DOI:
10.1039/C9RA10965J
(Paper)
RSC Adv., 2020,
10, 10624-10633
Effects of ultrasound irradiation on the properties of apricot kernels during accelerated debitterizing
Received
27th December 2019
, Accepted 21st February 2020
First published on 12th March 2020
Abstract
In this paper, studies were conducted to investigate the effects of ultrasonically accelerated debitterizing on the physicochemical properties of apricot kernels, such as color, texture, oil content, protein characteristics and amino acids, with UV-Vis spectroscopy, synchronous fluorescence spectroscopy, circular dichroism, electrophoresis, environmental scanning electron microscopy, and thermal property analysis. The results indicate that the novel debitterizing technique has insignificant influences on the oil and protein contents of apricot kernels; meanwhile, the color, texture and activity of beta-glucosidase were substantially improved, greatly contributing to the quality modification and shortening the debitterizing time. In addition, ultrasound greatly influenced the amino acid contents and compositions, the fluorescence spectra and the thermal properties of the apricot kernel proteins. In a word, all these results greatly contribute to our understanding of the debitterizing mechanism mediated by ultrasound irradiation and further prove the feasibility of this novel debitterizing technique in the practical processing of apricot kernels.
1. Introduction
An apricot kernel is the fruit seed of apricots, which belongs to the genus Prunus of the subfamily Prunoideae in the family Rosaceae, and it is rich in many nutrients essential to the human body. To be specific, its oil content, mainly unsaturated fatty acids, is approximately 50.0% (g g−1);1 its protein content is 23.0–27.0%, which provides 15% of the total energy of the seeds, and is composed of 8 different essential amino acids, wherein the proportion of umami amino acids is larger.2 In addition, the contents of the total soluble sugars and dietary fibers account for about 5.5% and 12.2% of the whole seed weight, respectively.3 Based on these components, apricot kernels are considered to be one of the most nutritionally balanced foods in the human diet due to the benefits of these nutrients to human health, especially in controlling or preventing diseases such as cough, asthma, and cardiovascular diseases as well as weight maintenance.4 Amygdalin is a characteristic compound in apricot kernels; although its content is not the highest (2–5%),5 it is very important to the product development and function evaluation of apricot kernels. Amygdalin, also called vitamin B17 or nitriloside, is considered to be nontoxic by itself; however, when ingested, it is easily hydrolyzed by beta-glycosidase to yield one molecule of hydrogen cyanide (HCN), two molecules of glycose, and one molecule of benzaldehyde,6 and these metabolic products are potentially toxic. Cyanide can cause sudden death because it is rapidly taken up into cells and blocks mitochondrial electron transport within seconds. Due to their high amygdalin content and relatively facile release of HCN, apricot seeds have drawn more attention from toxicologists than other seeds.7 Generally, the toxicity depends on the intake dose of the seeds. In other words, a lower intake dose is beneficial to health, while a higher dose is toxic (the lethal dose of HCN is 0.5 to 3.5 mg kg−1 body weight).8 Therefore, the debitterizing operation is an indispensable step during the processing of apricot kernels, through which the content of amygdalin is reduced to safe limits, resulting in the removal of the bitterness and potential toxicity of apricot kernels.9
The commonly employed methods for debitterizing apricot kernels include two categories: conventional and novel methods. The former comprises cold water debitterizing,10 hot water debitterizing,11 acid solution debitterizing and acid-base alternative debitterizing, while the latter includes vacuum debitterizing,12 ultrasound-assisted debitterizing and microwave-assisted debitterizing.13 Regarding conventional debitterizing methods, the main disadvantages are high energy consumption, time waste (from 6 to 7 hours to 6 to 7 days), labor-intensiveness, high pollution and high loss of nutritional compounds in apricot kernels. For instance, the contents of proteins, carbohydrates, phenols, flavonoids and amygdalin have been detected in the debitterizing water concentrate (DWC) generated from conventional industrial debitterizing, and the total loss of weight is about 17% of the apricot kernels after debitterizing.14 In comparison, novel techniques are relatively rapid, eco-friendly, low-cost and readily industrialized. Among these, ultrasound is regarded as the most promising technique for accelerating the debitterizing of apricot kernels.15 As a non-thermal processing technology, ultrasound irradiation usually influences the components and physicochemical properties of food by generating high-frequency vibrations and acoustic cavitation. In our previous studies, ultrasound was proved to greatly reduce the debitterizing time to 1 to 2 h, and the water consumption could also be decreased greatly;15 meanwhile, little information is available about the effects of ultrasound irradiation on the physicochemical properties of apricot kernels, such as color, protein and oil, during rapid debitterizing, which determines the quality of the debitterized apricot kernels to a great extent.
Ultrasonically accelerated debitterizing is basically regarded as the extraction of amygdalin driven by ultrasound irradiation from the apricot seeds into the debitterizing solution. As a novel technology, ultrasound-assisted extraction (UAE) has attracted much attention and has been widely used in the fields of natural products extraction in recent years, such as the extraction of antioxidant compounds,16 polyphenols,17 and ginsenosides.18 Generally, the mechanism of UAE is attributed to the synergistic performance of the cavitation, mechanical and thermal effects of the ultrasound, which can destroy the cell walls, reduce particle sizes and enhance the mass transfer, resulting in increased extraction efficiency.19 Also, the acoustic cavitation produced from the propagation of ultrasound waves is usually considered to be the main effect. In addition, ultrasound irradiation can cause greater penetration of the solvent into the sample matrix through mechanical effects, improving the contact area between the solid and the liquid; as a result, the target compounds rapidly diffuse from the solid into the solvent.20 Great attention has been paid to the mechanical and physical aspects of UAE in order to explain its mechanism; meanwhile, its effects on non-target compounds in the sample are often neglected, which is exactly what we are concerned with. That is to say, the effects of ultrasound irradiation on the quality of apricot kernels should be seriously considered during accelerated debitterizing.
