interfaces, 2019, 11, 31615–31626 CrossRef CAS PubMed.
- Q. Gao, A. Zhao, H. Guo, X. Chen, Z. Gan, W. Tao, M. Zhang, R. Wu and Z. Li, Dalton Trans., 2014, 43, 7998–8006 RSC.
- J. Liu and Y. Li, J. Mater. Chem., 2007, 17, 1797–1803 RSC.
- B. Liu, X. Deng, Z. Xie, Z. Cheng, P. Yang and J. Lin, Adv. Mater., 2017, 29, 1604878–1604886 CrossRef PubMed.
- C. Feng and X. Huang, Acc. Chem. Res., 2018, 51, 2314–2323 CrossRef CAS PubMed.
- B. Xu, Y. Liu, X. Sun, J. Hu, P. Shi and X. Huang, ACS Appl. Mater. Interfaces, 2017, 9, 16517–16523 CrossRef CAS PubMed.
- Y. Que, Y. Liu, W. Tan, C. Feng, P. Shi, Y. Li and H. Xiaoyu, ACS Macro Lett., 2016, 5, 168–173 CrossRef CAS.
- Y. Que, Z. Huang, C. Feng, Y. Yang and X. Huang, ACS Macro Lett., 2016, 5, 1339–1343 CrossRef CAS.
- R. Jiang, B. Li, C. Fang and J. Wang, Adv. Mater., 2014, 26, 5274–5309 CrossRef CAS PubMed.
- Q. Wu, M. Si, B. Zhang, K. Zhang, H. Li, L. Mi, Y. Jiang, Y. Rong, J. Chen and Y. Fang, Nanotechnology, 2018, 29, 295702–295712 CrossRef PubMed.
Footnote |
† Electronic supplementary information (ESI) available: Experimental section, zeta potential, hydrodynamic size, photothermal activity, DPBF depletion, MB depletion, linear relationship between PA signal and sample concentration, blood panel and serum biochemical index parameters. See DOI: 10.1039/c9ra09860g |
|
This journal is © The Royal Society of Chemistry 2020 |
Click here to see how this site uses Cookies. View our privacy policy here.