The aim of this study was to investigate the effects of ultrasonically accelerated debitterizing on the physicochemical properties of apricot kernels, including their color, texture, chemical composition, proteins and amino acids, to understand the mechanism of debitterizing by ultrasound and its effects on the quality of the seeds. All these results provide a theoretical base to evaluate the feasibility of ultrasonically accelerated debitterizing in the practical production of apricot kernels.
2. Materials and methods
2.1. Materials and reagents
Apricot kernels were purchased from the Northwest Herb Market in the city of Xi'an (Shaanxi Province, China). The water content of the apricot kernels is about 4.84 g water/100 g seeds. The beta-glucosidase activity assay kit, BCA protein assay kit (50 T) and SDS-PAGE gel preparation kit were all purchased from Solarbio Co. Ltd. (Beijing, China). Amygdalin (98.42% pure) was purchased from Chengdu Preferred Bio-Technology Co. Ltd (Chengdu, Sichuan Province, China). HPLC-grade methanol (99.9% pure) was bought from Thermo Fisher Scientific Co. Ltd (Shanghai, China). All other reagents were of analytical grade, including sodium hydroxide, sodium carbonate, glycerol, bovine serum protein, G-250, 3,5-dinitrosalicylic acid, glucose, hydrochloric acid, Folin–Ciocalteu reagent, and sodium chloride. These reagents were purchased from Tianli Chemical Reagent Co. Ltd (Tianjin, China). Ultrapure water was prepared using a Millipore Milli-Q purification system.
2.2. Preparation of debitterized apricot kernels by ultrasound irradiation
360 g of apricot kernels peeled with hot water were placed in a 5000 mL beaker containing 4320 mL water (1
:
12, g
:
mL), which was sealed with Parafilm to avoid evaporation of the solution. Debitterizing was conducted under the conditions of 55 °C, 59 kHz and 300 W, with the beaker fixed at a designated position in the ultrasonic bath (SB-500DTY, Ningbo Xinzhi Biotechnology Co. Ltd., Ningbo City, Zhejiang Province, China).15,21 During the operation, samples were collected at intervals; each time, 30 g apricot kernels and 360 mL debitterizing solution were respectively taken out until the debitterizing was complete. After that, the physicochemical properties of the collected samples were investigated, including amygdalin, color, texture, and proteins, to understand the effects of the ultrasonically accelerated debitterizing on the quality of the apricot kernels.
2.3. Determination of moisture content
The moisture content was determined for the above-collected samples after heating them an oven at a temperature of 105 °C to a constant weight.13
2.4. Determination of the amygdalin and beta-glucosidase activities of the apricot kernels
A certain amount of each collected sample was weighed and powdered; then, the amygdalin was extracted from the powder of apricot kernels with methanol (1
:
10, g
:
mL) under the conditions of ultrasound power (300 W), 59 kHz (frequency), 35 °C (temperature) and 45 min (ultrasound time).13 Subsequently, the amygdalin in the extraction solution was determined according to the reference with a slight modification.11 To be specific, the amygdalin was separated by an HPLC system (Dalian Elite Analytical Instruments Co. Ltd., Liaoning, China) consisting of two P230II pumps, a UV230II ultraviolet visible detector and a ZW230II column oven. A column of TC-C18 (250 mm × 4.6 mm, 5 μm) was placed in the column with the oven set at 35 °C. The mobile phase consisted of water and methanol (90
:
10, mL
:
mL, degassed by ultrasound for 20 min), and the flow rate was 1 mL min−1. The detection wavelength was 215 nm, and the injection volume was 10 μL. The amygdalin was identified by comparing the retention time with that of the corresponding injected standard, and its content was calculated according to the working curve constructed by the external standard method.
Considering that beta-glucosidase greatly contributes to the debitterizing of apricot kernels, the beta-glucosidase activity was investigated for the samples according to the ref. 15.
For ease of comparison, the contents are all expressed as the percentage of the dry base weight with subtraction of the moisture content.
2.5. Determination of oil content
The apricot kernels were dried and ground into powder with a cyclone mill, then placed into a conical flask (150 mL) with petroleum ether as the solvent. Finally, the oil was ultrasonically extracted according to the literature,11 and the defatted powder was stored at −18 °C until further analysis.
2.6. Color and texture analysis of the apricot kernels
The color of the apricot kernel powder was measured by an automatic color difference meter (SC-80C, Beijing Kangguang Optical Instrument Co., Ltd., China) and the parameters of L*, a*, b* and ΔE were recorded.
The texture of the apricot kernels was measured by a texture analyzer (TA.XTPlus, Stable Micro Systems, United Kingdom), including the hardness, fracturability, adhesiveness, springiness, cohesiveness, gumminess, chewiness and resilience of the apricot kernels, to understand the effects of ultrasound irradiation on the organoleptic properties of the debitterized apricot kernels.
2.7. Effects of the ultrasonically accelerated debitterizing on the physicochemical properties of proteins in apricot kernels
2.7.1. Extraction and determination of the protein of apricot kernels. Before and after debitterizing, the protein content of the apricot kernels was measured using an automatic Kjeldahl apparatus (Kjeltec 2300, FOSS, Sweden).The defatted powder and deionized water were mixed in a ratio of 1
:
25 (g mL−1), followed by adjusting the value of pH to 9.0 with 1 mol L−1 NaOH solution and stirring for 1 h at 40 °C. Subsequently, the mixture was centrifugated and the pH of the collected supernatant was adjusted with 1 mol L−1 HCl solution to 4.5, followed by centrifugation at 4000 rpm for 20 min; the precipitate was finally obtained, frozen under vacuum and stored for further use.
2.7.2. Measurement of UV-Vis spectra of the protein. The dissolved solution of the above-frozen protein was placed in a 1.0 cm quartz cell and scanned in the wavelength range of 200–300 nm with a TU-1810 spectrophotometer (PERSEE Analytical Instrument Co. Ltd., Beijing, China).15
2.7.3. Fluorescence spectroscopy analysis of the proteins. The protein solutions were measured by a fluorescence spectrophotometer (RF-6000, Shimadzu Co. Ltd., Japan) with the conditions of 25 ± 1 °C (temperature), 290–450 nm (scanning wavelength), 280 nm (excitation wavelength), 600 nm min−1 (scanning rate), a recording frequency of 0.5 nm per time, and slit widths of excitation and emission of 5 and 10 nm, respectively.
2.7.4. Circular dichroism (CD) analysis of the proteins. The CD spectrum of the protein in borate buffer solution (0.01 mol L−1, pH 7.4) was scanned in the range of 190–260 nm to investigate the changes in the secondary structures of the proteins by a circular dichroism spectrometer (Chirascan, Applied Photophysics Co. Ltd., United Kingdom), and the recorded UV-Vis spectra were analyzed according to the ref. 15.
2.7.5. Sodium dodecyl sulfate polyacrylamide gel electrophoresis (SDS-PAGE) analysis of the proteins. The gels were prepared according to the instructions of the SDS-PAGE gel preparation kit, and 10 μL of sample was loaded. The whole analysis was conducted according to the ref. 22.
2.7.6. Morphology analysis of the proteins by environmental scanning electron microscope (ESEM). Protein samples were mounted on bronze stubs using double-sided adhesive tape to allow surface visualization; the samples were then coated with a layer of gold (40–50 nm) in a sputter coater to avoid charging under the electron beam. Subsequently, the morphologies of the samples were investigated by a scanning electron microscopy spectrometer (Quanta-200, Philips-FEI Company, Amsterdam, Netherlands) under the conditions of 20 kV (operating voltage) and 15 Pa (vacuum). Measurements were taken in triplicate for each sample.
2.7.7. Thermal properties analysis of the proteins. A thermal analysis system (Q1000DSC + LNCS + FACS Q600SDT, TA Instruments Co. Ltd., America) was used to investigate the changes in the thermal properties of the proteins.15,22 To be specific, the protein sample was maintained at 30 °C for 1 min and then studied by differential scanning calorimetry (DSC) in the increasing temperature range from 30 °C to 140 °C at a rate of 10 °C min−1 and a nitrogen flow rate of 20 mL min−1. The thermal denaturation temperatures (Td) and enthalpy changes (ΔH) of the samples were calculated according to the software, respectively. Thermogravimetric analysis (TGA) of the proteins was investigated within the temperature range of 0–700 °C, and the relationships between the temperature and the protein weight were analyzed by the same heating rate and nitrogen flow rate used in the DSC experiments.
2.7.8. Amino acid analysis of the proteins. The amino acid compositions were determined using a HITACHI L-8900 automatic amino acid analyzer (Hitachi, Tokyo, Japan) according to the ref. 22 and 23. Three replicates were analyzed for each sample.
2.8. Statistical analysis
All the results were statistically analyzed by calculating the mean and standard deviation and are presented as the mean ± standard deviation of three determinations. Analysis of variance (ANOVA) was conducted using SPSS statistics software, version 20.0 (SPSS Inc. Chicago, IL, USA).
3. Results and discussion
3.1. Changes in amygdalin during the ultrasonically accelerated debitterizing of apricot kernels
As shown in Fig. 1, the content of amygdalin remaining in the apricot kernels quickly decreased with increasing ultrasound time; the residues were less than 0.91 mg g−1, which is a threshold value to evaluate whether the apricot kernel is bitter.13 That is to say, the apricot kernels had no bitter taste when the debitterizing time reached 90 min or longer. Compared with conventional hot-water debitterizing, ultrasonically accelerated debitterizing could greatly shorten the required time from 7 to 8 h to about 1.5 h,11 suggesting the feasibility of this novel technique. Generally, two reasons can explain the high efficiency of ultrasonically accelerated debitterizing. Firstly, the mechanical and cavitation effects of ultrasound waves can produce a large shear force and many microbubbles; this breaks the boundary layer, strengthens the surface erosion, and increases the contact and collision between the materials and the liquids, resulting in rapid mass transfer or degradation of the amygdalin in the apricot kernels.24 Secondly, the ultrasound cavitation can also activate the activity of beta-glucosidase,15 which subsequently accelerates the degradation of amygdalin, disrupts the original balance and increases the dissolution of the amygdalin, resulting in shortening of the debitterizing time. Furthermore, it is worth mentioning that the aim of debitterizing is not to completely remove all the amygdalin from the apricot kernels, but to allow them to retain a certain amount of amygdalin at which the bitterness cannot be tasted; in this way, the positive bioactive function of the amygdalin can also be protected. Considering this, the apricot kernels ultrasonically debitterized for 90 min were regarded as completely debitterized; this sample of debitterized apricot kernels was used in the following experiments.
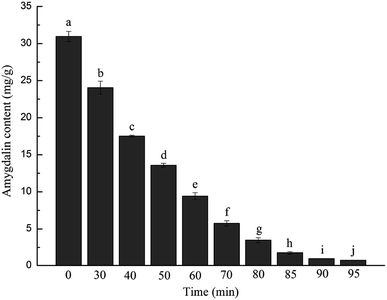 |
| Fig. 1 Changes in amygdalin content during the ultrasonically accelerated debitterizing of apricot kernels. | |
3.2. Color changes of apricot kernels before and after debitterizing
The CIELAB system with the parameters of L*, a*, b* has been widely employed for measuring color differences in the food industry; L* indicates lightness (0 to 100), with 0 being black and 100 being white, the coordinate a* is for red (+) and green (−), and the coordinate b* is for yellow (+) and blue (−). Furthermore, the limits of a* and b* are approximately + or −80.25 The values of a* were 9.14 ± 0.39 and 5.44 ± 0.22 and the values of b* were 22.55 ± 3.60 and 13.14 ± 0.77 before and after debitterizing; it is obvious that significant changes in the values of a* and b* occurred for the apricot kernels after being ultrasonically debitterized, while there is no significant difference in the L* values (94.52 ± 0.01 and 95.80 ± 0.80). To be specific, the a* and b* values significantly decreased after debitterizing, suggesting that the red and yellow colors decreased, while the brightness of the apricot kernels was not greatly influenced. ΔE is the comprehensive color difference deviation, and the theoretical limit of perception of the human eye (ΔE = 3.0) has been suggested as an absolute color discrimination threshold for food. Regarding the parameters of a*, b* and ΔE, it can be concluded that the debitterizing can change the color of apricot kernels by decreasing the levels of red and yellow; this variation can be recognized by the naked human eye because the ΔE value of 10.27 is much higher than the threshold value of 3.0. Also, these variations can be attributed to the transfer or removal of phenolic compounds from the external surface of the peeled apricot kernel, which is driven by ultrasound.26
3.3. Texture variations of apricot kernels after debitterizing
Generally, texture can reflect the taste of food and must regarded as an important indicator to evaluate the sensory properties of food; a texture analyzer is usually employed to determine the texture characteristics of food.27 As shown in Table 1, the hardness of the ultrasonically debitterized apricot kernels was lower than that of the untreated apricot kernels, and similar changes occurred in the cohesiveness, gumminess, chewiness and resilience. In contrast, the fracturability, adhesiveness and springiness of the ultrasonically treated samples were higher than those of the untreated apricot kernels. All these results greatly contribute to explain the difference in the organoleptic properties between the untreated and debitterized apricot kernels. Overall, ultrasound can not only accelerate the debitterizing but can also influence the sensory properties of apricot kernels, in turn affecting their edible and commercial value.
Table 1 Texture parameters of apricot kernels before and after debitterizinga
Parameter |
Apricot kernels |
Debitterized apricot kernels |
Different letters in the same row indicate significant differences at p < 0.05. |
Hardness/Ha (×104) |
3.21 ± 0.15a |
2.71 ± 0.05b |
Fracturability/Fr (×104) |
0.93 ± 0.03a |
1.12 ± 0.18a |
Adhesiveness/Ad |
−105.42 ± 6.72a |
−16.80 ± 2.29b |
Springiness/Sp |
0.51 ± 0.04a |
0.57 ± 0.02a |
Cohesiveness/Co |
0.51 ± 0.05a |
0.33 ± 0.04b |
Gumminess/Gu (×104) |
1.33 ± 0.03a |
0.64 ± 0.03b |
Chewiness/Ch (×103) |
6.30 ± 0.29a |
3.69 ± 0.30b |
Resilience/Re |
0.35 ± 0.02a |
0.17 ± 0.02b |
3.4. Changes in the moisture, protein and oil contents, and beta-glucosidase activity of apricot kernels
As shown in Fig. 2(A), the water content of the apricot kernels increased significantly after debitterizing; this can enable the amygdalin to be easily dissolved, transferred and degraded, resulting in accelerated debitterizing and texture variation of the apricot kernels. In the meantime, the moisture content can also be used to monitor the drying procedure and calculate the dry weight of the components. The activity of beta-glucosidase was greatly improved after ultrasonically accelerated debitterizing, as shown in Fig. 2(B); this can be attributed to the effects of ultrasound cavitation on the conformation and secondary structure of the enzymes15 and the increased water content in the apricot kernels because the activity of beta-glucosidase will increase once the enzyme contacts the water in the seed. Apricot kernels contain high amounts of recommended monounsaturated oleic acids, a moderate amounts of linoleic acid and low amounts of saturated fatty acids; thus, its fatty acid composition may be more favorable than that of olive oil. Additionally, the oils contain active compounds such as vitamin E.28 Regarding the contents of protein and oil, there were no significant differences after debitterizing, as shown in Fig. 2(C) and (D); i.e. the ultrasonic debitterizing did not cause great loss of these components, which is different from the results obtained from traditional hot-water debitterizing,11 illustrating the advantage of ultrasonically accelerated debitterizing.29 In addition, ultrasonic treatment is different from microwave treatment of apricot kernels, which changes their oil content and fatty acid composition.30
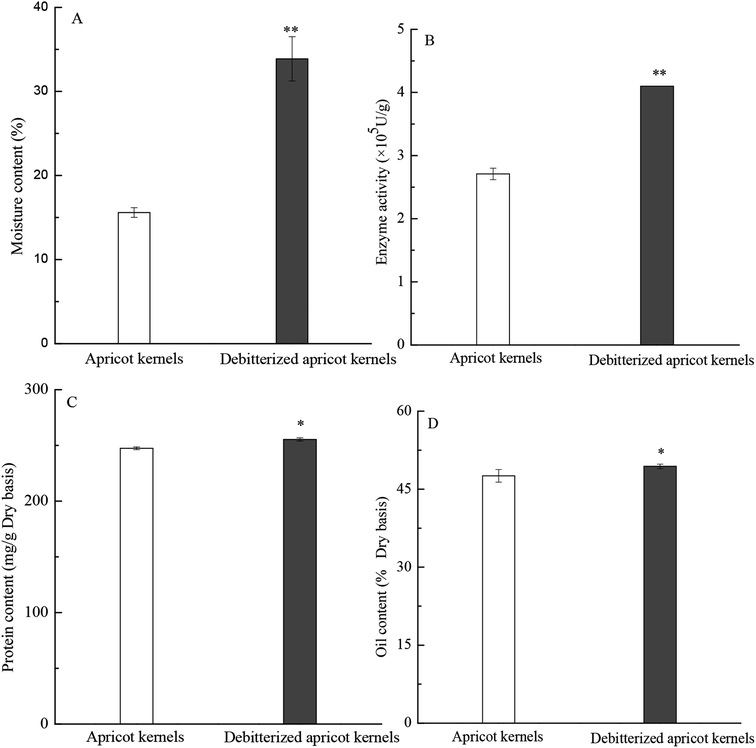 |
| Fig. 2 (A) Moisture, (B) the beta-glucosidase activity, (C) protein contents and (D) oil of apricot kernels before and after debitterizing. | |
3.5. Effects of ultrasonically accelerated debitterizing on the physicochemical properties of proteins in apricot kernels
3.5.1. Protein analysis by UV-Vis spectrum. Spectroscopy is a commonly used qualitative method to study the conformational changes of proteins because it allows non-invasive measurements of substances with lower concentrations and mild conditions.31 As shown in Fig. 3(A), the spectrum contains the typical absorption peaks of the protein, and the stronger peak at 210 nm accounts for the peptide bonds. After debitterizing, the peak intensity of the proteins at 210 nm declined, with a slight bathochromic shift from 210 nm to 212 nm; this implies the loosening and unfolding of the protein skeleton. Also, the decrease of the absorbance was very obvious in the range of 225–270 nm, which may indicate that the structure of the apricot kernel protein was changed by the ultrasound irradiation during the accelerated debitterizing, consequently influencing the stability of the protein.
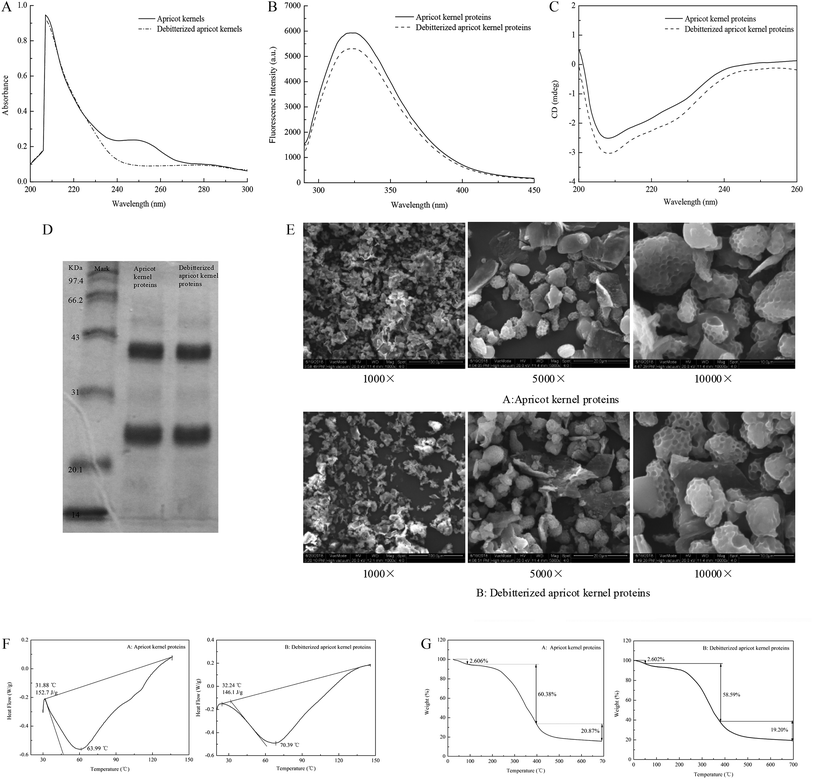 |
| Fig. 3 Physicochemical properties of the apricot kernel proteins before and after debitterizing. (A) UV-Vis spectra, (B) synchronous fluorescence spectra, (C) CD spectra, (D) electropherogram, (E) ESEM images, (F) DSC analysis, (G) and TGA analysis of apricot kernel proteins. | |
3.5.2. Protein analysis by fluorescence spectroscopy. In addition to UV-Vis spectrum analysis, synchronous fluorescence spectroscopy is commonly used to study the conformation of proteins; it can provide characteristic information about the molecular environment in the vicinity of fluorophores such as tryptophan (Trp) and tyrosine (Tyr). In addition, it has some advantages, such as simplifying the spectrum, reducing the spectral bandwidth and avoiding different perturbing effects.32 As shown in Fig. 3(B), the fluorescence intensity of the proteins greatly decreased after debitterizing, and a characteristic fluorescence emission spectrum of the protein is displayed with a maximum value at 325 nm due to the tryptophan (Trp) residues. The fluorescence spectrum of the proteins from the debitterized apricot kernels presented red shifts from 324 to 325.5 nm, and the maximum intensity decreased from 5944 to 5390 a.u.; this suggests enhanced polarity of the environment and reduced hydrophobicity of the proteins by ultrasound,32 such as damages to the hydrophobic groups in the tryptophan and tyrosine residues of the proteins.
3.5.3. Protein analysis by CD. The secondary structure of proteins is typically resolved through CD analysis; therefore, the CD spectra of the apricot kernel proteins were recorded in the range of 200–260 nm in order to further investigate the conformational changes induced by ultrasound irradiation. Generally, a large positive peak near 190 to 200 nm reflects parallel and anti-parallel structures, which are collectively due to beta-sheet structures. Meanwhile, a negative valley in the range of 200–220 nm indicates α-helical and random coil structures.33 As shown in Fig. 3(C), the negative and weakly positive peaks suggest that the secondary structures conform to the CD characteristics of proteins. CDNN software was used to perform the fitting calculations of the CD spectra to obtain the secondary structure contents of the apricot kernel proteins before and after debitterizing, and the results are listed in Table 2. The total amounts of the secondary structures were 120.2% and 119.6% for the proteins of the untreated apricot kernels and debitterized apricot kernels, respectively. Although there is no significant difference in the total amounts of the secondary structure contents before and after debitterizing, the individual contents of the five components changed to a certain extent. To be specific, the contents of the antiparallel and β-turn structures decreased, while the parallel structures increased; this can be attributed to changes in the force action within the proteins, such as hydrophobic interactions and hydrogen bonds. These forces can be interfered with or disrupted by the quicker nonlinear oscillation of the cavitation bubbles and the more frequent pulses of the local instant high temperature and pressure triggered by ultrasound,24 and all these variations may contribute to the explanation of the texture changes of ultrasonically debitterized apricot kernels.
Table 2 Contents of secondary structures of apricot kernel proteins before and after debitterizing
Secondary structure |
Apricot kernel protein |
Debitterized apricot kernel protein |
α-Helix (%) |
15.40 |
16.00 |
Antiparallel (%) |
17.70 |
16.20 |
Parallel (%) |
16.70 |
17.80 |
β-Turn (%) |
21.30 |
20.10 |
Random coil (%) |
49.10 |
49.50 |
3.5.4. Protein analysis by SDS-PAGE. The electropherograms of the apricot kernel proteins before and after debitterizing are shown in Fig. 3(D). According to the SDS-PAGE results, both the untreated and debitterized apricot kernel proteins contained two high abundance bands which correspond to the molecular weights of 22–26 kDa and 35–43 kDa, respectively. Regarding the color of the stained proteins, a slight fading occurred in the debitterized apricot kernel proteins and the bandwidth also slightly narrowed; this suggests that the structure of the proteins was ultrasonically altered to a certain extent during debitterizing of the apricot kernels.34
3.5.5. Morphology analysis of proteins by ESEM. As shown in Fig. 3(E), the morphology of the proteins was investigated at magnifications of 1000, 5000 and 10
000 times by ESEM. Overall, the differences in the morphology of the protein aggregations of the two samples were significant. In the pictures magnified 1000 times, the proteins of the untreated apricot kernels are closely clustered together, while the proteins of the ultrasonically-accelerated debitterized apricot kernels are slightly dispersed. In the images magnified 5000 times, the surface of the proteins can be observed to contain many pores. Furthermore, the protein surface of the debitterized samples was slightly damaged in comparison with the sample without debitterizing.35 With further magnification of 10
000 times, there is no significant difference in the morphology of the proteins; however, the morphology of the sample became slightly more porous and was slightly damaged after debitterizing by ultrasound irradiation. In a word, the morphology of the proteins was not greatly influenced by the novel ultrasonically accelerated debitterizing of apricot kernels, which is consistent with some of the results obtained above by other analyses.
3.5.6. DSC analysis of apricot kernel proteins. DSC is considered to be an important technique for analyzing the thermal properties of proteins; changes in the denaturation temperature and enthalpy can be resolved according to the peak temperature and peak area of the DSC curve.22 As shown in Fig. 3(F), the thermal denaturation temperatures (Td) of the apricot kernel proteins before and after debitterizing are 63.99 °C and 70.39 °C, respectively. In comparison, the denaturation temperature of the protein increased by about 7 °C after the apricot kernels were treated with ultrasound irradiation, likely reflecting the higher thermal stability of the proteins caused by the ultrasonically accelerated debitterizing. The value of the enthalpy change (ΔH) decreased from 152.7 J g−1 to 146.1 J g−1 for the apricot kernel proteins after debitterizing by ultrasound. Generally, ΔH, which is usually associated with the spatial structure of proteins, represents the amount of heat required for the denaturation of the tested proteins, and its value can reflect the proportion of denatured proteins. Moreover, the smaller the ΔH value, the higher the disorder of the protein and the lower the degree of aggregation.36 Considering the results, the conclusion can be made that the disorder of the proteins increased and the aggregation degree decreased after ultrasound irradiation of the apricot kernels. In a word, ultrasound irradiation may have a certain influence on the internal structure of the proteins, resulting in changes in some physicochemical properties, such as thermal and spectroscopic characteristics.
3.5.7. TGA analysis of apricot kernel proteins. Generally, TGA is employed to study changes in the mass/weight and thermal information of a sample with increasing temperature. As shown in Fig. 3(G), all the TGA curves of the proteins were divided into three stages, and the greatest weight losses all occurred in the second stage. Generally, the mass loss in the first phase is thought to arise mainly from the loss of free water and the structure variations of the proteins due to the high temperature.37 The second stage is usually considered to represent the procedure of thermal decomposition due to the higher temperature, in which the bounded water inside the proteins is lost and some groups such as hydroxyls are also destroyed, resulting in the highest weight loss of proteins at this stage. The minimum mass loss in the third stage is usually attributed to the degradation of impurities in the samples. Overall, under the same programmed conditions of increasing temperature, the loss of 83.856% of the untreated apricot kernel proteins was higher than that of 80.392% of the debitterized apricot kernel proteins; this can be attributed to the reduced ordering and aggregation of the internal structures of the proteins by ultrasound irradiation. In a word, ultrasonically accelerated debitterizing can affect the thermal properties and internal structures of the proteins, including the activity of enzymes, resulting in rapid debitterizing and variations of the sensory properties of the apricot kernels.
3.5.8. Amino acid composition analysis of apricot kernel proteins. Apricot kernel proteins are rich in amino acids; as shown in Table 3, 17 amino acids were detected in both samples, including essential amino acids such as threonine, valine, methionine, isoleucine, leucine, phenylalanine, lysine and histidine. In comparison, the amounts of amino acids in the debitterized apricot kernel proteins were higher than those in the non-debitterized sample. Notably, the debitterized apricot kernel proteins contained more glutamic acid in comparison with the apricot kernel proteins without debitterizing; the reasons for this remain unclear. Meanwhile, the calculated contents of EAA/NEAA and EAA/TAA of the samples are close to the ideal protein standards specified by the WHO/FAO, indicating that the amino acid compositions of the apricot kernel proteins are close to those required by the human body. In addition, other parameters to evaluate the amino acids were calculated and are listed in Table 3; most of the values for the amino acids of the debitterized apricot kernel proteins are higher than those of the non-debitterized sample. Overall, it appears that the nutritional value of apricot kernel protein can be improved by ultrasound debitterizing according to the amino acid analysis.38
Table 3 Amino acid compositions and evaluation parameters of apricot kernel proteins before and after debitterizing (g/100 g protein/dry basis)a
Amino acid |
Apricot kernel protein |
Debitterized apricot kernel protein |
Different letters in the same row indicate significant differences at p < 0.05. |
Aspartic acid/Asp |
8.54 ± 0.40a |
9.56 ± 0.25b |
Threonine*/Thr |
2.26 ± 0.09a |
2.51 ± 0.08a |
Serine/Ser |
2.64 ± 0.11a |
3.51 ± 0.11b |
Glutamic acid/Glu |
15.28 ± 0.12a |
19.32 ± 0.45b |
Proline/Pro |
6.42 ± 0.45a |
6.07 ± 1.21a |
Glycine/Gly |
3.62 ± 0.28a |
4.20 ± 0.14b |
Alanine/Ala |
3.83 ± 0.29a |
3.99 ± 0.10a |
Cystine/Cys |
1.38 ± 0.07a |
1.69 ± 0.05a |
Valine*/Val |
3.55 ± 0.02a |
3.57 ± 0.10a |
Methionine*/Met |
0.42 ± 0.05a |
0.13 ± 0.02b |
Isoleucine*/Ile |
3.12 ± 0.11a |
3.66 ± 0.06a |
Leucine*/Leu |
5.38 ± 0.12a |
6.08 ± 0.19b |
Tyrosine/Tyr |
1.98 ± 0.04a |
2.45 ± 0.08a |
Phenylalanine*/Phe |
4.24 ± 0.01a |
4.81 ± 0.21b |
Lysine*/Lys |
2.55 ± 0.18a |
2.66 ± 0.12a |
Histidine/His |
2.14 ± 0.06a |
2.39 ± 0.17a |
Arginine/Arg |
7.28 ± 0.18a |
8.47 ± 0.44b |
Total amino acids/TAA |
74.62 ± 0.05a |
85.06 ± 3.78b |
Essential amino acids/EAA |
21.50 ± 0.03a |
23.41 ± 0.79a |
Children essential amino acids/CEAA |
9.42 ± 0.24a |
10.85 ± 0.61b |
Non-essential amino acids/NEAA |
53.12 ± 0.05a |
61.65 ± 3.00b |
Hydrophobic (non-polar) amino acids/NAA |
26.95 ± 0.80a |
20.30 ± 1.89b |
Hydrophilic (polar) amino acids/PAA |
8.26 ± 0.13a |
10.16 ± 0.32b |
Basic amino acids/BAA |
11.97 ± 0.06a |
13.51 ± 0.73b |
Acidic amino acids/AAA |
23.82 ± 0.52a |
28.88 ± 0.70b |
Aromatic amino acids/ARAA |
6.21 ± 0.05a |
7.26 ± 0.29b |
Sulphur amino acids/SAA |
1.80 ± 0.03a |
1.82 ± 0.08a |
EAA/NEAA |
0.40 ± 0.00a |
0.38 ± 0.00a |
EAA/TAA |
0.29 ± 0.00a |
0.28 ± 0.00a |
CEAA/TAA |
0.13 ± 0.00a |
0.13 ± 0.00a |
4. Conclusions
Ultrasonically accelerated debitterizing did not significantly influence the oil and protein contents of apricot kernels, with only a very small weight loss caused by ultrasound during the debitterizing; meanwhile, the color, texture and the activity of beta-glucosidase were greatly improved, which greatly contributes to the quality modification and shortens the debitterizing time. Considering the effects of debitterizing on the properties of apricot kernel proteins, the results indicate that ultrasound did greatly influence the amino acid contents and compositions, the fluorescence spectrum, and the thermal properties of the apricot kernel proteins, i.e. ultrasound affected the molecular and structural characteristics of the proteins. In a word, all these results greatly contribute to the understanding of the debitterizing mechanism mediated by ultrasound irradiation and further proved the feasibility of this novel debitterizing technique in practical processing of apricot kernels. However, modulation of this technique should be further investigated to reduce the loss of weight and nutrients and increase the improvement of the quality and efficiency in the actual production of apricot kernels.
Conflicts of interest
The authors of the present work declare no conflict of interest.
Acknowledgements
This study was supported by the National Natural Science Foundation of China [Nos. 31101324, 31972206] and the Key Research Development Program of Shaanxi Province, China [No. 2018ZDXM-NY-086].
References
- G. Egea, M. M. González-Real, A. Baille, P. A. Nortes, P. Sánchez-Bel and R. Domingo, Agric. Water Manag., 2009, 96, 1605–1614 CrossRef.
- D. P. Richardson, A. Astrup, A. Cocaul and P. Ellis, Food Sci. Technol. Bull. Funct. Foods, 2009, 6, 41–50 CrossRef.
- S. Yada, K. Lapsley and G. W. Huang, J. Food Compos. Anal., 2011, 24, 469–480 CrossRef CAS.
- S. G. Li, F. Geng, P. Wang, J. K. Lu and M. H. Ma, J. Sci. Food Agric., 2016, 96, 3351–3357 CrossRef CAS.
- I. F. Bolarinwa, C. Orfila and M. R. Morgan, Food Chem., 2014, 152, 133–139 CrossRef CAS PubMed.
- Z. Zdrojewicz, A. Otlewska, P. Hackemer and A. Otlewska, Pol. Merkuriusz Lek., 2015, 38, 300–303 Search PubMed.
- N. Karsavuran, M. Charehsaz, H. Celik, B. M. Asma, C. Yakìncì and A. Aydìn, Toxicol. Environ. Chem., 2014, 96, 1564–1570 CrossRef CAS.
- I. F. Bolarinwa, C. Orfila and M. R. A. Morgan, Food Chem., 2015, 170, 437–442 CrossRef CAS PubMed.
- S. Sahin, J. Health Med. Inf., 2011, 2, 106–107 Search PubMed.
- A. Silem, H. O. Günter, J. Einfeldt and A. Boualia, Int. J. Food Sci. Technol., 2006, 41, 201–213 CrossRef CAS.
- Y. Song, Q.-A. Zhang, X. H. Fan and X. Y. Zhang, J. Food Process. Preserv., 2018, 42, 1–8 Search PubMed.
- Q. H. Zhang, J. Li, J. H. Pang, J. Cui and J. Z. Wang, Sci. Technol. Food Ind., 2014, 35, 248–252 CAS.
- N. Zhang, X. Y. Zhang, X. H. Fan and Q.-A. Zhang, Food & Machinery, 2018, 34, 189–194 Search PubMed.
- Q.-A. Zhang, C. X. Wei, X. H. Fan and F. F. Shi, CyTA - J. Food, 2018, 16, 422–428 CrossRef CAS.
- X. H. Fan, X. Y. Zhang, Q.-A. Zhang, W. Q. Zhao and F. F. Shi, Ultrason. Sonochem., 2019, 52, 468–476 CrossRef CAS PubMed.
- M. B. Hossain, N. P. Brunton, A. Patras, B. Tiwari, C. P. O'Donnell, A. B. Martin-Diana and C. Barry-Ryan, Ultrason. Sonochem., 2012, 19, 582–590 CrossRef CAS PubMed.
- S. S. Teh and E. J. Birch, Ultrason. Sonochem., 2014, 21, 346–353 CrossRef CAS.
- H. M. Lin, Y. G. Zhang, M. Han and L. M. Yang, Ultrason. Sonochem., 2013, 20, 680–684 CrossRef CAS.
- S. R. Shirsath, S. H. Sonawane and P. R. Gogate, Chem. Eng. Process, 2012, 53, 10–23 CrossRef CAS.
- B. Kumari, B. K. Tiwari, M. B. Hossain, N. P. Brunton and D. K. Rai, Food Bioprocess Technol., 2018, 11, 223–241 CrossRef CAS.
- N. Zhang, Q.-A. Zhang, J. L. Yao and X. Y. Zhang, Ultrason. Sonochem., 2019, 58, 104614 CrossRef PubMed.
- S. G. Li, S. Chu, J. K. Lu, P. Wang and M. H. Ma, J. Food Process. Preserv., 2018, 42, 1–7 CrossRef PubMed.
- R. Shibue, T. Sasamoto, M. Shimada, B. Zhang, A. Yamagishi and S. Akanuma, Sci. Rep., 2018, 8, 1–8 CrossRef CAS PubMed.
- Q.-A. Zhang, H. Shen, X. H. Fan, Y. Shen, X. Wang and Y. Song, Ultrason. Sonochem., 2015, 22, 149–154 CrossRef CAS PubMed.
- S. Suzanne Nielsen, Food analysis laboratory manual, Springer, New York, Dordrecht, Heidelberg, London, 2nd edn, 2010, DOI:10.1007/978-1-4419-1463-7.
- L. Feng, M. Zhang, B. Adhikari and Z. M. Guo, Food Bioprocess Technol., 2018, 11, 1328–1338 CrossRef CAS.
- F. Hamedi, M. Mohebbi, F. Shahidi and E. Azarpazhooh, Food Bioprocess Technol., 2018, 11, 1061–1074 CrossRef CAS.
- B. Matthaus and M. M. Özcan, J. Food Lipids, 2009, 16, 187–199 CrossRef.
- B. M. Iqdiam, H. Mostafa, R. Goodrich-Schneider, G. L. Baker, B. Welt and M. R. Marshall, Food Bioprocess Technol., 2018, 11, 634–644 CrossRef CAS.
- F. A. l. Juhaimi, M. Musa Ozcan, K. Ghafoor and E. E. Babiker, Food Chem., 2018, 243, 414–419 CrossRef PubMed.
- Q.-A. Zhang, X. Z. Fu and J. F. García Martín, Ultrason. Sonochem., 2017, 37, 405–413 CrossRef CAS PubMed.
- P. F. Qin, R. T. Liu, X. R. Pan, X. Y. Fang and Y. Mou, J. Agric. Food Chem., 2010, 58, 5561–5567 CrossRef CAS PubMed.
- D. J. Li, Y. Wang, J. J. Chen and B. M. Ji, Spectrochim. Acta, Part A, 2011, 79, 680–686 CrossRef CAS PubMed.
- J. Rosa-Millán, J. L. Orona-Padilla, V. M. Flores-Moreno and S. O. Serna-Saldívar, Int. J. Food Sci. Technol., 2018, 53, 1414–1424 CrossRef.
- A. B. Stefanović, J. R. Jovanović, M. B. Dojčinović, S. M. Lević, V. A. Nedović, B. M. Bugarski and Z. D. Knežević-Jugović, Food Bioprocess Technol., 2017, 10, 1224–1239 CrossRef.
- R. Jaenicke, Eur. J. Biochem., 1991, 202, 715–728 CrossRef CAS.
- S. N. Swain, K. K. Rao and P. L. Nayak, Polym. Int., 2005, 54, 739–743 CrossRef CAS.
- R. Ram, R. V. Chand, A. Forrest and P. C. Southgate, LWT--Food Sci. Technol., 2017, 86, 261–269 CrossRef CAS.
|
This journal is © The Royal Society of Chemistry 2020 |
Click here to see how this site uses Cookies. View our privacy policy here.